Recent advances in Ni-based electrocatalysts for low-energy hydrogen production via alternative pathways to water electrolysis
Abstract
Energy-efficient water electrolysis is one of the most promising techniques for generating green hydrogen as a carbon-free energy source. As a half-reaction of water splitting, the oxygen evolution reaction is kinetically sluggish, leading to large thermodynamic potential gaps compared to the hydrogen evolution reaction. In terms of cost-effective hydrogen generation, mitigating this overpotential is a challenging obstacle, but it remains a hurdle to overcome. It is necessary to advance energy-saving hydrogen production by substituting with an oxygen evolution reaction as a thermodynamically favorable anodic reaction. Additionally, depending on the specific small molecules used for the anodic oxidation reaction, it is possible to reduce environmentally harmful substances and produce value-added chemicals. Nickel-based electrocatalysts have received growing attention for their application in electrochemical reactions due to their affordability, versatility in structural tuning, and ability to function as active sites for bond formation and cleavage. The purpose of this paper is to probe how the morphology, structure, and composition of these catalysts affect the electrocatalyst performance for small molecule oxidation. Explaining these relationships can accelerate the development of sustainable hydrogen production techniques by identifying the design principles of high-performance nickel-based electrocatalysts.
Keywords
INTRODUCTION
It is widely acknowledged that the use of fossil fuels should be reduced due to environmental pollution and global warming problems[1]. Sustainable energy conversion and production technologies are being actively developed to reduce reliance on fossil fuels[2]. Hydrogen is one of the most efficient and promising alternatives to replace fossil fuels as a sustainable energy source[3].
Hydrogen has an energy density of 33.5 kWh per kg, about 2.6 times higher than diesel, with an energy density of 13 kWh per kg[4]. The high energy content accounts for its emergence as an alternative to fossil fuels[5]. Another point is that hydrogen fuel clearly reduces carbon dioxide emissions compared to petroleum-based fuels[6]. As interest in carbon neutrality increases worldwide, the demand for hydrogen is expected to expand further, thereby highlighting the need for efficient hydrogen production systems[7].
Hydrogen is obtained through various methods, including natural gas reforming, industrial byproduct hydrogen, and water electrolysis[8]. However, the hydrogen produced as a byproduct or reformed in industrial processes is not environmentally suitable, as it consumes much energy and incurs high operational costs, limiting the achievement of carbon neutrality[9]. Water electrolysis offers a substantial way to produce cost-efficient hydrogen reducing carbon dioxide emissions[10].
Most literature informs about the overpotential of oxygen evolution reaction (OER) in relation to the complex four-electron transfer process and mechanism[11]. Thermodynamically, the onset potential for OER is set at 1.23 V [vs. reversible hydrogen electrode (RHE)] with a current density of 10 mA cm-2. However, a higher potential is typically required to enhance the OER and subsequently increase hydrogen generation[12]. For this reason, it is essential to substitute the OER with a thermodynamically and kinetically favorable oxidation half-reaction to energy-saving hydrogen generation[13].
For example, urea, ammonia, and hydrazine are nitrogen-containing molecules that act as pollutants in the air or water. These molecules have the low theoretical onset potential of electrochemical oxidation[14]. The coupling with these anodic reactions with cathodic hydrogen evolution reaction (HER) enables the mitigation of environmental pollution while reducing the energy required to generate hydrogen[15]. Furthermore, other small-molecule oxidation reactions coupled with HER contribute to reducing the thermodynamic potential yield value-added products[16]. This approach offers the potential to produce hydrogen with lower energy requirements and an important strategy for improving its role as a sustainable energy source. As these technologies advance, more efficient and environmentally sustainable pathways for hydrogen production are expected to emerge.
Over the decades, research has focused on noble metal electrocatalysts such as platinum (Pt), Ru, and Ir. In 2009, the first Ni-based catalyst for urea electrolysis was reported, comparing its performance to Pt, Pt-Ir, and Rh[17]. For hydrazine oxidation, studies date back to 1981, with substantial efforts emerging in the 2010s[18]. In ammonia oxidation, Yao et al. explored Ni catalysts in alkaline solutions to assess activity[19]. Noble metals face challenges including high costs and limited industrial scalability, prompting interest in transition metals in the form of oxides, hydroxides, or chalcogenides[20]. Transition metals can also serve as active sites or supports in heterogeneous structures such as heterojunctions or 2D frameworks[21].
Ni stands out for its low cost, availability, and modifiable properties, making it suitable for large-scale applications[22]. Its surface can be engineered through heterostructures, doping, or alloying to enhance reaction kinetics and electrochemical surface area (ECSA)[23]. Doping modifies electronic structure of Ni, improving reaction dynamics. In alkaline conditions, Ni forms oxides and hydroxides, with oxidation state changes influencing activity and stability[24]. This paper reviews recent advancements in Ni-based catalysts, providing groundwork for future designs.
General design strategies of electrocatalyst for hydrogen production
Catalyst-support interaction
Carbon-based supports such as graphene, carbon nanotubes, and nitrogen-doped carbon offer high surface area, conductivity, and tunable properties for stabilizing catalysts[25]. They anchor metal nanoparticles (NPs), nanoclusters (NCs), or single-atom catalysts (SACs), improving dispersion, stability, and activity. Functional groups (e.g., carboxyl and hydroxyl) and chemical modifications(e.g., oxidation and sulfonation) anchor catalytic metals, prevent aggregation, and improve dispersion[26]. Nitrogen doping adjusts electronic structure, increases active sites, and stabilizes catalysts via strong metal-nitrogen bonds[27]. Sulfur or phosphorus doping boosts binding energy and charge transfer. Structural defects, such as single or double vacancies, create reactive sites that enhance metal binding and dispersion[28]. Hybrid materials (e.g., graphene-carbon nanotube composites) improve surface area and mass transport, while encapsulating NPs between carbon layers prevents leaching and aggregation for long-term efficiency[29,30]. Carbon supports improve electron transfer and reaction kinetics through strong catalyst-support interactions. For instance, Ru NCs on defective graphene enhance water-splitting efficiency by increasing electron conductivity[31]. SACs on nitrogen-doped carbon demonstrate optimized atomic coordination, stabilize intermediates, and improve kinetics, with annealing and impregnation techniques ensuring robust activity[32].
Metal-based supports such as reducible oxides (e.g., CeO2 and TiO2) stabilize NPs, NCs, and SACs through redox properties and tunable electronic interactions[33]. Strong metal-support interactions (SMSI) modulate adsorption energies(e.g., CO, NH, and OH species) and resist deactivation[34]. Oxygen or metal vacancies in oxides anchor SACs and prevent aggregation[35]. Dopants (e.g., nitrogen and sulfur) modify electronic density and improve charge transfer, influencing reactivity and selectivity[36]. Annealing under reducing or oxidizing conditions tunes the local coordination environment, enhancing activity and stability. For instance, Ir NCs on covalent organic polymers show improved stability and activity[37].
Surface engineering
Surface modification techniques, including the introduction of defects or heteroatom doping, enhance catalytic activity by increasing the number of available reactive sites and optimizing the adsorption of reactants[38]. Creating surface defects, such as nitrogen or oxygen vacancies, optimizes the adsorption and activation of target molecules such as Ammonia, Urea, Hydrazine and their intermediates[39]. The combination of crystalline and amorphous structures allows for efficient charge separation and transfer. The crystalline domains serve as pathways for electron transport, while the amorphous domains reduce recombination losses by trapping intermediates effectively[40].
Morphology engineering
Hierarchical structures, such as porous nanoarrays, increase the surface area and improve mass transfer. Ultrathin nanosheets or nanorods expose a greater fraction of reactive edges and corners, which are more catalytically active than flat surfaces[41]. By combining a stable core with a reactive shell, core-shell catalysts reduce degradation during reactions while maximizing active site availability[42]. Hollow or tubular designs minimize diffusion resistance, allowing reactants to access active sites more efficiently[43]. Layered structures expose interlayer spaces to reactants, facilitating catalytic reactions[44].
Synergistic effects through heterostructures
Different components in heterostructures provide complementary properties, such as enhanced charge separation or optimized adsorption energies for reactants. Heterostructures create localized electrophilic and nucleophilic domains, effectively adsorbing and activating target molecules[45]. The interaction between the materials at the interface creates new electronic states, enhancing the catalytic activity by lowering the energy barriers of the reaction[46]. The heterojunction improves the charge transfer between materials through the dynamic charge redistribution improving adsorption and reaction kinetics[47].
Adjustment of electronic structure and ensemble effects
Introducing multi-metallic components and dopants, such as Co, W, Mo, Fe, or heteroatoms including N, S, and P, can effectively optimize electronic structures, facilitate charge redistribution, and lower energy barriers. Modifying charge distributions using dopants or lattice engineering can stabilize these species, improving catalytic performance[44]. The formation of high-valent nickel species, such as Ni3+ or Ni4+, is crucial for active catalytic phases[48]. Co enhances the electron density at the Ni sites, while Mo contributes to improved hydrogen adsorption and desorption[49]. Adjusting the d-band center or density of states (DOS) optimizes the adsorption and desorption of reactants and intermediates. For example, in NiMoO3S, sulfur doping improved electron transfer efficiency and urea adsorption by shifting the DOS closer to the Fermi level thereby increasing carrier concentration and facilitating electron transport[50].
Electrochemical measurement and performance evaluation criteria
Electrochemical measurements for urea oxidation reaction (UOR), ammonia oxidation reaction (AOR), hydrazine oxidation reaction (HzOR), and small molecule oxidation (SMOR) typically involve cyclic voltammetry (CV), linear sweep voltammetry (LSV), chronoamperometry (CA), chronopotentiometry (CP), and electrochemical impedance spectroscopy (EIS)[51-54].
CV and LSV tests, conducted with or without the target substance, assess activity overpotentials and compare catalytic performance, often normalized to ECSA. Lower overpotentials at 10 mA/cm2 or
Oxidation reactions must occur at lower overpotentials than OER, within a favorable potential region preceding OER onset. By detecting the amount of product generated within this region, key metrics such as selectivity (%), yield (%), and faradaic efficiency (FE, %) can be calculated by[52]:
Where n, F and Q represent the number of electrons transferred, the Faraday constant (96,485 C/mol) and total electric charge (in coulombs) passed during the electrochemical formation of a specific product, respectively. Gas chromatography (GC) and differential electrochemical mass spectrometry (DEMS) are commonly utilized techniques for detecting gaseous products. Ion chromatography (IC) is generally used to analyze ionic species, and high-performance liquid chromatography (HPLC) is used for identifying
Energy efficiency (η) is a critical parameter in evaluating the performance of electrochemical systems. When the electrochemical conversion of the target substance is conducted on both electrodes of an electrolytic cell, η can be calculated as the ratio of the absolute value of the cell energy (Ecell) to the applied voltage (U), expressed as[52,58]:
Here, Ecell is determined by the FE and the respective electrode potentials as follows:
The energy contribution (E) of each reaction is further derived from the Gibbs free energy (∆G0) and is determined by:
Notably, the maximum combined FE of the system can reach up to 200%, as the contributions of the anodic and cathodic half-reactions are additive, with each capable of achieving 100%[52].
Ni-based electrocatalyst for urea oxidation reaction
Urea has been substantiated as a hydrogen carrier due to its distinctive advantages, which include nontoxicity, nonflammability, convenience in storage and transportation, and substantial hydrogen contents[51,59,60]. The thermodynamic equilibrium potential of hydrogen production using urea oxidation as an anodic reaction is only 0.37 V vs. RHE[61,62]. However, the UOR is a six-electron reaction [CO(NH2)2 + 6OH- → N2 + CO2 + 5H2O + 6e-] involving several complex processes such as continuous dehydrogenation, C-N bond cleavage, N-N coupling, CO2 formation, and CO2 desorption[63,64]. This complexity accentuates the need to explore Ni-based electrocatalysts as a potential solution to enhance η.
Among the catalysts utilized for the UOR, Ni-based catalysts are widely favored due to their low cost, high current density, and low overpotential in alkaline conditions[65,66]. In particular, Ni-based hydroxides are recognized as promising UOR electrocatalysts, as they form highly active oxyhydroxides in alkaline environments, enhancing their catalytic performance[67,68]. This demonstrates a strong correlation between the electrocatalytic performance of urea oxidation and the redox activity of active surfaces, bringing to light the need for further investigation into these mechanisms[56,69]. Understanding these pathways is indispensable for optimizing Ni-based electrocatalysts and improving their efficiency in the UOR.
The research to boost the efficiency of UOR focuses on maximizing catalyst activity through metal doping and novel synthesis methods and optimizing reaction pathways[51,70]. However, the mechanisms and reaction pathways of the UOR remain poorly understood. Possible mechanisms and reaction pathways have been revealed using in situ surface-enhanced Raman (SER) and Operando synchrotron-radiation
Indirect chemical oxidation mechanism:
Direct mechanism:
With several possible UOR pathways, this study will mainly investigate various designed compositions and structures of Ni-based electrocatalysts for UOR.
Nickel oxide and (oxy)hydroxide-based electrocatalyst for UOR (NiO, NiOOH, and NiOH)
The UOR mechanism in alkaline environments, as posited by Botte, is referred to as indirect chemical oxidation. Another mechanism, called direct chemical oxidation, was proposed by several researchers[73-76]. In the indirect mechanism, NiOOH is first electrochemically oxidized and then reduced back to Ni(OH)2, indicating that NiOOH acts as an oxidizing agent to oxidize urea[77-79]. Since the Ni in the catalyst regenerates to its initial state (Ni2+) after the oxidation reaction process, it exhibits sustained catalytic activity[80]. In the direct mechanism, urea undergoes electrochemical oxidation facilitated by NiOOH, thereby preserving the integrity of the active NiOOH species[56].
Ni-based (oxy)hydroxides such as NiO, Ni(OH)2, NiOOH, and Ni-based layered double hydroxides (LDH) have demonstrated good catalytic performance for the UOR. For instance, Liu et al. reported a study on growing Ni Manganese (Mn) LDH on carbon fiber cloth (CFC). The nanoarray structure provides numerous active sites, enhancing catalytic activity[81]. Due to the presence of Mn, the oxidation peak of Ni to Ni3+ occurs at a lower voltage compared to Ni(OH)2[81-83]. Additionally, U-NiMn LDH exhibits superior electrocatalytic performance compared to bulk NiMn LDH by exposing more catalytic active sites. U-NiMn LDH was fabricated using a hydrothermal method on CFC [Figure 1A]. Atomic force microscopy (AFM) confirmed that the catalyst forms a nanosheet structure with a thickness of 5.5 nm to 5.7 nm, highlighting the structure of U-NiMn LDH.
Figure 1. Examples of Ni-layered double hydroxide catalysts. (A) Scheme of synthesis of the Ultrathin NiMn-LDH/CFC; (B) Corresponding Tafel slopes; (C) UOR stability evaluates the U-NiMn-LDH/CFC; (D) Multi-current process acquired with the U-NiMn-LDH/CFC catalyst; (E) CV curves of U-NiMn-LDH/CFC and B-NiMn-LDH; (F) XRD patterns of U-NiMn-LDH/CFC before and after long-term stability test of UOR and OER; (G) Mn 2p in U-NiMn-LDH/CFC before and after long-term durability tests of UOR and OER[81]. This figure is quoted with permission from Liu et al. LDH: Layered double hydroxides; CFC: carbon fiber cloth; UOR: urea oxidation reaction; CV: cyclic voltammetry; XRD: X-ray diffraction; OER: oxygen evolution reaction.
The honeycomb shape enhances catalytic activity by providing a large surface area, numerous active sites, and high conductivity[84,85]. The transmission electron microscopy (TEM)-energy-dispersive X-ray spectroscopy (EDS) spectrum of NiMn-LDH/CFC revealed an atomic ratio of approximately 2.97:1 (Ni), consistent with the atomic ratios typically observed in LDHs. In this study, the U-NiMn-LDH/CFC catalyst demonstrated UOR activity at 1.351 V (vs. RHE) with a current density of 20 mA cm-2 in a 1.0 M KOH solution containing 0.5 M urea, showing superior performance compared to commercial RuO2 (1.443 V). Additionally, the oxidation potential of the UOR was 272 mV lower than that of the OER, confirming that the UOR is a more thermodynamically favorable reaction. The U-NiMn-LDH/CFC catalyst exhibited a Tafel slope of 38.9 mV dec-1 [Figure 1B], indicating fast UOR kinetics, and maintained a current density of 34 mA cm-2 with only a 2.8% decrease over 25 hours [Figure 1C], demonstrating excellent stability. Additionally, stable potential levels at varying current densities in a 1.0 M KOH and 0.5 M urea solution highlighted its effective mass transport capabilities [Figure 1D]. The CV curves of Ni(OH)2/CFC and
After long-term electrocatalysis for the UOR, characterization of U-NiMn-LDH/CFC using scanning electron microscopy (SEM), TEM, X-ray diffraction (XRD) analysis, Raman spectroscopy, and X-ray photoelectron spectroscopy (XPS) confirmed structural and compositional stability. XRD data showed the main peak remained stable, while SEM and TEM images confirmed the nanosheet structure was intact. XPS data identified Ni, Mn, and O with Ni 2p peaks shifting slightly, indicating higher oxidation states
The high activity of Ni-based electrocatalysts in UOR is primarily attributed to a combination of key factors. The presence of Mn3+ in the catalyts enhances the electronic structure, facilitating the conversion of Ni2+ to Ni3+, which improves the electrocatalytic activity for the UOR[90]. The generated Ni species during the oxidation reaction play a significant role in urea electrolysis. Based on these analyses, Ni-based LDH not only generates active NiOOH species during urea oxidation but also provides an active site without precious metals[91]. A similar example is the heterostructure studied by Yan et al., in which the Ni Cobalt(Co) LDH exhibits low onset potential and high activity, attributed to the abundant NiCo LDH-NiCo(OH)2 interface and the dual metal characteristics[92].
Ni-based electrocatalysts have researched various strategies to improve UOR performance, particularly by engineering Ni-based oxides, hydroxides, and LDH. These strategies include morphological design, heteroatom doping, and surface engineering. For instance, Miao et al. demonstrated that dual cation modification using Co and Mn significantly enhances the catalytic activity and stability of Ni hydroxide [Ni(OH)2] nanosheets[93].
Additionally, Periyasamy et al. demonstrated that spinel NiMn oxide, particularly Ni1.5Mn1.5O4, exhibits superior electrochemical activity and low charge transfer resistance compared to other compositions, confirming its potential as an effective UOR catalyst[94]. Furthermore, Periyasamy et al. demonstrated that surface structure engineering of two-dimensional Ni(OH)2 nanocrystals contributes to improved UOR performance, achieving optimal results by utilizing specific KOH concentrations during synthesis[94]. The advancements in Ni(OH)2-based catalysts offer promising solutions for efficient urea conversion and hydrogen production, contributing to the progress of sustainable energy technologies[95].
Nickel-based chalcogenides and phosphides electrocatalyst for UOR (NiS, NiSe, and NiP)
Ni-based catalysts are commonly used in the UOR, with NiOOH acting as a key species. NiOOH is either directly or indirectly involved in the conversion of urea into N2 or CO2. Therefore, the aim of using
Figure 2. (A) Synthesis of Ni2P nanoflakes; (B) XRD pattern; (C) SEM image; (D) TEM image (inset shows the corresponding HRTEM image and SAED pattern); and (E) STEM-EDS elemental mapping shows uniformity of elemental[100]. This figure is quoted with permission from Liu et al. XRD: X-ray diffraction; SEM: scanning electron microscopy; TEM: transmission electron microscopy; HRTEM: high-resolution TEM; EDS: energy-dispersive X-ray spectroscopy; SAED: selected area electron diffraction.
XPS analysis of Ni2P nanoflakes shows Ni 2p peaks indicating Ni2+ in Ni-O/Ni-PO43- bonds, with Ni-P peaks at lower binding energies, suggesting that Ni ions in Ni2P are more negatively charged and enhance electron transfer [Figure 3A and B]. The P 2p spectrum confirms Ni2P formation with P-O bonds, while O 1s reveals surface oxidation, supported by STEM-EDS mapping [Figure 3C and D].Compared to
Figure 3. XPS spectra, (A) Ni 2p XPS spectrum for Ni2P nanoflake; (B) Ni 2p XPS spectrum for β-Ni(OH)2; (C) p 2p XPS spectrum for Ni2P nanoflakes; (D) O 1s XPS spectrum for Ni2P nanoflakes; (E) RRDE polarization curves in 1 M KOH solution at 1,600 rpm with and without 0.5 M urea at 5 mV s-1 and the ring potential is set as 0.2 V (vs. RHE) to detect the as-generated O2; (F) Polarization curves of the two-electrode water splitting systems with Pt mesh||Ni2P electrodes in 1 M KOH solution with and without 0.5 M urea at 5 mV s-1 (G) UOR mechanism on Ni2P and β-Ni(OH)2 nanoflakes in alkaline media[100]. This figure is quoted with permission from Liu et al. XPS: X-ray photoelectron spectroscopy; RRDE: rotating ring-disk electrode; UOR: urea oxidation reaction; RHE: reversible hydrogen electrode.
The stability of Ni2P nanoflakes during UOR was confirmed by CP, showing minimal potential shifts. Rotating ring-disk electrode (RRDE) tests revealed superior UOR performance for Ni2P compared to OER. Ni2P promotes NiOOH formation via PO43-, enhancing catalytic activity. While β-Ni(OH)2 generates more NiOOH, Ni2P enables efficient recovery of Ni(OH)2, supporting a direct UOR pathway, as confirmed by ECSA analysis. This direct oxidation pathway, along with in-situ NiOOH generation, contributes to superior UOR performance of Ni2P compared to the indirect pathway in β-Ni(OH)2 [Figure 3E and F]. The mechanism of Ni2P during the UOR is illustrated in Figure 3G[100].
Recently, Ni-based catalysts coordinated with diverse anions have garnered attention due to their excellent electrical conductivity and adjustable electronic configurations. Among them, Ni3S2 has good durability and metallic conductivity[102]. Zeng et al. have developed various Ni sulfides, some of which exhibit enhanced catalytic activity due to improved electronic structure achieved by doping with P and Mo [Figure 4A and B]. This study addresses a strategy to accelerate NiOOH production through an in-situ method to phosphorylate the surface of Ni sulfide to improve the efficiency of urea electrolysis[103]. Surface phosphorylation facilitates proton transfer, optimizes electronic structure, and increases active sites by introducing phosphate groups that act as proton acceptors. These modifications accelerate NiOOH formation and improve the urea oxidation kinetics, thereby enhancing overall electrolysis performance[104][Figure 4C].
Figure 4. (A) Scheme of Ni-based sulfide; (B) XRD, SEM, TEM and HAADF-STEM image of Ni3S2@Ni3P and line scanning profile of
Ni-based electrocatalyst for ammonia oxidation reaction
Ammonia (NH3) exhibits a high hydrogen density, making it an excellent candidate for use as a hydrogen carrier, with a hydrogen content of approximately 17.6% by weight[105]. Its molecular structure allows efficient hydrogen storage, providing an effective method for storage and transportation. Furthermore, the absence of oxygen eliminates the danger of explosion, unlike in water electrolysis[106]. The AOR is an important process to generate hydrogen with Nitrogen at a theoretical voltage of 0.097 V (vs. RHE). This is significantly lower than the required voltage for water electrolysis, which is 1.23 V (vs. RHE)[107].
Based on the study with the Pt surface, two distinct mechanisms were postulated, depending on the timing of N-N coupling. The first mechanism involves N-N coupling occurring on hydrogenated NHx absorbed species (Gerischer and Mauerer, G-M). The second mechanism involves N adatoms coupling with each other, where the adatoms closely interact and form a bond (Oswin and Salomon, O-S). These two mechanisms play a crucial role in enhancing the efficiency of the AOR. The study results indicate that the mechanism proposed by Gerischer and Mauerer is kinetically preferred. Additionally, research demonstrates the activity of Ni-based catalysts using an ammonia volcano plot[108,109]. Ni-based catalysts are significant due to their ability to activate N-H bonds in adsorbed species such as NHx, which is vital for facilitating the initial adsorption of ammonia on the catalyst surface. This activation not only initiates the reaction but also enhances the stability of key reaction intermediates, preventing their decomposition during the bond formation process[110,111]. Consequently, this stabilization allows for more efficient N-N bond formation, promoting a smoother progression of the reaction. Furthermore, Ni-based catalysts optimize the thermodynamic pathways by lowering energy barriers, making the overall reaction occur more readily[108].
Nickel oxide and (oxy)hydroxide-based electrocatalyst for AOR (NiO, NiOOH and NiOH)
Ni-based catalysts, known for their excellent reactivity under alkaline conditions, are the focus of active research. Nickel oxide (NiO) significantly enhances the efficiency of ammonia electrolysis and is expected to contribute to sustainable energy production[108,112]. Although the lack of understanding of the reaction mechanisms of ammonia oxidation, Herron et al. suggest that Ni, due to its stability in alkaline electrolytes, is considered a potential substitute for Pt, one of the best electrocatalysts for the AOR[108]. Ni-based compounds convert to NiOOH during oxidation process, with Cu doping enhancing the AOR activity of Ni(1-x)CuxOOH [Figure 5A and B]. Doping with Cu2+ increases the electron density around O-O-Ni, promoting NH3 adsorption, though performance declines at high Cu doping levels[108,113]. Based on this, Fe3+ was co-doped to modify Ni(1-x)CuxOOH. Fe3+ polarizes the electron cloud, further increasing the electron density around O-O-Ni and lowering the ∆G0 for adsorption[108] [Figure 5C and D].
Figure 5. (A) Ni-Cu-Fe-OOH and the schematic illustration of the ammonia oxidation process; (B) The energy barriers of the different reaction steps of the N - N mechanism in Ni-Cu-Fe-OOH and Ni-Cu-OOH. The inset figure shows the energy barrier of the bifurcation step of the G-M mechanism and the N + N mechanism in the Ni-Cu-Fe-OOH system. Charge difference (C) in Ni-Cu-OOH system; and (D) Ni-Cu-Fe-OOH system (purple corresponds to charge accumulation, while yellow corresponds to charge depletion)[111]. This figure is quoted with permission from Zhu et al.
When both Cu and Fe are co-doped into NiOOH, the electron density around the O atoms bonded to the dopants changes, lowering the energy barrier and leading to activation that enhances AOR activity. The synthesized NiCuFe electrode achieved a 90% ammonia removal efficiency at 0.55 V after 12 h, one of the highest efficiencies reported to date [Figure 6A and B]. This study presents a valuable strategy for developing high-performance AOR catalysts. The results suggest a higher likelihood of the N-N coupling reaction occurring via the N-N coupling pathway, rather than the Gerischer and Mauerer mechanisms[108,114].
Figure 6. (A) Ammonia removal efficiency at different condition of pH and (B) different ammonia concentration; (C) XPS of XAS spectra of Ni L2,3-edge; (D) Schematic illustration of energy barrier during ammonia adsorption on the surface of Ni-Cu-Fe-OOH and Ni-Cu-OOH; (E) CV profile and (F) Chronoamperometry curves of the NiCuFe electrode and NiCu electrode in 0.5 M NaOH w/wo 55 × 10-3 NH4Cl; (G) UV-vis curves of the electrolyte tested for AOR after 12 h, 15 h, 18 h, and 24 h, respectively; (H) Ammonia removal efficiency and Faradaic efficiency of activated NiCuFe electrode under different anode potentials[111]. This figure is quoted with permission from Zhu et al. XPS: X-ray photoelectron spectroscopy; XAS: X-ray absorption spectroscopy; CV: cyclic voltammetry; AOR: ammonia oxidation reaction; UV: ultraviolet.
The synthesized catalysts exhibited XRD peaks similar to those of commercial NiCu bimetallic electrodes. The shift in the (111) and (200) peaks due to Fe doping indicates an expansion of the interlayer spacing, which can facilitate the accommodation of OH- ions and potentially increase AOR activity. XPS analysis confirmed the electronic states of the surface material. Ni exhibited a distinct 2p3/2 peak at 856.7 eV, with a strong satellite peak indicating the presence of Ni2+. This peak showed a shift of 0.7 eV compared to the synthesized NiCuFe/CP, suggesting that the surface Ni metal was converted to Ni2+. The Cu 2p3/2 peak, located at 934.7 eV, displayed a strong satellite peak characteristic of Cu2+. After activation, the Fe 2p3/2 peak appeared broad around 713.9 eV, associated with Fe3+. The O 1s spectrum showed a main peak at 531.3 eV, attributed to metal hydroxides. These results confirm that the electrode surface was fully oxidized[108]
CV demonstrated the catalytic activity for the AOR, showing varying current densities depending on the presence or absence of NH4Cl. The presence of Fe significantly enhanced AOR activity, as indicated by the CV data [Figure 6E]. To assess the stability of the a-NiCuFe electrode, CP was performed at 0.55 V. The results confirmed that the a-NiCuFe electrode exhibited the highest current density under ammonia conditions, with the declining trend indicating the oxidation of ammonia [Figure 6F]. After the AOR test, the nitrate concentration in the electrolyte was measured, with no nitrite ions detected. IC revealed a nitrate concentration of 0.528 ppm, suggesting that the NiCuFe electrode facilitates N-N coupling, demonstrating its effectiveness as the AOR catalyst [Figure 6G and H]. By co-doping Cu and Fe onto NiOOH, the energy barrier is lowered, enabling efficient electrooxidation of ammonia[111].
Ni-based electrocatalyst for the hydrazine oxidation reaction
Hydrazine has been extensively used in aerospace as a propellant and rocket fuel, as a foaming agent in the synthesis of polymeric foams, as well as a precursor to pharmaceuticals, agrochemicals, and in steam cycles of nuclear and conventional power plants as an oxygen scavenger[115,116]. On the other hand, hydrazine is an exceedingly hazardous and carcinogenic compound, presenting a substantial threat to human health[117]. HzOR plays a critical role in both the elimination of hydrazine from industrial wastewater and as an alternative to the OER, minimizing the overpotential at the anode and thereby promoting a more
In the alternating pathway, the adsorption configuration of *N2H2 is HNNH*, where the NH groups alternate. In the distal pathway, the adsorption configuration of N2H2* is *NNH2, with one nitrogen bonded to two protons.
Under acidic conditions, the theoretically calculated standard equilibrium potential is
Under alkaline conditions, the theoretically calculated standard equilibrium potential is
Both are more negative than the potentials at which hydrazine begins to oxidize in acidic or alkaline solutions. The dehydrogenation of hydrazine to molecular nitrogen, a transformation that is seemingly straightforward, is accompanied by substantial kinetic constraints[120].
Nickel oxide and (oxy)hydroxide-based electrocatalyst for HzOR(NiO, NiOH, and NiOOH)
Ni-based electrocatalysts with suitable d-band configurations and versatile structures have gained significant attention for hydrazine oxidation (HzOR)[121]. NiO demonstrates superior performance in HzOR due to active phase formation, oxygen defects, and multi-metallic oxide structures[115,122]. Sakamoto et al. developed NiO/Nb2O5/C composites using evaporation drying and thermal annealing. Extended X-ray absorption fine structure (EXAFS) analysis revealed oxygen vacancies in the composites (ratios 8:1 and 4:1), leading to high activity, durability, and selectivity. NiO, a non-stoichiometric p-type semiconductor, thermodynamically favors Ni vacancies, reducing Ni-O coordination. Metallic Ni facilitates N-N bond breaking, while oxygen defects stabilize the electrooxidation process[123].
Ni/Fe and Ni/C oxides also demonstrate strong catalytic activity for HzOR, influenced by Ni oxidation states and metal synergies[124]. Askari et al. synthesized a NiFe2O4-reduced graphene oxide (rGO) nanocatalyst using hydrothermal methods, achieving 98% stability after 5,000 cycles[125]. Yang et al. created a NiC2O4-Nb2O5 hybrid on Ni foam via a two-step low-temperature reaction with Ni3S2 as a sacrificial template. Electron transfer from Ni to Nb redistributed charge within the composite, improving the rate-determining step (RDS)[126]. Before and after the OER and HzOR test, the binding energy of Ni2+ within the Ni 2p3/2 orbital decreases from 857.0 eV to 855.9 eV, the latter corresponding to the binding energy of Ni(OH)2. Additionally, the observed minimal Tafel slope (84 mV/dec) suggests that the presence of electron-deficient Ni species at the SNiC2O4-Nb2O5 interface likely facilitates the adsorption of hydrazine molecules, thereby promoting highly favorable catalytic kinetics for the HzOR[127].
Ni hydroxide/oxyhydroxide (Ni(OH)2/NiOOH) is a principal redox system widely used as a positive electrode in various applications[128]. LDHs, resembling two-dimensional materials, exhibit superior catalytic performance due to their unique geometry and hierarchical nanostructure, which enhance conductivity and ion transport, significantly improving HzOR activity[129]. LDHs are also effective supports, offering tunable elemental compositions[130]. Babar et al. synthesized a bimetallic Ni-Fe hydroxide nanoarray [NiFe(OH)2-SD/NF] on Ni foam using stepwise electrodeposition. This method produced an amorphous NiFe(OH)2 structure with numerous active sites and random bond orientations. Synergistic interactions between Ni and Fe, particularly the presence of Fe ions alongside Ni(OH)2, greatly enhanced catalytic efficiency in alkaline HzOR. This enhancement is attributed to the facile intercalation of OH-, facilitated by the increased porosity, structural disorder, and the presence of edge sites or defects within the Ni(OH)2 matrix. Moreover, the amorphous structure of NiFe(OH)2 contains a greater number of unsaturated sites similar to those of metallic Ni, making it a highly effective catalyst for HzOR[131]. Liu et al. developed a ternary copper- Ni-Co LDH nanosheet array catalyst featuring sulfurization-induced and Cu-doped edge amorphization. The results indicated that the optimal concentration of the Cu dopant effectively modulated the electronic structure of the catalyst and enhanced the edge amorphization, which enriched the presence of high-valence Ni3+ ions. This effect is attributed to the formation of amorphous species during the sulfurization process, where Ni ions exhibit partial unsaturation, thus improving the performance in HzOR[48].
Nickel-based chalcogenides and phosphides electrocatalyst for HzOR(NiS, NiSe, and NiP)
Transition metal phosphide (MP) are highly effective electrocatalysts due to their superior electrical conductivity and rapid reaction kinetics[132]. Successive distortions in Ni phosphides arise from the interplay between metal-metal and phosphorus-phosphorus bonding. These compounds exhibit minor differences in binding energies compared to pure metals, retaining high electrical conductivity for efficient electron transport and reaction kinetics[133]. Their diverse structural phases and tunable electronic configurations contribute to significant synergistic effects[134].
A three-dimensional (3D) Ni-Co phosphide heterostructure, deposited on Ni foam (Ni-Co-P/NF), has been synthesized via a two-step hydrothermal method [Figure 7A]. Compared to CoP, the Co 2p spectra of
Figure 7. (A) HRTEM image of the Ni-Co-P/NF with a further magnified image in the inset; STEM-EDS elemental mapping of
A new reaction pathway for HzOR was proposed, involving N-N bond breakage in hydrazine at voltages above 0.2 V, suggesting a more complex mechanism than previously understood. At these voltages, Raman spectra revealed increased peak intensity for NH2, indicating the accumulation of the *NH2 intermediate from N2H4 bond cleavage. This new pathway (Path II) suggests that N2H4 adsorbs on the Ni-Co-P/NF surface, breaking into two *NH2 groups, which then dehydrogenate to produce N2[135,137]. The traditional proton-coupled electron transfer (PCET) process (Path I) remains the primary HzOR mechanism. However, Path II, involving N-N bond cleavage, becomes favorable at potentials above 0.2 V, where stronger binding of N atoms to the catalyst surface significantly reduces the N-N bond energy barrier (ΔG = 0.065 eV). This facilitates N-N bond weakening, enabling Path II to complement the PCET process
Liu et al. reported the synthesis of a heterojunction catalyst supported on Ni foam, consisting of amorphous NixP and crystalline Ni NPs, using a single-step electrodeposition technique. The catalytic activity of the HzOR in an alkaline electrolyte was compared with the performance of reference catalysts, a-NixP/NF and Ni/NF, under the same conditions. The a-NixP /Ni/NF catalyst exhibited superior performance with an onset potential of -0.11 V and a high current density of 1,215 mA cm-2 at +0.30 V, surpassing the reference catalysts. EIS and CV measurements showed the catalyst had the lowest charge transfer resistance and highest double-layer capacitance, indicating a larger ECSA. When normalized to ECSA, it demonstrated 1.3 and 1.5 times higher activity than a- NixP /NF and Ni/NF, respectively[40]. The superior performance of
Metal sulfides exhibit favorable electronic properties and defect profiles, with the assembly of Ni-based heterostructures demonstrating enhanced performance due to localized modulation of the electronic structure[139]. Zhou et al. synthesized CoNi-alloy@CoNi-sulfide core-shell nanoarrays in a three-step process: (1) CoNi-LDH nanosheet arrays were grown on a Ni foil via a hydrothermal method, (2) the arrays were reduced to form conductive CoNi-alloy (CoNi-R) particles, and (3) a CoNi-sulfide shell was formed through in situ vulcanization, producing CoNi-R-S [Figure 8A]. This distinctive structure exhibits a synergistic effect, where the conductive CoNi alloy core facilitates efficient electron transfer, while the CoNi-sulfide shell presents a high density of active sites that significantly enhance the electrooxidation of hydrazine[139-141]. TEM analysis revealed the nanosheet morphology of CoNi-R-S, where the CoNi-sulfide shell (7 nm thick) encapsulates the CoNi-alloy core (32 nm diameter). HRTEM analysis confirmed the coexistence of nanocrystalline CoNi-sulfide and CoNi-alloy. Elemental analysis verified the core-shell structure, with sulfur concentrated in the shell and Co/Ni in the core [Figure 8B]. Density functional theory (DFT) calculations showed that CoNi-sulfide has a smaller bandgap (0.75 eV) than CoNi-oxide (1.45 eV), suggesting better electron transport. The dissociation of hydrogen from N2H4 was more thermodynamically favorable on CoNi-sulfide, with lower activation energies for the dehydrogenation steps compared to
Figure 8. (A) Schematic illustration for the synthesis of CoNi-alloy@CoNi-sulfide nanoarrays (B) TEM image, crystal fringes and HAADF-STEM image and EDS mapping of CoNi-R-S; (C) The energy profiles for the reaction pathways over CoNi-sulfide and CoNi-oxide[141]. This figure is quoted with permission from Zhou et al. EDS: Energy-dispersive X-ray spectroscopy; TEM: transmission electron microscopy; HAADF-STEM: high-angle annular dark-field scanning transmission electron microscopy.
The CoNi-alloy core provides high conductivity for efficient electron transport, while the CoNi-sulfide shell offers abundant active sites for hydrazine electrooxidation. The hierarchical structure also facilitates mass diffusion and electrolyte penetration, contributing to the superior catalytic performance[141,142].
Metal nitrides exhibit exceptional catalytic efficiency across various reactions, particularly in the HzOR, due to their metallic properties, high electrical conductivity, and strong chemical stability and structural integrity[141]. The incorporation of nitrogen into Ni nitride alters the electronic properties of metals by increasing the electron density on its surface. This modification enhances electrocatalytic activity by improving the adsorption of intermediates[143]. Recently, molybdenum-doped Ni nitride (Mo-Ni3N) porous nanosheets grown on Ni foam (Mo-Ni3N/Ni/NF) were synthesized. The synergistic effects of interfacial engineering and chemical substitution in the molybdenum-doped Ni nitride demonstrated exceptional electrocatalytic properties for HzOR under alkaline conditions. The XPS survey confirmed Mo, Ni, and N in the Mo-Ni3N/Ni/NF sample, with no Mo in undoped Ni3N/Ni/NF, indicating successful Mo doping. The Ni 2p spectrum showed metallic Ni, Ni nitrides, and Ni2+ due to surface oxidation, with a 0.1 eV shift suggesting electron transfer between Mo and Ni. The N 1s peak at 397.2 eV indicated a metal-N bond, shifted by 0.5 eV, confirming Mo-Ni interaction. Mo 3d peaks revealed Mo3+, Mo4+, and Mo6+ states, and Ar+ etching confirmed Ni3N presence by leaving only metallic Ni and Ni3N peaks [Figure 9A]. Mo- Ni3N/Ni/NF exhibited superior catalytic activity for HzOR in alkaline media, outperforming Ni3N/Ni/NF. It achieved a working potential of -0.3 mV to reach a current density of 10 mA cm-2, and only 75 mV was needed for
Figure 9. (A) XPS spectra performed on the Mo/Ni3N/Ni and Ni3N/Ni powder scratched off the Ni foam. High-resolution XPS spectra of Mo/Ni3N/Ni for (A) Ni 2p, (B) N 1s, and (C) Mo 3d, respectively; High-resolution XPS spectra of Mo/Ni3N/Ni after Ar ion etching for Ni 2p, N 1s and Mo 3d, respectively; (B) The investigation on HzOR and HER activity for Mo/Ni3N/Ni/NF LSV curves and (C) Tafel plots of different catalysts toward HzOR (D) I-t test for Mo/Ni3N/Ni/NF toward HzOR (up) measured at the work potential of 124 mV and HER (down) at the work potential of 68 mV, both without iR correction; (E) The density of states and (F) Charge density difference and planar-averaged electron density difference of Mo-Ni3N/Ni; (G) Gibbs free energy profiles for the stepwise dehydrogenation process of N2H4 with solvent interactions on Mo-Ni3N/Ni, Ni3N/Ni, and Ni3N[144]. This figure is quoted with permission from Liu et al. XPS: X-ray photoelectron spectroscopy; HER: hydrogen evolution reaction; LSV: linear sweep voltammetry.
Similarly, incorporating copper can significantly modify the electronic configuration and improve the adsorption energy of intermediates, thereby accelerating catalytic kinetics. Porous nanosheets of copper-Ni nitride (Cu1Ni2-N), anchored onto CFC, were synthesized using CuNi-LDH as a precursor through a thermal ammonolysis process. Specifically, the Cu1Ni2-N electrode exhibits an overpotential of 71.4 mV at a current density of 10 mA cm-2 in 1 M KOH solution, while concurrently achieving an extraordinarily low potential of 0.5 mV at 10 mA cm-2 for the HzOR in a 1.0 M KOH/0.5 M hydrazine electrolyte. As confirmed by both experimental results and DFT calculations, Cu1Ni2-N exhibits excellent electrical conductivity, facilitating rapid electron transport during electrocatalytic processes. The DOS analysis shows that the
A strong local electric field is established between electron-rich Ni3N and electron-deficient Co3N by systematically engineering bimetallic nitrides. The electric field is leveraged to further activate electron transfer by introducing Mn, leading to improved system performance. The closely aligned crystalline structures of Ni3N and Co3N establish an intrinsic conductive pathway, facilitating efficient electron transfer. From the DFT calculation, introducing Mn reduces the work function (Wf) difference between
Heterostructured Ni-based electrocatalysts for HzOR
Electrochemical reactions occurring through heterogeneous catalysis are highly surface-sensitive, with their efficiency being strongly influenced by the surface chemical states and properties of the catalyst[147]. Consequently, heterostructure catalysts have been developed to optimize these interactions, utilizing key mechanisms such as the synergistic effect, electronic effect, surface defect effect, and multi-component synergistic effect[148,149].
Meanwhile, SACs, defined by atomically dispersed active sites and optimized utilization of metallic atoms enhance electrochemical activity[150,151]. The presence of unsaturated coordination optimizes the electronic DOS, leading to an increase in adsorption energy while simultaneously reducing the activation energy required for reactions[152]. As a specific example in the context of HzOR, Zhou et al. synthesized a Ti3C2Tx-MXene, with rich titanium vacancies, which served as a substrate for the immobilization of discrete Ni single atoms (SAs) via a “self-reduction” strategy [Figure 10A]. DFT computations were conducted to elucidate the origin of the elevated HzOR activity. Incorporating Ni SAs into the MXene matrix shifts the
Figure 10. (A) Synthesis pathways for the Pd/NiCo2O4 composite[156]. This figure is quoted with permission from Senthil et al; (B) Projected electronic density of states of d orbitals and total electronic density states plots for Ni atom in Ti3C2Tx, Ti3C2Tx, and
Due to their inherently superior catalytic properties, noble metals such as palladium (Pd), Pt, Rh, and Ru have been incorporated into Ni-based catalysts to enhance the catalytic efficiency of the HzOR[155]. For example, Pd atomically dispersed on bimetallic NiCo2O4 nanoplates enhances and accelerates the kinetics of hydrazine dehydrogenation. Additionally, Pd deposition occurs as NPs, NCs, or SAs on NiFe-LDHs, adjusting the d-band center and promoting stronger hybridization between Pd d-orbitals and the σ orbitals of N2H4 molecules. Recently, the synthesis of single-Pd-NPs-functionalized NiCo2O4 (Pd/NiCo2O4 composite) was achieved through a synergistic hydrothermal and calcination process, using 2D NiCo-LDH as the precursor. Then pulsed laser irradiation (PLI) methodology was employed to functionalize Pd NPs with diverse concentrations on the NiCo2O4 surface [Figure 10A]. The Pd/NiCo2O4-2 composite demonstrated superior electrocatalytic activity for the HzOR in an alkaline medium. Notably, it recorded a very low operating potential of -6 mV (vs. RHE) at a current density of 10 mA cm-2, indicating excellent performance[156]. According to In Situ/Operando Raman spectroscopy, new peaks at 462 cm-1 and 530 cm-1 related to γ-NiOOH and at 636 cm-1 and 814 cm-1 corresponding to NH2 signals appearing during HzOR
Liu et al. investigated the size effect of Pd spcies at the subnanometer scale in HzOR, ranging from NPs and NCs to SAs on a NiFe-LDH, by fine-tuning precursors and reduction methods [Figure 11A][160]. Pd NCs on NiFe-LDH were found to be the most effective catalysts for hydrazine oxidation, outperforming SAs and NPs different from previous studies. Despite lower metal loading in Pd SAs/NiFe, its mass activity remained low, demonstrating that HzOR activity is more influenced by the size of Pd species than by the metal content. The order of activity is Pd NCs/NiFe > > Pd NPs/NiFe > PdSAs/NiFe. In order to elucidate the catalytic properties of the three Pd catalysts, in situ EIS was conducted to examine the dynamic characteristics during the HzOR. The low-frequency region in the Bode plots reflects hydrazine mass transfer. Both Pd NCs/NiFe and Pd NPs/NiFe exhibited a transition peak at 0.15 V (vs. RHE), indicating electrooxidation of hydrazine [Figure 11B]. However, Pd SAs/NiFe showed no transition peak, indicating poor HzOR activity[160]. The DFT calculation indicated that Pd NCs/NiFe demonstrated the best catalytic performance due to optimal electronic structure and minimal steric hindrance, allowing easier dehydrogenation steps compared to Pd SAs and Pd NPs[159,160]. Both electronic structure and steric effects significantly influence HzOR activity [Figure 11C and D][160].
Figure 11. (A) Illustration of the Preparation of Pd SAs/NiFe, Pd NCs/NiFe, and Pd NPs/NiFe (B) Bode plots for the three Pd catalysts; (C) Reaction pathways of three Pd catalysts for the HzOR; (D) Intermediates of the HzOR for Pd NCs/NiFe[160]. This figure is quoted with permission from Liu et al. SAs: Single atoms; NPs: nanoparticles; NCs: nanoclusters.
Recently, partially substituting Ni with Rh within Ni-BDC [i.e., a metal-organic framework (MOF) built from Ni nodes and 1,4-benzenedicarboxylate (BDC) ligands) was synthesized. Rh atoms function as the catalytic sites facilitating hydrogen evolution, whereas Ni is accountable for the oxidation of hydrazine during the process of hydrazine oxidation-assisted seawater splitting. An exceptional level of stability was attained for the synthesized NiRh0.016-BDC, which can be ascribed to the contractive nature of the Ni-O bond subsequent to the incorporation of Rh, thereby demonstrating that appropriate node engineering could markedly augment the long-term stability of MOF catalysts. The DFT computations indicated that the Rh nodes within NiRh-BDC can accept electrons from the neighboring Ni, thereby functioning as active sites that exhibit enhanced adsorption of H2O* and H* during the HER, while the modified Ni site could facilitate a reduction in the energy barrier associated with N2H4 dehydrogenation as a consequence of charge transfer with proximate atomic Rh during the HzOR[161].
In the case of introduced Pt, the catalytic properties of the PtNi catalyst may arise from its interaction with hydrazine surface adsorbates, which act as intermediaries for both non-faradaic dehydrogenation and faradaic electrooxidation processes. The PtNi nanocatalyst exhibits high catalytic efficiency for the complete dehydrogenation of N2H4. Comparative investigations reveal that the catalytic efficiency of the Pt0.2Ni0.8 catalyst is enhanced due to its reduced particle dimensions and demonstrates superiority over alternative nanocatalysts, such as Pt, Ni, Pd0.2Ni0.8, and Au0.2Ni0.8[161].
Ru has been investigated to enhance the efficiency of the HzOR by accelerating dehydrogenation kinetics, and Ru is proposed as a potential candidate for the HER due to its hydrogen adsorption energy being comparable to that of Pt. In this regard, atomic Ni(Co)-Ru-P interfacial sites with Ru SAs (Ru1-NiCoP) were constructed on a matrix of NiCoP nanowire arrays (NWAs). According to the EXAFS data and DFT calculation, the geometric and electronic structure analyses suggest that the d-electron distribution and energy levels in NiCoP are effectively modulated by Ru-SA, leading to improved electrocatalytic performance for HzOR[162].
Bimetallic alloys often exhibit superior catalytic performance compared to their monometallic counterparts[163]. Alloying allows for tuning of adsorption/desorption energies of reaction intermediates, often bringing them closer to optimal values for catalysis[164]. The presence of a second metal can alter the electronic structure of the catalyst surface, potentially lowering activation barriers[165]. From this perspective, NiCo/MXene was synthesized through the deposition of NiCo-MOF nanosheets onto MXene-coated copper foam, subsequently undergoing annealing in an ammonia atmosphere [Figure 12A and B]. DFT calculations explored the origin of the HzOR activity on Ni3Co alloy. Among the facets studied (100, 110, 111), the (100) facet showed the strongest N2H4 adsorption with the most negative binding energy (-1.54 eV to -1.89 eV), indicating the highest activity for activating N2H4. Charge transfer from N2H4 to Ni and Co atoms weakens N-H bonds, facilitating HzOR. The dehydrogenation steps are more favorable on the (100) facet, with the RDS being the conversion of N2H3 to N2H2*, while the (110) and (111) facets face higher energy barriers during the initial dehydrogenation [Figure 12C][166].
Figure 12. (A) Schematic illustration of the synthetic strategy of NiCo@C/MXene/CF; (B) Free energy profiles of stepwise HzOR on different facets of Ni3Co alloy. Inset is the corresponding structural evolution of reaction intermediates adsorbed on the (100) facet of Ni3Co; (C) Elemental mapping showing the uniform distribution of C, N, Ti, Ni, and Co elements in this electrode. Scale bar, 5 μm[166]. This figure is quoted with permission from Sun et al.
Feng et al. synthesized single crystalline ultrathin NixCo1-x alloy nanosheet arrays (ANSAs) exhibiting finely tunable compositions (NixCo1-x, where x ranges from 0.9 to 0.5) through the gentle topochemical reduction of NixCo1-x(OH)2 nanosheet array precursors under mild conditions. The remarkable electrocatalytic efficiency of ultrathin Ni0.6Co0.4-ANSA can be attributed to its exceptionally high intrinsic activity towards hydrazine oxidation, as well as its significantly increased surface area and the abundance of unsaturated atoms (i.e., surface atoms, step/corner atoms) resulting from the ultrathin two-dimensional nanostructures. These unsaturated atoms may serve as highly efficient sites for electrocatalytic activity[167]. Similarly, Sun et al. developed a 3D hierarchically nanotubular Ni-Cu alloy on Ni foam [Ni(Cu)/NF] for HzOR catalyst. The optimal HzOR performance of Ni(Cu)/NF is achieved with 2% Cu doping after 400 s dealloying, attributed to a large ECSA and a 3D hierarchical porous structure. This structure enhances electron transfer, mass transport, and gas release, despite the activity decreasing with further Cu dissolution[168]. Zhang et al. synthesized a 3D Ni-Fe alloy catalyst supported on NF using hydrothermal method and reductive calcination. The Ni-Fe/NF catalyst exhibits excellent performance in hydrazine oxidation, offering high activity, stability, and selectivity. Specifically, the influence of Fe on enhancing the HzOR activity while simultaneously suppressing the non-faradaic decomposition of hydrazine[169].
CARBON CONTAINED SMALL MOLECULE OXIDATION REACTION
By incorporating chemical compounds with lower oxidation potentials into the water electrolysis process, it becomes possible to couple thermodynamically favorable oxidation reactions with the HER, allowing the electrolytic system to achieve significant benefits at both the anode and cathode while simultaneously reducing energy consumption[129]. Additionally, certain carbon-containing SMOR can lead to the production of high-value chemicals[129]. For example, 5-Hydroxymethylfurfural (HMF) can be oxidized to the valuable product 2,5-furandicarboxylic acid (FDCA). The theoretical overpotential for the electrooxidation of HMF to FDCA can be derived from the thermodynamic considerations of the reaction. The complete oxidation of HMF to FDCA involves a six-electron transfer process[170]. This means that theoretically, six electrons are required to convert one molecule of HMF to one molecule of FDCA.
For the overall reaction, the calculated standard reaction potential is only 0.113 V (vs. normal hydrogen electrode, NHE), which is much smaller than the OER[171]. The oxidation of alcohols represents a highly promising and viable candidate for engaging in substitutional reactions specifically associated with the process of OER, which is a critical aspect of various electrochemical systems and energy conversion technologies[133]. The thermodynamic potential, a crucial parameter in evaluating the energetic feasibility of chemical reactions, particularly for the oxidation of methanol (CH3OH), is measured to be 0.103 V (vs. RHE)
During the methanol oxidation reaction (MOR), there is potential for the generation of a valuable product, formic acid (FA), which can be synthesized through this chemical transformation[172]. Furthermore, the glycerol oxidation reaction (GOR) exhibits a minimal theoretical oxidation potential [0.003 V (vs. RHE)], thereby rendering it a compelling alternative to the OER for the generation of hydrogen.
Ni-based electrocatalysts capable of efficiently oxidizing carbon-containing small molecules, including the substances mentioned above, were investigated as suitable alternatives for the OER[173,174].
Nickel oxide and (oxy)hydroxide-based electrocatalyst for SMOR (NiO, NiOH, and NiOOH)
Most carbon-based molecules, such as various forms of alcohols and aldehydes, typically undergo nucleophilic oxidation reaction (NOR), a key mechanism in organic chemistry that involves electron transfer from a nucleophile to an electrophilic species[175,176]. Recently, the β-Co0.1Ni0.9(OH)2/NF was synthesized for the oxidation of ethanol, phenylcarbinol, HMF, and benzylamine in NOR, producing acetic acid, benzoic acid, FDCA, and benzonitrile, respectively, with a lower theoretical potential than the OER. For β-Ni(OH)2 and NiO catalysts, the activity of NOR arises from β-Ni(OH)O (from lattice oxygen) and NiO(OH)ads (from adsorbed oxygen), respectively. The β-Ni(OH)2 operates via a unique two-step,
Figure 13. (A) LSV curves for b-Ni(OH)2/NF and b-Co0.1Ni0.9(OH)2/NF in 1 M KOH with 50 mM phenylcarbinol, HMF, benzylamine and ethanol. Inset shows the conversion, selectivity, and faradic efficiency of EOR for b-Co0.1Ni0.9(OH)2/NF; (B) Proposed NOR mechanism scheme for b-Ni(OH)2[176]. This figure is quoted with permission from Chen et al.; (C) Schematic presentation of the electron-injection mechanism for Ni-NiOOH/Ni(OH)2-nanowire electrode and Ni-NiO-NiOOH/Ni(OH)2-nanowire electrode; (D) Schematic diagram of the activated process[178]. This figure is quoted with permission from Trafela et al.; (E) Adsorption energies of OH-, glycerol, and the
Huang et al. synthesized a Pd/Ni(OH)2/rGO hybrid electrocatalyst in which Ni(OH)2 significantly enhanced the performance of Pd for the EOR in an alkaline solution by improving both activity and durability. The catalyst maintained a high mass activity of 440 mA mg-1 after prolonged use, attributed to the role of Ni(OH)2 in facilitating the removal of carbonaceous poisons. Additionally, Ni(OH)2 reduced the selectivity for C2 products, thereby enhancing reaction control[177].
In the case of aldehydes, Trafela et al. investigated a mechanism for transforming Ni nanowire (Ni-NW) electrodes, originally composed of metallic Ni coated with NiO, into a catalytically active, disordered
In the regards of GOR, NiCrO with vacancies (NiCrO-VCr,O) was synthesized by hydrothermal and annealing process and subsequently employed CV forming oxygen vacancies. The optimized NiCrO-VCr,O catalyst achieved impressive glycerol electrooxidation performance. In situ analyses (EIS, Raman spectroscopy, and attenuated total reflection (ATR) FTIR spectroscopy revealed distinct interfacial behaviors, unlike NiSx/Ni and NiOx/Ni nanorod arrays (NRAs), which undergo deep reconstruction to NiOOH for both OER and GOR; NiSex/Ni NRAs experience only shallow reconstruction under GOR, allowing direct glycerol oxidation at the interface [Figure 13C]. DFT calculations confirmed the enhanced adsorption of OH- and glycerol on NiCrO-VCr,O, indicating favorable negative adsorption energies
A NiOOH/Ni3S2/NF catalyst was synthesized by electrodepositing NiOOH on Ni3S2 nanosheets, enhancing electron transport and glycerol adsorption. The catalyst achieved a high FE (97.7%) for FA at 1.4 V (vs. RHE). The TEM analysis conducted subsequent to the GOR, in conjunction with the in situ Raman spectroscopy investigations, provided compelling evidence which indicated that the NiOOH species served as the genuine active site throughout the GOR process. In situ IR and 1H NMR studies identified the GOR pathway, while Raman spectroscopy revealed NiOOH formation at active sites [Figure 14A-C][179].
Figure 14. (A) The XPS high-resolution S 2p spectra and The Raman spectra of NiOOH/ Ni3S2/NF catalysts before and after the reaction; (B) The in situ IR spectra and the in situ Raman spectra of NiOOH/Ni3S2/NF catalysts in 1 M KOH with 0.1 M glycerol; (C) Proposed GOR pathway in alkaline media based on the NiOOH/Ni3S2/NF electrode[179]. This figure is quoted with permission from Xu et al. XPS: X-ray photoelectron spectroscopy; GOR: glycerol oxidation reaction.
Nickel-based chalcogenides and phosphides electrocatalyst for SMOR(NiS, NiSe, and NiP)
Recently, the Ni-based catalyst that engages in coordination with diverse anions on Ni NRAs (NiOx/Ni,
Figure 15. (A) In situ Raman spectra of NiSex/Ni NRAs at a constant potential of 1.35 V (vs. RHE) for 60 min during GOR and in situ
NiS exhibits superior electrocatalytic activity for the MOR and EOR in alkaline media, due to an increased ECSA and sulfate ions on the NiOOH surface. NiS achieves FEs over 95% for formate from MOR and over 80% for acetate from EOR, with nearly double the product yield compared to Ni-only electrodes in 10,000 s tests [Figure 15A]. DFT calculations indicate that sulfate groups on the NiOOH surface enhance electroconductivity and electron transfer, favoring ethanol oxidation with a lower total reaction energy
Figure 16. (A) Stable adsorption configurations of intermediates involved in the process of MOR on the NiOOH surface from the side and top view; (B) CV curves without and with 1 M methanol or ethanol at a scan rate of 50 mV s-1. CV curves as a function of methanol and ethanol concentration from 0.1 M to 1 M; (C) Comparison of Faradaic efficiency between the NiS and Ni electrodes[181]. This figure is quoted with permission from Li et al. CV: Cyclic voltammetry; MOR: methanol oxidation reaction.
Jiang et al. developed the Pd-Ni-P electrocatalyst, containing Ni and P dopants, exhibits enhanced EOR activity in alkaline media due to charge transfer effects and increased active sites. Compared to Pd-Ni and Pd-blk, Pd-Ni-P has a more amorphous structure, smaller particle size, lower onset potential, and reduced overpotential by 110 mV[182].
Heterostructured nickel based electrocatalyst for SMOR
Recently, Araujo et al. synthesized bimetallic electrocatalysts composed of Pd and Ni, followed by a comprehensive comparison of these catalysts to evaluate their kinetics in the MOR. Pd, Pd3Ni, and PdNi catalysts exhibit different MOR behaviors due to their unique interactions with intermediates and varying CO poisoning tolerance. Pd shows lower CO tolerance, favoring early intermediates such as formaldehyde (CH2O) over CO2. Pd3Ni more effectively avoids CO formation by following a pathway through CH2OOH, resulting in higher CO2 production and enhanced MOR efficiency. PdNi demonstrates the highest MOR activity by favoring highly oxidized intermediates (e.g., CHOO), which prevent CO formation and rely on OH- concentration to promote direct oxidation pathways to CO2. DFT calculations and experimental data confirm that greater OH- adsorption of PdNi supports its superior MOR performance compared to Pd and Pd3Ni[183].
Three bimetallic catalysts composed of Pt and Ni, designated as PtNi1 through PtNi3, were synthesized with differing Ni concentrations. Spectroscopic analyses revealed that surface Ni(OH)x islands form under alkaline conditions but block *OH adsorption, rendering the bifunctional mechanism inactive. Instead, Ni modulates the electronic structure of Pt, enhancing catalytic performance and promoting selective partial carbon-carbon (C-C) bond cleavage toward glycolate formation. PtNi2 demonstrates the highest glycerol oxidation activity due to an optimized Pt-Ni coordination that weakens *CO and *OH binding on Pt
Figure 17. (A) Products formed at different potentials with the Pt/C catalyst detected by online sampling coupled with HPLC. Each potential was held for 10 min, and 200 μL of electrolyte containing glycerol and oxidation products were withdrawn at the end of chronoamperometry. Formate should also be produced during the reaction. However, its HPLC signal is embedded in the glycerol peak and thus cannot be quantified; (B) Illustration of the Electronic Effect in PtNi Electrocatalysts (Green: Ni, gray: Pt) Under Potential less than 1 V (vs. RHE)[184]. This figure is quoted with permission from Luo et al. HPLC: High-performance liquid chromatography; RHE: reversible hydrogen electrode.
Nitaya et al. synthesized Pd NPs supported on Ni SAs encapsulated in carbon nanotubes (NiSA). The primary rationale for the pronounced enhancement in the alcohol oxidation of Pd/NiSA can be attributed to the distinctive synergetic effect exerted by the Ni SACs that effectively draw electrons from the supported Pd NPs. The Pd NPs, which possess a positive charge resulting from the elevation of the energy level of the Pd 3d orbitals, significantly extract electrons during the charge transfer mechanism, thereby promoting the cleavage of the C-C bonds[185].
CONCLUSION AND OUTLOOK
In conclusion, advancements in Ni-based electrocatalysts address challenges in energy-efficient hydrogen production. By facilitating various oxidation reactions as substitutes for OER, these catalysts reduce energy consumption, offering a sustainable alternative to fossil fuels [Table 1].
Summary of electrocatalytic performance of different applications
Catalyst | Electrolyte | Potential | Tafel slope | Stability | Reference |
Ultra thin-Ni-Mn LDH | 0.5 M Urea/1.0 M KOH | Overpotential = 1.351 V | 38.9 mV/dec | 40 h | [81] |
Co and Mn co-doped Ni(OH)2 nanosheet | 0.33 M Urea/1.0 M KOH | Overpotential = 1.38 V | 35 mV/dec | 25 h | [94] |
Mo/Ni-P | 0.5 M Urea/1.0 M KOH | Overpotential = 1.43 V | 30.2 mV/dec | 12 h | [99] |
Ni2P nanoflakes | 0.5 M Urea/1.0 M KOH | Overpotential = 1.6 V | 30.2 mV/dec | 20 h | [100] |
Ni-Fe phosphide/CF | 0.33 M Urea/1.0 M KOH | Overpotential = 1.39 V | 30.2 mV/dec | 8 h | [101] |
Ni3S2@Ni3P | 0.33 M Urea/1.0 M KOH | Overpotential = 1.36 V | 19.13 mV/dec | 20 h | [104] |
P-MoO2@CoNiP | 0.33 M Urea/1.0 M KOH | Overpotential = 1.38 V | 47.6 mV/dec | 100 h | [126] |
NiCuFe electrode | 0.055 M NH4Cl/0.5 M NaOH | Overpotential = 0.55 V | 47.6 mV/dec | 12 h | [111] |
Cu1Ni2-N/CFC | 0.5 M Hydrazine/1.0 M KOH | Overpotential = 0.5 mV | 19.13 mV/dec | 75 h | [121] |
Ni2B/rGO | 0.5 M Hydrazine/1.0 M NaOH | Overpotential = 0.3 V | 19.13 mV/dec | 50 h | [122] |
SNiC2O4-Nb2O5/NF | 0.5 M Hydrazine/1.0 M KOH | Overpotential = 1.41 V | 81 mV/dec | 100 h | [127] |
NixP/Ni/NF | 0.5 M Hydrazine/1.0 M NaOH | Overpotential = 0.3 V | - | 100 h | [40] |
Mo-Ni3N/Ni/NF | 0.1 M Hydrazine/1.0 M KOH | Overpotential = 0.55 mV | - | 110 h | [144] |
CoNi-R-S | 20 mM Hydrazine /1.0 M KOH | - | 67 mV/dec | 6,000 s | [142] |
Ni SACs/Ti3C2Tx | 0.5 M Hydrazine/1.0 M KOH | Onsetpotential = -0.03 V | 62 mV/dec | 24,000 s | [153] |
PW-Co3N NWA/NF | 0.1 M Hydrazine/1.0 M KOH | Overpotential = 0.358 V | 14 mV/dec | 10 h | [146] |
NiCo@C/MXene/CF | 0.5 M Hydrazine/1.0 M KOH | Overpotential = 0.049 V | 54.2 mV/dec | 125 h | [166] |
NiCrO-VcrO nanosheets | 0.1M Glycerol/1 M KOH | Overpotential = 1.37 V | 13.93 mV/dec | 12 h | [175] |
β-Co0.1Ni0.9(OH)2 | 0.05 M EtOH/1 M KOH | Overpotential = 1.29 V | - | 20,000 s | [176] |
Disordered-β-NiOOH/β-Ni(OH)2 | 0.004 M HCHO/0.1 M NaOH | Overpotential = 0.4 V | 99 mV/dec | - | [178] |
NiOOH/Ni3S2/NF | 0.1 M Glycerol/1 M KOH | Overpotential = 0.073 V | - | 24 h | [179] |
NiSex/Ni NRAs | 0.1 M Glycerol/1 M KOH | Overpotential = 1.43 V | 114.73 mV/dec | 48 h | [180] |
NiS-based electrode | 1 M MeOH/1 M KOH 1 M EtOH/1 M KOH | Overpotential = 1.368 V Overpotential = 1.339 V | - - | 10,000 s | [181] |
Pd-Ni-P | 1 M EtOH/0.1 M KOH | Overpotential = 0.11 V | 92 mV/dec | 120 min | [182] |
PtNi2/Ni(OH)x | 1 M Glycerol/1 M KOH | Overpotential = 0.8 V | - | 2 h | [184] |
Optimizing catalytic design involves adjusting electronic structure, surface area, and morphology to enhance performance. Metal doping or alloying modifies active sites, lowering reaction barriers in oxidation reactions such as UOR, AOR, HzOR, and SMOR. Ni-based catalysts with LDH structures excel due to their structural tunability, akin to 2D materials. For HzOR, integrating transition metals such as Co or Mn with Ni improves efficiency by modulating electronic structure and enhancing hydrazine dehydrogenation kinetics [Figure 18].
Figure 18. Summary of outlook highlighting the advantages, challenges and potential solutions of Ni-based electrocatalyst for different applications. UOR: Urea oxidation reaction; AOR:ammonia oxidation reaction; HzOR: hydrazine oxidation reaction; SMOR: small molecule oxidation.
Limitations and potential solutions
Despite advancements, challenges remain. The complexity of multi-electron transfer in oxidation reactions requires further investigation to clarify mechanisms. Achieving long-term stability under harsh conditions, such as AOR and HzOR, is difficult due to catalyst degradation. While progress has been made in understanding this degradation, robust designs such as core-shell structures (e.g., conductive alloy cores with oxide or sulfide shells) show promise in enhancing stability and activity.
Future guidelines in anodic reaction development
To advance anodic reactions, challenges such as selective adsorption, byproduct formation, and competing reactions (e.g., OER) can be addressed by leveraging the versatility of nickel. For UOR and SMOR, designing multi-metal active sites tailored to specific intermediates enhances reaction kinetics. Transition metals (e.g., Ti, Fe, Cu) and noble metals (e.g., Rh, Ir, Ru) synergize with Ni, while high-entropy materials enable quaternary or quinary combinations to improve performance. Investigating Ni-metal combinations for synergetic effects remains a key focus.
For AOR and HzOR, suppressing NH3 formation and promoting N-N coupling requires strategies such as dimeric active site design and optimizing site distances. SA dimers, sub-nanosized alloys, and partial anion substitution (e.g., N, P, S) can facilitate coupling by modulating site distances. Future research should refine synthetic methods for dimeric site formation and controlled metal or anion substitution.
Industrial perspective of promising or commercial catalyst
Mass production of electrocatalysts demands precision manufacturing techniques capable of producing millions of membrane electrode assembly (MEA) annually. For commercial electrocatalysts, production must ensure high-quality, eco-friendly catalysts using scalable methods such as roll-to-roll processing and efficient recycling. Ni-based catalysts dominate due to cost-efficiency balance, but challenges such as degradation, reverse currents, and inefficiencies at high current densities persist. Advancing MEA-level electrocatalyst design is crucial to address real-world issues such as mass transport, thermal management, and three-phase boundary interactions.
Bridging the gap between academia and practical applications
Future research should explore new material combinations and synthesis techniques to enhance the activity, selectivity, and stability of Ni-based catalysts. Machine learning (ML) facilitates high-throughput catalyst screening, reaction mechanism analysis, and structure-property relationship identification. By integrating ML with DFT, predictive precision improves while reducing discovery time and resources. Advanced ML models such as convolutional neural network (CNN) and gradient boosting regressions predict adsorption energies, reaction pathways, and optimal catalyst configurations, accelerating design under industrial conditions. Combining the computational hydrogen electrode (CHE) model with ML further refines active site identification and configuration optimization, boosting stability and efficiency. ML-trained models for Ni-based catalysts minimize trial-and-error, optimizing performance for UOR, AOR, HzOR, and SMOR applications.
In situ spectroscopy, such as High-energy-resolution fluorescence-detected X-ray absorption spectroscopy (HERFD-XAS) and Ambient pressure (AP) XPS, bridges academia and industry by providing precise insights into the electronic and geometric changes of catalysts under operational conditions. HERFD-XAS identifies shifts in oxidation states and coordination environments, while AP-XPS monitors real-time structural transformations, such as Ni(OH)2 converting to active NiOOH species. These techniques offer actionable data on catalyst behavior, crucial for industrial-scale optimization.
In summary, despite challenges, advancements in Ni-based electrocatalysts are driving sustainable hydrogen production. Current research offers a foundation for innovations in catalyst design. Interdisciplinary efforts in materials science, electrochemistry, and modeling can accelerate the development of efficient, durable catalysts, supporting global energy sustainability.
DECLARATIONS
Authors’ contributions
Topic selection, literature search, manuscript writing: Kim, M.; Joung, S.
Manuscript discussion and data curation: Kim, M.; Lee, S.; Kwon, H.
Topic selection, manuscript review, editing and supervision: Lee, H.
Availability of data and materials
Not applicable.
Financial support and sponsorship
This work was supported by the National Research Foundation of Korea (NRF) grant (NRF-2022R1A2C2093415), the Korea Basic Science Institute (National Research Facilities and Equipment Center) grant funded by the Ministry of Education (2022R1A6C101A751), and the SungKyunKwan University and the BK21 FOUR (Graduate School Innovation) funded by the Ministry of Education (MOE, Korea) and NRF.
Conflicts of interest
All authors declared that there are no conflicts of interest.
Ethical approval and consent to participate
Not applicable.
Consent for publication
Not applicable.
Copyright
© The Author(s) 2025.
REFERENCES
1. Welsby, D.; Price, J.; Pye, S.; Ekins, P. Unextractable fossil fuels in a 1.5 °C world. Nature 2021, 597, 230-4.
2. Zhang, H.; Nai, J.; Yu, L.; Lou, X. W. Metal-organic-framework-based materials as platforms for renewable energy and environmental applications. Joule 2017, 1, 77-107.
3. Glenk, G.; Reichelstein, S. Economics of converting renewable power to hydrogen. Nat. Energy. 2019, 4, 216-22.
4. Kazemi, A.; Manteghi, F.; Tehrani, Z. Metal Electrocatalysts for hydrogen production in water splitting. ACS. Omega. 2024, 9, 7310-35.
5. Staffell, I.; Scamman, D.; Velazquez, A. A.; et al. The role of hydrogen and fuel cells in the global energy system. Energy. Environ. Sci. 2019, 12, 463-91.
6. Gunathilake, C.; Soliman, I.; Panthi, D.; et al. A comprehensive review on hydrogen production, storage, and applications. Chem. Soc. Rev. 2024, 53, 10900-69.
7. Pickering, B.; Lombardi, F.; Pfenninger, S. Diversity of options to eliminate fossil fuels and reach carbon neutrality across the entire European energy system. Joule 2022, 6, 1253-76.
8. Parkinson, B.; Balcombe, P.; Speirs, J. F.; Hawkes, A. D.; Hellgardt, K. Levelized cost of CO2 mitigation from hydrogen production routes. Energy. Environ. Sci. 2019, 12, 19-40.
9. Wu, W.; Zhai, H.; Holubnyak, E. Technological evolution of large-scale blue hydrogen production toward the U.S. hydrogen energy earthshot. Nat. Commun. 2024, 15, 5684.
10. Li, W.; Feaster, J. T.; Akhade, S. A.; et al. Comparative techno-economic and life cycle analysis of water oxidation and hydrogen oxidation at the anode in a CO2 electrolysis to ethylene system. ACS. Sustainable. Chem. Eng. 2021, 9, 14678-89.
11. Lu, F.; Zhang, Y.; Liu, S.; et al. Surface proton transfer promotes four-electron oxygen reduction on gold nanocrystal surfaces in alkaline solution. J. Am. Chem. Soc. 2017, 139, 7310-7.
12. Zegeye, T. A.; Chen, W.; Hsu, C.; Valinton, J. A. A.; Chen, C. Activation energy assessing potential-dependent activities and site reconstruction for oxygen evolution. ACS. Energy. Lett. 2022, 7, 2236-43.
13. Arshad, F.; Haq, T. U.; Hussain, I.; Sher, F. Recent advances in electrocatalysts toward alcohol-assisted, energy-saving hydrogen production. ACS. Appl. Energy. Mater. 2021, 4, 8685-701.
14. Guo, W.; Zhang, K.; Liang, Z.; Zou, R.; Xu, Q. Electrochemical nitrogen fixation and utilization: theories, advanced catalyst materials and system design. Chem. Soc. Rev. 2019, 48, 5658-716.
15. Wang, H.; Sun, M.; Ren, J.; Yuan, Z. Circumventing challenges: design of anodic electrocatalysts for hybrid water electrolysis systems. Adv. Energy. Mater. 2023, 13, 2203568.
16. Lu, Y.; Chen, M.; Wang, Y.; Yang, C.; Zou, Y.; Wang, S. Aqueous electrocatalytic small-molecule valorization trilogy. Chem 2024, 10, 1371-90.
17. Boggs, B. K.; King, R. L.; Botte, G. G. Urea electrolysis: direct hydrogen production from urine. Chem. Commun. 2009, 4859-61.
18. Fukumoto, Y.; Matsunaga, T.; Hayashi, T. Electrocatalytic activities of metal electrodes in anodic oxidation of hydrazine in alkaline solution. Electrochim. Acta. 1981, 26, 631-6.
19. Yao, K.; Cheng, Y. Investigation of the electrocatalytic activity of nickel for ammonia oxidation. Mater. Chem. Phys. 2008, 108, 247-50.
20. Lyu, F.; Wang, Q.; Choi, S. M.; Yin, Y. Noble-metal-free electrocatalysts for oxygen evolution. Small 2019, 15, 1804201.
21. Zhang, H.; Gu, H.; Shi, G.; et al. Two-dimensional covalent framework derived nonprecious transition metal single-atomic-site electrocatalyst toward high-efficiency oxygen reduction. Nano. Lett. 2023, 23, 3803-9.
22. Jiang, K.; Li, J.; Zheng, Z.; et al. Bimetallic phosphide NiCoP electrocatalyst synthesized by one-step electrodeposition for efficient hydrogen evolution in acidic and alkaline solution. ACS. Appl. Energy. Mater. 2024, 7, 7895-905.
23. Park, G. D.; Park, J.; Kim, J. K.; Kang, Y. C. Recent advances in heterostructured anode materials with multiple anions for advanced alkali-ion batteries. Adv. Energy. Mater. 2021, 11, 2003058.
24. Ahmad, M. U.; Suresh, G.; Bang, J. H. Rescrutinizing the iron effect on oxygen evolution reaction catalysts under industrially relevant working conditions: overlooked mass transfer limitation driven by the iron incorporation. ACS. Energy. Lett. 2024, 9, 4953-8.
25. Du, R.; Zhang, N.; Zhu, J.; et al. Nitrogen-doped carbon nanotube aerogels for high-performance ORR catalysts. Small 2015, 11, 3903-8.
26. Singh, B.; Gawande, M. B.; Kute, A. D.; et al. Single-atom (iron-based) catalysts: synthesis and applications. Chem. Rev. 2021, 121, 13620-97.
27. Masa, J.; Xia, W.; Muhler, M.; Schuhmann, W. On the role of metals in nitrogen-doped carbon electrocatalysts for oxygen reduction. Angew. Chem. Int. Ed. 2015, 54, 10102-20.
28. Gerber, I. C.; Serp, P. A theory/experience description of support effects in carbon-supported catalysts. Chem. Rev. 2020, 120, 1250-349.
29. Etesami, M.; Nguyen, M. T.; Yonezawa, T.; Tuantranont, A.; Somwangthanaroj, A.; Kheawhom, S. 3D carbon nanotubes-graphene hybrids for energy conversion and storage applications. Chem. Eng. J. 2022, 446, 137190.
30. Shah, S. S. A.; Najam, T.; Bashir, M. S.; et al. Identification of catalytic active sites for durable proton exchange membrane fuel cell: catalytic degradation and poisoning perspectives. Small 2022, 18, 2106279.
31. Barman, B. K.; Sarkar, B.; Ghosh, P.; Ghosh, M.; Mohan, R. G.; Nanda, K. K. In situ decoration of ultrafine Ru nanocrystals on n-doped graphene tube and their applications as oxygen reduction and hydrogen evolution catalyst. ACS. Appl. Energy. Mater. 2019, 2, 7330-9.
32. Choi, J.; Seo, S.; Kim, M.; Han, Y.; Shao, X.; Lee, H. Relationship between Structure and performance of atomic-scale electrocatalysts for water splitting. Small 2024, 20, 2304560.
33. Lang, R.; Du, X.; Huang, Y.; et al. Single-atom catalysts based on the metal-oxide interaction. Chem. Rev. 2020, 120, 11986-2043.
34. Chen, J.; Zhang, Y.; Zhang, Z.; et al. Metal-support interactions for heterogeneous catalysis: mechanisms, characterization techniques and applications. J. Mater. Chem. A. 2023, 11, 8540-72.
35. Li, R.; Luo, L.; Ma, X.; Wu, W.; Wang, M.; Zeng, J. Single atoms supported on metal oxides for energy catalysis. J. Mater. Chem. A. 2022, 10, 5717-42.
36. De, S.; Burange, A. S.; Luque, R. Conversion of biomass-derived feedstocks into value-added chemicals over single-atom catalysts. Green. Chem. 2022, 24, 2267-86.
37. Che, W.; Tao, T.; Baek, J. Strategies for boosting the activity of single-atom catalysts for future energy applications. J. Mater. Chem. A. 2022, 10, 10297-325.
38. He, T.; Puente-santiago, A. R.; Xia, S.; Ahsan, M. A.; Xu, G.; Luque, R. Experimental and theoretical advances on single atom and atomic cluster-decorated low-dimensional platforms towards superior electrocatalysts. Adv. Energy. Mater. 2022, 12, 2200493.
39. Zhao, X.; Levell, Z. H.; Yu, S.; Liu, Y. Atomistic understanding of two-dimensional electrocatalysts from first principles. Chem. Rev. 2022, 122, 10675-709.
40. Liu, J.; Wen, H.; Zhang, Z.; Wang, P. An amorphous/nanocrystalline NixP/Ni heterojunction for electrooxidation of hydrazine. J. Mater. Chem. A. 2023, 11, 14213-20.
41. Zhang, Y.; Lv, Q.; Chi, K.; et al. Hierarchical porous carbon heterojunction flake arrays derived from metal organic frameworks and ionic liquid for H2O2 electrochemical detection in cancer tissue. Nano. Res. 2021, 14, 1335-43.
42. Gawande, M. B.; Goswami, A.; Asefa, T.; et al. Core-shell nanoparticles: synthesis and applications in catalysis and electrocatalysis. Chem. Soc. Rev. 2015, 44, 7540-90.
43. Pandikassala, A.; Gangadharan, P. K.; Veedu, R. N.; Kurungot, S. Polydopamine-derived iron-doped hollow carbon nanorods as an efficient bifunctional electrocatalyst for simultaneous generation of hydrogen and electricity. Energy. Fuels. 2022, 36, 11245-60.
44. Ahsan, M. A.; He, T.; Noveron, J. C.; Reuter, K.; Puente-Santiago, A. R.; Luque, R. Low-dimensional heterostructures for advanced electrocatalysis: an experimental and computational perspective. Chem. Soc. Rev. 2022, 51, 812-28.
45. Wang, Z.; Fei, H.; Wu, Y. N. Unveiling advancements: trends and hotspots of metal-organic frameworks in photocatalytic CO2 reduction. ChemSusChem 2024, 17, e202400504.
46. Gong, Q.; Yang, D.; Yang, H.; et al. Cobalt ditelluride meets tellurium vacancy: an efficient catalyst as a multifunctional polysulfide mediator toward robust lithium-sulfur batteries. ACS. Nano. 2024, 18, 28382-93.
47. Moniz, S. J. A.; Shevlin, S. A.; Martin, D. J.; Guo, Z.; Tang, J. Visible-light driven heterojunction photocatalysts for water splitting - a critical review. Energy. Environ. Sci. 2015, 8, 731-59.
48. Liu, W.; Xie, J.; Guo, Y.; Lou, S.; Gao, L.; Tang, B. Sulfurization-induced edge amorphization in copper-nickel-cobalt layered double hydroxide nanosheets promoting hydrazine electro-oxidation. J. Mater. Chem. A. 2019, 7, 24437-44.
49. Huang, C.; Xia, Z.; Wang, J.; et al. Highly efficient and stable electrocatalyst for hydrogen evolution by molybdenum doped Ni-Co phosphide nanoneedles at high current density. Nano. Res. 2024, 17, 1066-74.
50. Ge, W.; Lin, L.; Wang, S.; et al. Electrocatalytic urea oxidation: advances in mechanistic insights, nanocatalyst design, and applications. J. Mater. Chem. A. 2023, 11, 15100-21.
51. Gao, X.; Zhang, S.; Wang, P.; Jaroniec, M.; Zheng, Y.; Qiao, S. Z. Urea catalytic oxidation for energy and environmental applications. Chem. Soc. Rev. 2024, 53, 1552-91.
52. Guo, L.; Zhang, X.; Gan, L.; et al. Advances in selective electrochemical oxidation of 5-hydroxymethylfurfural to produce high-value chemicals. Adv. Sci. 2023, 10, 2205540.
53. Alzaabi, A.; Almarzooqi, F.; Choi, D. Ammonia electro-catalysis for hydrogen production: Mechanisms, materials, and scalability. Int. J. Hydrogen. Energy. 2024, 94, 23-52.
54. Liu, H.; Liu, Y.; Li, M.; Liu, X.; Luo, J. Transition-metal-based electrocatalysts for hydrazine-assisted hydrogen production. Mater. Today. Adv. 2020, 7, 100083.
55. Zheng, X.; Yang, J.; Li, P.; et al. Dual-atom support boosts nickel-catalyzed urea electrooxidation. Angew. Chem. Int. Ed. 2023, 62, e202217449.
56. Rao, N. N.; Alex, C.; Mukherjee, M.; et al. Evidence for exclusive direct mechanism of urea electro-oxidation driven by in situ- generated resilient active species on a rare-earth nickelate. ACS. Catal. 2024, 14, 981-93.
57. Peng, X.; Zeng, L.; Wang, D.; et al. Electrochemical C-N coupling of CO2 and nitrogenous small molecules for the electrosynthesis of organonitrogen compounds. Chem. Soc. Rev. 2023, 52, 2193-237.
58. Wang, T.; Tao, L.; Zhu, X.; et al. Combined anodic and cathodic hydrogen production from aldehyde oxidation and hydrogen evolution reaction. Nat. Catal. 2022, 5, 66-73.
59. Rollinson, A. N.; Jones, J.; Dupont, V.; Twigg, M. V. Urea as a hydrogen carrier: a perspective on its potential for safe, sustainable and long-term energy supply. Energy. Environ. Sci. 2011, 4, 1216-24.
60. Rollinson, A. N.; Rickett, G. L.; Lea-langton, A.; Dupont, V.; Twigg, M. V. Hydrogen from urea-water and ammonia-water solutions. Appl. Catal. B. Environ. 2011, 106, 304-15.
61. Liu, D.; Liu, T.; Zhang, L.; et al. High-performance urea electrolysis towards less energy-intensive electrochemical hydrogen production using a bifunctional catalyst electrode. J. Mater. Chem. A. 2017, 5, 3208-13.
62. Ding, H.; Zhao, Z.; Zeng, H.; et al. Heterojunction-induced local charge redistribution boosting energy-saving hydrogen production via urea electrolysis. ACS. Mater. Lett. 2024, 6, 1029-41.
63. Gao, X.; Bai, X.; Wang, P.; et al. Boosting urea electrooxidation on oxyanion-engineered nickel sites via inhibited water oxidation. Nat. Commun. 2023, 14, 5842.
64. Ji, Z.; Song, Y.; Zhao, S.; Li, Y.; Liu, J.; Hu, W. Pathway manipulation via Ni, Co, and V ternary synergism to realize high efficiency for urea electrocatalytic oxidation. ACS. Catal. 2022, 12, 569-79.
65. Wang, Z.; Guo, P.; Liu, M.; et al. Rational design of metallic NiTex (x = 1 or 2) as bifunctional electrocatalysts for efficient urea conversion. ACS. Appl. Energy. Mater. 2019, 2, 3363-72.
66. Zhou, M.; Weng, Q.; Popov, Z. I.; et al. Construction of polarized carbon-nickel catalytic surfaces for potent, durable, and economic hydrogen evolution reactions. ACS. Nano. 2018, 12, 4148-55.
67. Fu, X.; Pu, B.; Pan, L.; et al. Composition regulation of Ni-BDC MOF architecture to enhance electrocatalytic urea oxidation in alkaline solution. Mater. Chem. Front. 2024, 8, 3272-9.
68. Feng, C.; Wang, F.; Liu, Z.; et al. A self-healing catalyst for electrocatalytic and photoelectrochemical oxygen evolution in highly alkaline conditions. Nat. Commun. 2021, 12, 5980.
69. Naduvil, K. M. S.; Alex, C.; Rao, N. N.; et al. Unfolding the significance of regenerative active species in nickel hydroxide-based systems for sustained urea electro-oxidation. Chem. Mater. 2024, 36, 5343-55.
70. Jin, L.; Ji, R.; Wan, H.; et al. Boosting the electrocatalytic urea oxidation performance by amorphous-crystalline Ni-TPA@NiSe heterostructures and mechanism discovery. ACS. Catal. 2023, 13, 837-47.
71. Zhu, D.; Zhang, H.; Miao, J.; et al. Strategies for designing more efficient electrocatalysts towards the urea oxidation reaction. J. Mater. Chem. A. 2022, 10, 3296-313.
72. Song, J.; Qian, Z.; Yang, J.; Lin, X.; Xu, Q.; Li, J. In situ/operando investigation for heterogeneous electro-catalysts: from model catalysts to state-of-the-art catalysts. ACS. Energy. Lett. 2024, 9, 4414-40.
73. Vedharathinam, V.; Botte, G. G. Direct evidence of the mechanism for the electro-oxidation of urea on Ni(OH)2 catalyst in alkaline medium. Electrochim. Acta. 2013, 108, 660-5.
74. Zhu, X.; Dou, X.; Dai, J.; et al. Metallic nickel hydroxide nanosheets give superior electrocatalytic oxidation of urea for fuel cells. Angew. Chem. Int. Ed. 2016, 55, 12465-9.
75. Zhao, J.; Zhang, Y.; Guo, H.; et al. Defect-rich Ni(OH)2/NiO regulated by WO3 as core-shell nanoarrays achieving energy-saving water-to-hydrogen conversion via urea electrolysis. Chem. Eng. J. 2022, 433, 134497.
76. Huang, C. J.; Xu, H. M.; Shuai, T. Y.; Zhan, Q. N.; Zhang, Z. J.; Li, G. R. Modulation strategies for the preparation of high-performance catalysts for urea oxidation reaction and their applications. Small 2023, 19, 2301130.
77. Schranck, A.; Marks, R.; Yates, E.; Doudrick, K. Effect of urine compounds on the electrochemical oxidation of urea using a nickel cobaltite catalyst: an electroanalytical and spectroscopic investigation. Environ. Sci. Technol. 2018, 52, 8638-48.
78. Modak, A.; Mohan, R.; Rajavelu, K.; Cahan, R.; Bendikov, T.; Schechter, A. Metal-organic polymer-derived interconnected Fe-Ni alloy by carbon nanotubes as an advanced design of urea oxidation catalysts. ACS. Appl. Mater. Interfaces. 2021, 13, 8461-73.
79. Vedharathinam, V.; Botte, G. G. Experimental investigation of potential oscillations during the electrocatalytic oxidation of urea on Ni catalyst in alkaline medium. J. Phys. Chem. C. 2014, 118, 21806-12.
80. Wang, G.; Ling, Y.; Lu, X.; et al. A mechanistic study into the catalytic effect of Ni(OH)2 on hematite for photoelectrochemical water oxidation. Nanoscale 2013, 5, 4129-33.
81. Liu, G.; Huang, C.; Yang, Z.; Su, J.; Zhang, W. Ultrathin NiMn-LDH nanosheet structured electrocatalyst for enhanced electrocatalytic urea oxidation. Appl. Catal. A. Gen. 2021, 614, 118049.
82. Tian, T.; Gao, H.; Zhou, X.; et al. Study of the active sites in porous nickel oxide nanosheets by manganese modulation for enhanced oxygen evolution catalysis. ACS. Energy. Lett. 2018, 3, 2150-8.
83. Rao, R. R.; Corby, S.; Bucci, A.; et al. Spectroelectrochemical analysis of the water oxidation mechanism on doped nickel oxides. J. Am. Chem. Soc. 2022, 144, 7622-33.
84. Chia, X.; Pumera, M. Characteristics and performance of two-dimensional materials for electrocatalysis. Nat. Catal. 2018, 1, 909-21.
85. Feng, D.; Lei, T.; Lukatskaya, M. R.; et al. Robust and conductive two-dimensional metal-organic frameworks with exceptionally high volumetric and areal capacitance. Nat. Energy. 2018, 3, 30-6.
86. Pu, Y.; Lawrence, M. J.; Celorrio, V.; et al. Nickel confined in 2D earth-abundant oxide layers for highly efficient and durable oxygen evolution catalysts. J. Mater. Chem. A. 2020, 8, 13340-50.
87. He, X.; Yin, F.; Li, Y.; et al. NiMnO3/NiMn2O4 oxides synthesized via the aid of pollen: ilmenite/spinel hybrid nanoparticles for highly efficient bifunctional oxygen electrocatalysis. ACS. Appl. Mater. Interfaces. 2016, 8, 26740-57.
88. Liu, Z.; Zhang, N.; Xiong, Y. In situ raman characterizations for enhanced understandings on electrocatalysis. J. Phys. Chem. C. 2024, 128, 13651-65.
89. Yang, X.; Zhang, H.; Xu, W.; Yu, B.; Liu, Y.; Wu, Z. A doping element improving the properties of catalysis: in situ Raman spectroscopy insights into Mn-doped NiMn layered double hydroxide for the urea oxidation reaction. Catal. Sci. Technol. 2022, 12, 4471-85.
90. Andaveh, R.; Sabour, R. A.; Seif, A.; et al. In situ assembly of a superaerophobic CoMn/CuNiP heterostructure as a trifunctional electrocatalyst for ampere-level current density urea-assisted hydrogen production. ACS. Appl. Mater. Interfaces. 2024, 16, 8717-32.
91. Chen, Z.; Wei, W.; Shon, H. K.; Ni, B. Designing bifunctional catalysts for urea electrolysis: progress and perspectives. Green. Chem. 2024, 26, 631-54.
92. Yan, X.; Hu, Q.; Wang, G.; et al. NiCo layered double hydroxide/hydroxide nanosheet heterostructures for highly efficient electro-oxidation of urea. Int. J. Hydrogen. Energy. 2020, 45, 19206-13.
93. Miao, F.; Cui, P.; Gu, T.; Yu, S.; Yan, Z.; Hai, G. Dual cation-modified hierarchical nickel hydroxide nanosheet arrays as efficient and robust electrocatalysts for the urea oxidation reaction. Dalton. Trans. 2024, 53, 1599-606.
94. Periyasamy, S.; Subramanian, P.; Levi, E.; Aurbach, D.; Gedanken, A.; Schechter, A. Exceptionally active and stable spinel nickel manganese oxide electrocatalysts for urea oxidation reaction. ACS. Appl. Mater. Interfaces. 2016, 8, 12176-85.
95. Sharma, S.; Kadyan, P.; Sharma, R. K.; Kumar, N.; Grover, S. Progressive updates on nickel hydroxide and its nanocomposite for electrochemical electrode material in asymmetric supercapacitor device. J. Energy. Storage. 2024, 87, 111368.
96. Ge, J.; Kuang, J.; Xiao, Y.; Guan, M.; Yang, C. Recent development of nickel-based catalysts and in situ characterization techniques for mechanism understanding of the urea oxidation reaction. Surf. Interfaces. 2023, 41, 103230.
97. Yang, K.; Hao, L.; Hou, Y.; Zhang, J.; Yang, J. Summary and application of Ni-based catalysts for electrocatalytic urea oxidation. Int. J. Hydrogen. Energy. 2024, 51, 966-81.
98. Jeon, S. S.; Lim, J.; Kang, P. W.; Lee, J. W.; Kang, G.; Lee, H. Design principles of NiFe-layered double hydroxide anode catalysts for anion exchange membrane water electrolyzers. ACS. Appl. Mater. Interfaces. 2021, 13, 37179-86.
99. Jiang, L.; Pan, Y.; Zhang, J.; et al. Mo propellant boosting the activity of Ni-P for efficient urea-assisted water electrolysis of hydrogen evolution. J. Colloid. Interface. Sci. 2022, 622, 192-201.
100. Liu, H.; Zhu, S.; Cui, Z.; Li, Z.; Wu, S.; Liang, Y. Ni2P nanoflakes for the high-performing urea oxidation reaction: linking active sites to a UOR mechanism. Nanoscale 2021, 13, 1759-69.
101. Yun, W. H.; Das, G.; Kim, B.; Park, B. J.; Yoon, H. H.; Yoon, Y. S. Ni-Fe phosphide deposited carbon felt as free-standing bifunctional catalyst electrode for urea electrolysis. Sci. Rep. 2021, 11, 22003.
102. Vij, V.; Sultan, S.; Harzandi, A. M.; et al. Nickel-based electrocatalysts for energy-related applications: oxygen reduction, oxygen evolution, and hydrogen evolution reactions. ACS. Catal. 2017, 7, 7196-225.
103. Zeng, L.; Liu, Z.; Sun, K.; et al. Multiple modulations of pyrite nickel sulfides via metal heteroatom doping engineering for boosting alkaline and neutral hydrogen evolution. J. Mater. Chem. A. 2019, 7, 25628-40.
104. Guo, X.; Qiu, L.; Li, M.; et al. Accelerating the generation of NiOOH by in-situ surface phosphating nickel sulfide for promoting the proton-coupled electron transfer kinetics of urea electrolysis. Chem. Eng. J. 2024, 483, 149264.
105. Chang, F.; Gao, W.; Guo, J.; Chen, P. Emerging materials and methods toward ammonia-based energy storage and conversion. Adv. Mater. 2021, 33, 2005721.
106. Chen, L.; Shi, J. Chemical-assisted hydrogen electrocatalytic evolution reaction (CAHER). J. Mater. Chem. A. 2018, 6, 13538-48.
107. Fang, J. Y.; Zheng, Q. Z.; Lou, Y. Y.; et al. Ampere-level current density ammonia electrochemical synthesis using CuCo nanosheets simulating nitrite reductase bifunctional nature. Nat. Commun. 2022, 13, 7899.
108. Herron, J. A.; Ferrin, P.; Mavrikakis, M. Electrocatalytic oxidation of ammonia on transition-metal surfaces: a first-principles study. J. Phys. Chem. C. 2015, 119, 14692-701.
109. Hassan, N. S.; Jalil, A. A.; Saravanan, R.; et al. Recent progress on electrocatalysts in ammonia electrooxidation reaction for clean hydrogen production. J. Mater. Chem. A. 2024, 12, 23202-17.
110. Pillai, H. S.; Li, Y.; Wang, S. H.; et al. Interpretable design of Ir-free trimetallic electrocatalysts for ammonia oxidation with graph neural networks. Nat. Commun. 2023, 14, 792.
111. Zhu, M.; Yang, Y.; Xi, S.; et al. Deciphering NH3 adsorption kinetics in ternary Ni-Cu-Fe oxyhydroxide toward efficient ammonia oxidation reaction. Small 2021, 17, 2005616.
112. Samanta, R.; Shekhawat, A.; Sahu, P.; Barman, S. Review and perspective of nickel and its derived catalysts for different electrochemical synthesis reactions in alkaline media for hydrogen production. Energy. Fuels. 2024, 38, 73-104.
113. Wang, Y.; Ling, Y.; Wang, B.; et al. A review of progress in proton ceramic electrochemical cells: material and structural design, coupled with value-added chemical production. Energy. Environ. Sci. 2023, 16, 5721-70.
114. Katsounaros, I.; Figueiredo, M. C.; Calle-vallejo, F.; et al. On the mechanism of the electrochemical conversion of ammonia to dinitrogen on Pt(100) in alkaline environment. J. Catal. 2018, 359, 82-91.
115. Burshtein, T. Y.; Yasman, Y.; Muñoz-moene, L.; Zagal, J. H.; Eisenberg, D. Hydrazine oxidation electrocatalysis. ACS. Catal. 2024, 14, 2264-83.
116. Tong, Y.; Chen, P. Recent progress of advanced electrocatalysts for hydrogen production via hydrazine-assisted water electrolysis. Inorg. Chem. Front. 2024, 11, 6218-45.
117. Matsumoto, M.; Kano, H.; Suzuki, M.; Katagiri, T.; Umeda, Y.; Fukushima, S. Carcinogenicity and chronic toxicity of hydrazine monohydrate in rats and mice by two-year drinking water treatment. Regul. Toxicol. Pharmacol. 2016, 76, 63-73.
118. Zhu, W.; Gandi, N. A.; Wu, Q.; et al. Simultaneous electrocatalytic hydrogen production and hydrazine removal from acidic waste water. Chem. Eng. Sci. 2022, 258, 117769.
119. Attia, A. A.; Silaghi-Dumitrescu, R. Computational investigation of the initial two-electron, two-proton steps in the reaction mechanism of hydroxylamine oxidoreductase. J. Phys. Chem. B. 2014, 118, 12140-5.
120. Zhou, H.; Xiong, B.; Chen, L.; Shi, J. Modulation strategies of Cu-based electrocatalysts for efficient nitrogen reduction. J. Mater. Chem. A. 2020, 8, 20286-93.
121. Wang, Z.; Xu, L.; Huang, F.; et al. Copper-nickel nitride nanosheets as efficient bifunctional catalysts for hydrazine-assisted electrolytic hydrogen production. Adv. Energy. Mater. 2019, 9, 1900390.
122. Wen, H.; Chen, C.; Tang, P.; Wang, P. Ultrasmall nickel boride nanoparticles supported on reduced graphene oxide as a high-performance catalyst for hydrazine electrooxidation. Electrochim. Acta. 2023, 471, 143364.
123. Sakamoto, T.; Masuda, T.; Yoshimoto, K.; et al. NiO/Nb2O5/C hydrazine electrooxidation catalysts for anion exchange membrane fuel cells. J. Electrochem. Soc. 2017, 164, F229-34.
124. Uddin, M. E.; Kim, N. H.; Kuila, T.; Lee, S. H.; Hui, D.; Lee, J. H. Preparation of reduced graphene oxide-NiFe2O4 nanocomposites for the electrocatalytic oxidation of hydrazine. Compos. Part. B. Eng. 2015, 79, 649-59.
125. Askari, M. B.; Salarizadeh, P.; Beitollahi, H.; Tajik, S.; Eshghi, A.; Azizi, S. Electro-oxidation of hydrazine on NiFe2O4-rGO as a high-performance nano-electrocatalyst in alkaline media. Mater. Chem. Phys. 2022, 275, 125313.
126. Yang, X.; Bu, H.; Qi, R.; et al. Boosting urea-assisted water splitting over P-MoO2@CoNiP through Mo leaching/reabsorption coupling CoNiP reconstruction. J. Colloid. Interface. Sci. 2024, 676, 445-58.
127. Chen, X. H.; Fu, H. C.; Wu, L. L.; et al. Tuning the d-band center of NiC2O4 with Nb2O5 to optimize the Volmer step for hydrazine oxidation-assisted hydrogen production. Green. Chem. 2022, 24, 5559-69.
128. Rathore, D.; Banerjee, A.; Pande, S. Bifunctional tungsten-Doped Ni(OH)2/NiOOH nanosheets for overall water splitting in an alkaline medium. ACS. Appl. Nano. Mater. 2022, 5, 2664-77.
129. Ren, J.; Chen, L.; Wang, H.; Tian, W.; Yuan, Z. Water electrolysis for hydrogen production: from hybrid systems to self-powered/catalyzed devices. Energy. Environ. Sci. 2024, 17, 49-113.
130. Fan, G.; Li, F.; Evans, D. G.; Duan, X. Catalytic applications of layered double hydroxides: recent advances and perspectives. Chem. Soc. Rev. 2014, 43, 7040-66.
131. Babar, P.; Lokhande, A.; Karade, V.; et al. Trifunctional layered electrodeposited nickel iron hydroxide electrocatalyst with enhanced performance towards the oxidation of water, urea and hydrazine. J. Colloid. Interface. Sci. 2019, 557, 10-7.
132. Parra-puerto, A.; Ng, K. L.; Fahy, K.; Goode, A. E.; Ryan, M. P.; Kucernak, A. Supported transition metal phosphides: activity survey for HER, ORR, OER, and corrosion resistance in acid and alkaline electrolytes. ACS. Catal. 2019, 9, 11515-29.
133. Blanchard, P. E. R.; Grosvenor, A. P.; Cavell, R. G.; Mar, A. X-ray photoelectron and absorption spectroscopy of metal-rich phosphides M2P and M3P (M = Cr-Ni). Chem. Mater. 2008, 20, 7081-8.
134. Zhu, Y.; Lu, P.; Li, F.; Ding, Y.; Chen, Y. Metal-rich porous copper cobalt phosphide nanoplates as a high-rate and stable battery-type cathode material for battery-supercapacitor hybrid devices. ACS. Appl. Energy. Mater. 2021, 4, 3962-74.
135. Zhu, L.; Huang, J.; Meng, G.; et al. Active site recovery and N-N bond breakage during hydrazine oxidation boosting the electrochemical hydrogen production. Nat. Commun. 2023, 14, 1997.
136. Li, S. H.; Qi, M. Y.; Tang, Z. R.; Xu, Y. J. Nanostructured metal phosphides: from controllable synthesis to sustainable catalysis. Chem. Soc. Rev. 2021, 50, 7539-86.
137. Li, C.; Wang, Y.; Wang, X.; Azam, T.; Wu, Z. Electrochemical refining of energy-saving coupled systems toward generation of high-value chemicals. Chem 2024, 10, 2666-99.
138. Behera, S.; Ganguly, S.; Loha, C.; Mondal, B.; Ghosh, S. Critical role of interface design in acceleration of overall water splitting and hybrid electrolysis process: state of the art and perspectives. Energy. Fuels. 2023, 37, 7603-33.
139. Zhai, P.; Zhang, Y.; Wu, Y.; et al. Engineering active sites on hierarchical transition bimetal oxides/sulfides heterostructure array enabling robust overall water splitting. Nat. Commun. 2020, 11, 5462.
140. Yang, C.; Wang, T.; Li, C.; Li, Y.; Liu, D.; Zhang, Q. Recent progress on 2D material-based nanoarchitectures for small molecule electro-oxidation. Mater. Chem. Front. 2024, 8, 404-33.
141. Zhou, L.; Shao, M.; Zhang, C.; et al. Hierarchical CoNi-sulfide nanosheet arrays derived from layered double hydroxides toward efficient hydrazine electrooxidation. Adv. Mater. 2017, 29.
142. Moges, E. A.; Chang, C.; Tsai, M.; Su, W.; Hwang, B. J. Electrocatalysts for value-added electrolysis coupled with hydrogen evolution. EES. Catal. 2023, 1, 413-33.
143. Wu, Y.; Liu, X.; Han, D.; et al. Electron density modulation of NiCo2S4 nanowires by nitrogen incorporation for highly efficient hydrogen evolution catalysis. Nat. Commun. 2018, 9, 1425.
144. Liu, Y.; Zhang, J.; Li, Y.; Qian, Q.; Li, Z.; Zhang, G. Realizing the synergy of interface engineering and chemical substitution for Ni3N enables its bifunctionality toward hydrazine oxidation assisted energy-saving hydrogen production. Adv. Funct. Mater. 2021, 31, 2103673.
145. Li, K.; Zhou, G.; Tong, Y.; Ye, Y.; Chen, P. Interface engineering of a hierarchical p-modified Co/Ni3P heterostructure for highly efficient water electrolysis coupled with hydrazine degradation. ACS. Sustainable. Chem. Eng. 2023, 11, 14186-96.
146. Wang, X.; Hu, H.; Yan, X.; Zhang, Z.; Yang, M. Activating interfacial electron redistribution in lattice-matched biphasic Ni3N-Co3N for energy-efficient electrocatalytic hydrogen production via coupled hydrazine degradation. Angew. Chem. Int. Ed. 2024, 63, e202401364.
147. Dou, J.; Sun, Z.; Opalade, A. A.; Wang, N.; Fu, W.; Tao, F. F. Operando chemistry of catalyst surfaces during catalysis. Chem. Soc. Rev. 2017, 46, 2001-27.
148. Xu, Q.; Zhang, J.; Zhang, H.; et al. Atomic heterointerface engineering overcomes the activity limitation of electrocatalysts and promises highly-efficient alkaline water splitting. Energy. Environ. Sci. 2021, 14, 5228-59.
149. Su, J.; Zhang, S.; Liu, Q.; Hu, G.; Zhang, L. The janus in monodispersed catalysts: synergetic interactions. J. Mater. Chem. A. 2021, 9, 5276-95.
150. Chen, Y.; Ji, S.; Chen, C.; Peng, Q.; Wang, D.; Li, Y. Single-atom catalysts: synthetic strategies and electrochemical applications. Joule 2018, 2, 1242-64.
151. Wang, Y.; Su, H.; He, Y.; et al. Advanced electrocatalysts with single-metal-atom active sites. Chem. Rev. 2020, 120, 12217-314.
152. Huang, C.; Dong, J.; Sun, W.; et al. Coordination mode engineering in stacked-nanosheet metal-organic frameworks to enhance catalytic reactivity and structural robustness. Nat. Commun. 2019, 10, 2779.
153. Zhou, S.; Zhao, Y.; Shi, R.; et al. Vacancy-rich MXene-immobilized Ni single atoms as a high-performance electrocatalyst for the hydrazine oxidation reaction. Adv. Mater. 2022, 34, 2204388.
154. Liu, Y.; Zhang, J.; Li, Y.; et al. Manipulating dehydrogenation kinetics through dual-doping Co3N electrode enables highly efficient hydrazine oxidation assisting self-powered H2 production. Nat. Commun. 2020, 11, 1853.
155. Khalafallah, D.; Zhi, M.; Hong, Z. Development trends on nickel-based electrocatalysts for direct hydrazine fuel cells. ChemCatChem 2021, 13, 81-110.
156. Senthil, R. A.; Jung, S.; Min, A.; et al. Revealing the impact of pulsed laser-produced single-Pd nanoparticles on a bimetallic NiCo2O4 electrocatalyst for energy-saving hydrogen production via hybrid water electrolysis. ACS. Catal. 2024, 14, 3320-35.
157. Shih, Y.; Wu, Z.; Luo, J. Electrochemical ammonia oxidation on terephthalic acid framework (BDC-NH2) derived core-shell nickel oxide/nickel-enriched 2D carbon nanoflakes (NiO/Ni/CNF). Appl. Catal. B. Environ. Energy. 2024, 357, 124244.
158. Liao, W.; Zhao, Q.; Wang, S.; et al. Insights into mechanisms on electrochemical oxygen evolution substitution reactions. J. Catal. 2023, 428, 115161.
159. Zhai, Y.; Jin, C.; Xia, Q.; et al. Atomically confined Ru sites in octahedral Co3O4 for high-efficiency hydrazine oxidation. Adv. Funct. Mater. 2024, 34, 2311063.
160. Liu, G.; Nie, T.; Wang, H.; et al. Size sensitivity of supported palladium species on layered double hydroxides for the electro-oxidation dehydrogenation of hydrazine: from nanoparticles to nanoclusters and single atoms. ACS. Catal. 2022, 12, 10711-7.
161. Xu, X.; Chen, H. C.; Li, L.; et al. Leveraging metal nodes in metal-organic frameworks for advanced anodic hydrazine oxidation assisted seawater splitting. ACS. Nano. 2023, 17, 10906-17.
162. Hu, Y.; Chao, T.; Li, Y.; et al. Cooperative Ni(Co)-Ru-P sites activate dehydrogenation for hydrazine oxidation assisting self-powered H2 production. Angew. Chem. Int. Ed. 2023, 62, e202308800.
163. De, S.; Zhang, J.; Luque, R.; Yan, N. Ni-based bimetallic heterogeneous catalysts for energy and environmental applications. Energy. Environ. Sci. 2016, 9, 3314-47.
164. Nakaya, Y.; Furukawa, S. Catalysis of alloys: classification, principles, and design for a variety of materials and reactions. Chem. Rev. 2023, 123, 5859-947.
165. Nørskov, J. K.; Bligaard, T.; Hvolbaek, B.; Abild-Pedersen, F.; Chorkendorff, I.; Christensen, C. H. The nature of the active site in heterogeneous metal catalysis. Chem. Soc. Rev. 2008, 37, 2163-71.
166. Sun, F.; Qin, J.; Wang, Z.; et al. Energy-saving hydrogen production by chlorine-free hybrid seawater splitting coupling hydrazine degradation. Nat. Commun. 2021, 12, 4182.
167. Feng, G.; Kuang, Y.; Li, P.; et al. Single crystalline ultrathin nickel-cobalt alloy nanosheets array for direct hydrazine fuel cells. Adv. Sci. 2017, 4, 1600179.
168. Sun, Q.; Wang, L.; Shen, Y.; et al. Bifunctional copper-doped nickel catalysts enable energy-efficient hydrogen production via hydrazine oxidation and hydrogen evolution reduction. ACS. Sustainable. Chem. Eng. 2018, 6, 12746-54.
169. Zhang, Z.; Tang, P.; Wen, H.; Wang, P. Bicontinuous nanoporous Ni-Fe alloy as a highly active catalyst for hydrazine electrooxidation. J. Alloys. Compd. 2022, 906, 164370.
170. Prajapati, A.; Govindarajan, N.; Sun, W.; et al. Mechanistic insights into the electrochemical oxidation of 5-hydroxymethylfurfural on a thin-film Ni anode. ACS. Catal. 2024, 14, 10122-31.
171. Li, Z.; Han, Y.; Huang, B.; Xie, Z.; Wei, Q. Electrochemical oxidation of 5-hydroxymethylfurfural over a molybdenum sulfide modified nickel-based catalyst. Mater. Adv. 2023, 4, 2449-56.
172. Gupta, D.; Kafle, A.; Nagaiah, T. C. Dinitrogen reduction coupled with methanol oxidation for low overpotential electrochemical NH3 synthesis over cobalt pyrophosphate as bifunctional catalyst. Small 2023, 19, 2208272.
173. Simões, M.; Baranton, S.; Coutanceau, C. Electrochemical valorisation of glycerol. ChemSusChem 2012, 5, 2106-24.
174. Tuleushova, N.; Holade, Y.; Cornu, D.; Tingry, S. Glycerol electro-reforming in alkaline electrolysis cells for the simultaneous production of value-added chemicals and pure hydrogen - mini-review. Electrochem. Sci. Adv. 2023, 3, e2100174.
175. Xia, Z.; Ma, C.; Fan, Y.; et al. Vacancy optimized coordination on nickel oxide for selective electrocatalytic oxidation of glycerol. ACS. Catal. 2024, 14, 1930-8.
176. Chen, W.; Xie, C.; Wang, Y.; et al. Activity origins and design principles of nickel-based catalysts for nucleophile electrooxidation. Chem 2020, 6, 2974-93.
177. Huang, W.; Ma, X. Y.; Wang, H.; et al. Promoting effect of Ni(OH)2 on palladium nanocrystals leads to greatly improved operation durability for electrocatalytic ethanol oxidation in alkaline solution. Adv. Mater. 2017, 29.
178. Trafela, Š.; Zavašnik, J.; Šturm, S.; Žužek, R. K. Controllable voltammetric formation of a structurally disordered NiOOH/Ni(OH)2 redox pair on Ni-nanowire electrodes for enhanced electrocatalytic formaldehyde oxidation. Electrochim. Acta. 2020, 362, 137180.
179. Xu, L.; Yang, Y.; Li, C.; et al. Unveiling the mechanism of electrocatalytic oxidation of glycerol by in-situ electrochemical spectroscopy. Chem. Eng. J. 2024, 481, 148304.
180. Wang, S.; Yan, Y.; Du, Y.; et al. Inhibiting the deep reconstruction of Ni-based interface by coordination of chalcogen anions for efficient and stable glycerol electrooxidation. Adv. Funct. Mater. 2024, 34, 2404290.
181. Li, J.; Tian, X.; Wang, X.; et al. Electrochemical Conversion of alcohols into acidic commodities on nickel sulfide nanoparticles. Inorg. Chem. 2022, 61, 13433-41.
182. Jiang, R.; Tran, D. T.; Mcclure, J. P.; Chu, D. A class of (Pd-Ni-P) electrocatalysts for the ethanol oxidation reaction in alkaline media. ACS. Catal. 2014, 4, 2577-86.
183. Araujo, R. B.; Martín-yerga, D.; Santos, E. C. D.; Cornell, A.; Pettersson, L. G. Elucidating the role of Ni to enhance the methanol oxidation reaction on Pd electrocatalysts. Electrochim. Acta. 2020, 360, 136954.
184. Luo, H.; Yukuhiro, V. Y.; Fernández, P. S.; et al. Role of Ni in PtNi bimetallic electrocatalysts for hydrogen and value-added chemicals coproduction via glycerol electrooxidation. ACS. Catal. 2022, 12, 14492-506.
Cite This Article
How to Cite
Download Citation
Export Citation File:
Type of Import
Tips on Downloading Citation
Citation Manager File Format
Type of Import
Direct Import: When the Direct Import option is selected (the default state), a dialogue box will give you the option to Save or Open the downloaded citation data. Choosing Open will either launch your citation manager or give you a choice of applications with which to use the metadata. The Save option saves the file locally for later use.
Indirect Import: When the Indirect Import option is selected, the metadata is displayed and may be copied and pasted as needed.
About This Article
Special Issue
Copyright
Data & Comments
Data
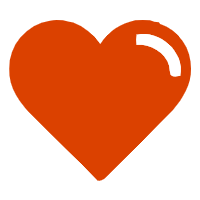
Comments
Comments must be written in English. Spam, offensive content, impersonation, and private information will not be permitted. If any comment is reported and identified as inappropriate content by OAE staff, the comment will be removed without notice. If you have any queries or need any help, please contact us at [email protected].