Regulation and function of the RSK family in colorectal cancer
Abstract
P90 Ribosomal S6 Kinases (RSKs) constitute a class of Serine/Threonine (Ser/Thr) protein kinases and play a critical role as downstream targets in the Raf/MEK/ERK signaling pathway. Gaining insight into the biological function of RSK family proteins, given their functions in various tumors, is vital. The RSK family is involved in the regulation of cellular functions including cell proliferation, motility, invasion, and survival. The RSK family comprises four human isoforms (RSK1, RSK2, RSK3, and RSK4). The activation of RSK protein kinases is mediated through direct phosphorylation by extracellular signal-regulated kinase 1/2 (ERK1/2) and phosphoinositide-dependent kinase 1 (PDK1) after the Ras/MAPK (mitogen-activated protein kinase) pathway is activated. Recent evidence suggests that RSK family proteins promote the onset and metastasis of cancer, leading to the association of the protein expression of these kinases with various cancer types, such as colorectal cancer (CRC), breast cancer, lung cancer, kidney cancer, leukemia, esophageal squamous cell carcinoma, ovarian cancer, glioma, and endometrial cancer. In this review, we summarize the latest research on the RSK family, focusing on its role in patients with CRC, along with associated treatment challenges and limitations. This information enhances our comprehension of the regulation and function of RSK family proteins, highlighting their potential as both clinical biomarkers for diagnosing CRC and targets for molecular therapeutic interventions in the future.
Keywords
INTRODUCTION
CRC ranks as the third most prevalent cancer globally[1]. The global incidence of CRC is increasing, and it is estimated that there will be approximately 2.5 million CRC patients by 2035[2]. Despite standard treatment modalities such as surgery, radiotherapy, and chemotherapy, the prognosis of CRC remains unfavorable, with a 5-year survival rate hovering around 50%-60%[1]. Therefore, in-depth research into the pathogenesis of CRC is of paramount importance for more efficient diagnosis, development of treatment, and prognosis. A better understanding of the pathogenesis of CRC is crucial for exploring novel therapies.
RSKs form a class of Ser/Thr kinases positioned downstream of the Ras-MAPK cascade[3]. The RSK family comprises four human isoforms (RSK1, RSK2, RSK3, RSK4)[4]. RSKs exhibit significant sequence homology and feature two distinct functional kinase domains[5]. RSKs phosphorylate numerous cytoplasmic and nuclear substrates, playing crucial roles in the regulation of cell proliferation, motility, invasion, and survival[6].
The ribosomal S6 kinase was first identified and named S6K in 1985 when Erikson et al. purified a protein kinase specific for ribosomal protein S6 from Xenopus laevis eggs. Moreover, S6K can phosphorylate all sites in S6 related to stimulus-induced growth[7] [Figure 1]. Later, Jones et al. cloned two protein kinases (S6KI and S6KII) and renamed them P90 RSK/RSKs[5]. Alcorta et al. isolated the mouse homolog (RPS6KA1/RSK1) of Xenopus laevis cDNA, and a partial cDNA fragment encoded a second murine RSK isoform (RPS6KA3/RSK2). Analyses also revealed the two-domain structure of RSKs[8]. RPS6KA2/RSK3 was a novel pp90rsk Ser/Thr kinase cloned from a human cDNA library that was translocated to the nucleus and potentially phosphorylated nuclear substrates[9]. RPS6KA6/RSK4 is a novel gene isolated from cosmids derived from a yeast artificial chromosome (YAC), including the critical interval located in the nonspecific X-linked mental retardation (MRX) gene[10]. In addition, two novel protein kinases in the NCBI EST database that were activated in vitro and in vivo by either MAPK/ERKs or SAPK2/p38 were identified and named MSK1/2, which regulated the activation of cAMP-response element-binding protein (CREB), the transcription factors, and activating transcription factor 1 (ATF1) through growth factors or stress-related signaling[11].
Figure 1. Discovery of the RSKs. Timeline depicting the key milestones in the discovery of the RSKs, including the discovery of Ribosomal S6 Kinase (S6K), Ribosomal S6 Kinase (RSKs), RPS6KA1 (RSK1), RPS6KA3 (RSK2), RPS6KA2 (RSK3), RPS6KA5 (MSK1), RPS6KA4 (MSK2) and RPS6KA6 (RSK4).
Following the aforementioned discoveries, an increasing number of studies have provided compelling evidence indicating increased or decreased RSK expression in malignant tumor tissues, including breast cancer[12,13], lung cancer[14], CRC[15], kidney cancer[16], head and neck squamous cell carcinoma (HNSCC)[17] and ovarian cancer[18]. We provide a summary of RSK expression in specific tumor issues. Overall, these studies revealed a significant role for RSK family proteins in tumorigenesis, with RSK protein expression levels positively or negatively correlating with clinical prognosis. In 2014, Cai et al. discussed the correlation between low expression of RSK4 in CRC tissues and poor patient prognosis[15]. In addition to the expression of RSK family proteins in tumor tissues and its correlation with clinical prognosis, understanding the regulation and function of the RSK family in cancer is essential for our comprehension of its role in tumorigenesis and metastasis. RSKs play different roles in promoting cancers, including nuclear signaling, cell proliferation, cell-cycle progression, cell growth, protein synthesis, cell migration, and cell survival[19]. FMS-like tyrosine kinase 3 - internal tandem duplications (FLT3-ITD) promotes the proliferation and survival of acute myelocytic leukemia (AML) by activating the MEK/ERK pathway and PDK1 to significantly activate RSK1/2[20][Table 1].
RSK family expression in specific tumor tissues
Increased expression | Decreased expression | |
RSK1 | Lung adenocarcinomas[14], Leukemia[20], CRC[21], Renal cancer[22], Ovarian cancer[23], Glioma[24] | Breast cancer[25] |
RSK2 | Glioma[24], Breast cancer[25,26], Lung cancer[27], Leukemia[28], Ovarian cancer[29] | CRC[30] |
RSK3 | - | Breast cancer[25], Lung cancer[31], Ovarian cancer[32] |
RSK4 | Renal cancer[33], Glioma[34,35] | Breast cancer[36], Lung cancer[37], CRC[38], Leukemia[39], Esophageal cancer[40], Ovarian cancer[41] |
In recent years, the regulation and function of the RSK family in CRC have gained much attention. Evidence has shown that the RSK family proteins regulate signaling pathways and thus participate in the biological activities underlying CRC. TBX21 inhibited CRC progression in an ARHGAP29/RSK/GSK3β dependent manner[42]. In addition, RSK family proteins are expressed in a specific pattern in CRC cells. Compared with that in normal colorectal tissues, RSK4 expression was markedly downregulated in CRC tissues, while RSK1 and RSK2 expression was not significantly different between these two tissue types[43].
In view of the multiple functions and specific expression patterns of RSK family proteins in these cancers, we have made great efforts to interfere with them to halt the progression of cancers. Since the first RSK inhibitor, SL0101, was identified, an increasing number of inhibitors have been discovered or designed to improve the prognosis of patients. Through various inhibitory compounds, including SL0101, BI-D1870, LJH685, LJI308, BIX02565, and fluoromethyl ketone (FMK), RSK activation can be inhibited[44,45]. However, limitations to the outcomes are clear and are not limited to the lack of RSK inhibitor selectivity and specificity, and as a result, clinical research progress on the RSK family has been hindered by the scarcity of advancements.
In this article, we discuss the latest advancements in the regulation and function of RSK family proteins in CRC. We hope that this review increases the understanding of this small yet important oncogene and provides insights for future research aimed at developing targeted therapies and biomarkers.
OVERVIEW OF THE RSK FAMILY
The RSK family is a group of Ser/Thr protein kinases that are important downstream targets in the Raf/MEK/ERK signaling pathway. As a class of Ras/MAPK kinases, the RSK family is widely expressed in mammalian cells and tissues. These RSK isoforms regulate cell proliferation through their interactions and the gene signaling that they regulate. The RSK family comprises four human isoforms, RSK1, RSK2, RSK3, and RSK4.
The variation in tissue expression levels implies distinct functions of RSK isoforms. RSK1 exhibits predominant expression in the cerebellum, lung, kidney, and pancreas. RSK2 demonstrates elevated expression in skeletal muscle, the pancreas, the heart, the cerebellum, the occipital pole, and the frontal lobes, while RSK3 is primarily found in skeletal muscle, the heart, the pancreas, and the medulla. In mice, the expression of the RSK1 gene is notably elevated in tissues characterized by a high proliferative capacity, and similarly, the RSK2 gene is highly expressed in tissues with high synaptic activity, including the neocortex, hippocampus, and Purkinje cells. In addition, the expression of the RSK3 gene is pronouncedly high in developing neural and sensory tissues[46]. In contrast, the expression of RSK4 is considerably lower than that of RSK1-3 and is expressed in the whole brain, cerebellum, heart, skeletal muscle, and kidney tissue of adult mice[47].
Structures of RSK family proteins
The RSK family proteins share a highly similar structure, with amino acid homology ranging from 80% to 85% among its four isoforms[4] [Figure 2]. The structure of RSK is distinguished by two separate kinase domains - a carboxyl-terminal kinase domain (CTKD) and an amino-terminal kinase domain (NTKD). These domains are separated by a linker region of approximately 100 amino acids, with the C- and N-terminal regions flanking each end of the linker[48]. These RSK proteins exhibit a substantial similarity, with identity ranging from 73% to 80% among them, primarily differing in their C- and N-terminal sequences[44]. The CTKD bears resemblance to the C-terminal domain (CTD) of the calcium/calmodulin-dependent protein kinase (CaMK) family, whereas the NTKD shares homology with that of AGC kinases (PKA, PKC, PKG). The CTKD functions in RSK autophosphorylation, while the NTKD is involved in phosphorylating substrates[48]. Furthermore, the D-domain, a crucial ERK1/2-docking site, is located in the C-terminal region, where it facilitates the docking and activation of RSK by ERK1/2[44,49]. It conforms to the kinase interaction motif (KIM) consensus sequence[50]. In addition, RSKs carry a type 1 PDZ (PSD-95/Dlg/ZO-1) motif for domain-binding(Ser-Thr-Xaa-Leu), resulting in interactions with PDZ domain-containing proteins[51].
Figure 2. The domain structure of RSKs. RSK1-4 are distinguished by two functional domains - CTKD and NTKD, separated by a linker region. The C-terminal region includes D-domain, a crucial ERK1/2-docking site.
After the stimulation of mitogen, ERK1/2 phosphorylates Thr573, RSK’s CTKD activation loop, causing the activation of CTKD[52] [Figure 3]. This necessitates a Pro-directed ERK consensus sequence in the RSK activation loop as well as ERK docking on the RSK C-terminal D domain[51,53]. ERK1/2 may also make the turn motif (Ser363) and Thr359 phosphorylated in the RSK linker region. Subsequently, the activated CTKD is phosphorylated on a hydrophobic motif, establishing for PDK1 a docking site. Following binding, Ser221 is phosphorylated by PDK1, the NTKD activation loop in RSK, resulting in complete RSK protein activation, and then RSK substrates phosphorylation via the NTKD. In addition, Ser749 is phosphorylated by the NTKD in the D domain, modulating the interaction of RSK isoforms with ERK1/2. This series of coordinated phosphorylation events and protein-protein interactions eventually lead to RSK activation and subsequent signaling[52]. In detail, CTKD activates NTKD through hydrophobicity-related motif phosphorylation[54,55]. The interaction between RSK and ERK1/2 is mediated by the Leu-Arg-Gln-Arg-Arg motif in the D domain. RSK autophosphorylation near the D domain promotes both the dissociation of ERK1/2, potentially regulating the duration of RSK activity and/or subcellular localization. Both RSK1 and RSK2 in the inactive state exhibited a higher affinity for ERK1/2 compared with either RSK following mitogen stimulation. During the activation of ERK1/2, the complex formed between RSK and ERK temporarily dissociates and then reassembles after signaling is terminated[51].
Figure 3. The activation of the RSK family. The RSK protein kinases undergo activation through direct phosphorylation triggered by the Ras/MAPK pathway. This activation can be impeded by inhibiting MEK (PD98059, PD184352 and U0126), ERK (SCH772984) and the RSK family itself (SL0101, BI-D1870, LJH685, LJI308, BIX02565 and FMK).
Functions of RSK family proteins
Accumulating convincing evidence shows that RSK family proteins regulate nuclear signaling, cell-cycle progression, cell proliferation, cell growth, protein synthesis, cell migration, and cell survival[19]. The RSK family modulates nuclear protein activity associated with the inflammatory response, including the transcription factor nuclear factor κB (NF-κB). RSK1 phosphorylates the protein inhibitor of NF-κBα (IκBα) and IκBβ on sites related to their facilitated degradation, thus increasing the activity of NF-κB[56,57]. The RSK family comprises important effectors of ERK in global transcription regulation. The RSK family regulates approximately 20% of ERK-controlled mRNA in Madin–Darby canine kidney (MDCK) cells, involving mechanisms such as the activation of transcription factors[19]. The RSK family participates in cell proliferation by regulating cell cycle mediators. RSK2 has been demonstrated to enhance cell cycle progression through the phosphorylation of c-Fos, a transcription factor responsible for positively regulating the expression of cyclin D1 during the G1/S transition[58]. Mammalian target of rapamycin (mTOR) participates in ribosome biogenesis, mRNA translation, and cell growth, and multiple growth-regulatory pathways influence its activity. By phosphorylating tuberous sclerosis complex 2 (TSC2) on Ser1798, activated RSK increases mTOR signaling, inhibiting guanine nucleotide-activating protein (GAP) activity towards the small GTPase Rheb[59]. RSK1/2 stimulated cell motility and invasion by the activation of a transcriptional program that regulated the intracellular motility apparatus, the extracellular environment, and receptors mediating interactions between these compartments[19]. In addition, the RSK family has been observed to have a positive impact on cell survival through various mechanisms, including an increase in transcription of survival-promoting genes dependent on CREB, the activation of NF-κB, and the inhibition of death-associated protein kinase (DAPK) pro-apoptotic activity through phosphorylation on Ser289[19]. In recent decades, RSK1-3 have been extensively studied, and their roles as crucial regulatory factors downstream of the ERK signaling pathway have been reported[51,60,61]. For example, activation of the ERK1/2 signaling pathway by parathyroid hormone-related peptide promotes RSK phosphorylation to induce colorectal cancer metastasis[62]. After stimulation by various factors, RSK1-3 can be translocated from the cytoplasm to the nucleus, where they promote cell growth, proliferation, and differentiation[63]. In contrast, fewer studies have explored the regulation and function of RSK4. RSK4 may function as an oncogene by inhibiting the action of specific ERK-targeted transcription-related kinases.
RSK ISOFORMS AND CRC
Although RSK1-4 share a high degree of sequence identity in their kinase domains, there are variations in their N- and C-terminal regions, indicating their regulating specific functions[64]. In terms of RSK isoform expression, RSK1 mRNA exhibited high expression in the lungs, the pancreas, kidneys, the bone marrow, and T cells. Most RSK2 mRNA was expressed in T cells, lymph nodes, and prostate tissue. RSK3 mRNA was primarily detected in the lungs and skeletal muscle[65]. However, compared to the other three isoforms, RSK4 mRNA is expressed at significantly lower levels in both embryonic and adult tissues[19,46].
Expression of RSK family proteins and their correlations with clinical prognosis in CRC
The RSK family proteins exhibit specific levels of expression and correlation with the clinical prognosis of patients with CRC. Peng et al. explored the role of tumor necrosis factor receptor-associated factor 2 (TRAF2) in mediating the epidermal growth factor (EGF) -induced signal transduction pathway and found that compared with their levels in normal colorectal tissue, TRAF2 and RSK2 were both overexpressed in CRC tissue[66] [Figure 4], and therefore, the expression of these proteins may be potential molecular indicators for CRC diagnosis. RSK4 was expressed at a low level in CRC tissue. A study revealed that the RSK4 protein expression had a close correlation with the clinical pathologic classifications of tumor differentiation (G), lymph node metastasis (N), and clinical staging levels in patients with CRC, and patients exhibiting high expression of RSK4 tended to have a worse prognosis than those with low-level expression. Therefore, it was suggested that low RSK4 expression in CRC may promote tumor progression, leading to poor prognosis. RSK4 may be an independent factor and tumor marker for the prediction of prognosis in patients with CRC[15].
The RSK family in the proliferation of CRC cells
In addition, RSK4 may play a notable role in the regulation of senescence and immortalization in CRC cells[16]. Elimination of RSK4 appeared to suppress p53-dependent cell cycle arrest and decrease the mRNA expression of the cyclin-dependent inhibitor p21. Inhibition of RSK4 resulted in premature senescence of CRC cells triggered by DNA damage, oxidative stress, and oncogene activation[67]. Therefore, RSK4 potentially serves as a meaningful tumor suppressor gene, as it participates in regulating CRC cell senescence induction and contributes to CRC cell proliferation control[16].
The RSK family in CRC metastasis
In terms of tumor metastasis, studies have indicated that RSK2 phosphorylates T-bet, thereby mitigating metastasis and growth of colon cancer. The absence of RSK2 can significantly reduce interferon-gamma (IFN-γ) secretion levels because of the inappropriate phosphorylation state of a regulator of IFN-γ expression called T-bet. Reduced IFN-γ levels can contribute to immune response suppression, thereby promoting liver and lung metastasis of colon cancer cells. T-bet phosphorylation at serines 498 and 502 mediated by RSK2 was important for suppressing colon cancer cell metastasis and CRC tumor growth, as it positively regulated the RSK2/T-bet/IFN-γ signaling pathway[30]. Additionally, Ye et al. concluded that the RSK4 gene may inhibit the epithelial-mesenchymal transition (EMT) in the CRC context and metastasis of CRC cells, which may indicate the potential of RSK4 as an inhibitor of tumor progression and metastasis[38].
The RSK family in the drug resistance of CRC cells
BRAF-oncogene mutations are evident in approximately 10% of CRC patients, with a valine 600 (V600) mutation closely associated with poor prognosis[68,69]. BRAF mutations exhibit higher prevalence among females, older individuals, and are more commonly found in right-sided colon tumors, particularly those in proximal locations, showing poor differentiation, mucinous histology, and infiltrating lymphocytes, often indicating MMR deficiency (MSI-H)[70]. The presence of a BRAF mutation correlates with shorter disease-free and overall survival in MMR-proficient (MSI-L) tumors. Intriguingly, this mutation leads to improved and extended disease-free survival in MSI-H tumors; however, its impact on overall survival appears to be not significantly altered. Moreover, BRAF mutation serves as a potential therapeutic target in metastatic CRC. However, targeting BRAF necessitates concurrent blockade of other pathways, such as epidermal growth factor receptor (EGFR), MEK, and PI3K. This approach differs from melanoma and non-small cell lung cancer treatments, where BRAF inhibition alone produces therapeutic benefits[71,72]. BRAF V600E-type CRC cells primarily induce chemotherapy resistance through autophagy. This resistance mechanism involves blocking the MEK/ERK/RSK signaling pathway to inhibit LKB1 phosphorylation, thereby activating AMP-dependent protein kinase (AMPK)-mediated autophagy[73]. However, further research is required to fully understand the relationship between RSK and drug resistance and the underlying mechanism in the CRC context.
RSK INHIBITORS
Considering that RSK is activated by ERK1/2, MEK1/2 inhibitors such as PD98059, PD184352, U0126, and SCH772984 are able to prevent RSK activation in cells[74]. The majority of RSK inhibitors are targeted to the CTKD or NTKD. We provide the chemical structures of several RSK inhibitors currently under investigation[75] [Figure 5] and a summary of their characteristics [Table 2].
Figure 5. Chemical structures of RSK inhibitors. Chemical structures of several RSK inhibitors, including SL0101, BI-D1870, LJH685, LJI308, BIX02565, and FMK, are provided in this figure.
RSK inhibitors and their characteristics
Inhibitor | Characteristics | IC50 | EC50 | References |
SL0101 | The first identified RSK inhibitor; Compound instability and low active state in vivo | 89 nmol/L RSK2 | 50 μmol/L | [12,76] |
BI-D1870 | RSK1-4 inhibitor; Poor drug stability, high clearance, and a short plasma half-life | 31 nmol/L RSK1 24 nmol/L RSK2 18 nmol/L RSK3 15 nmol/L RSK4 | 1 μmol/L | [77,78] |
LJH685 | Derivatives of BI-D1870; Fewer off-target effects; Poor pharmacokinetics | 6 nmol/L RSK1 5 nmol/L RSK2 4 nmol/L RSK3 | 730-790 nmol/L | [79,80] |
LJI308 | 6 nmol/L RSK1 4 nmol/L RSK2 13 nmol/L RSK3 | 200-300 nmol/L | ||
BIX02565 | RSK2 inhibitor; Poor selectivity | 1 nmol/L RSK2 | - | [81,82] |
FMK | RSK1/2/4 inhibitor; Only inhibit RSK activation | 15 nmol/L RSK2 | 200 nmol/L | [83-85] |
SL0101 was the first identified RSK inhibitor[12]. Although numerous analogs of SL0101 have been designed and have exerted negative effects on breast cancer cell growth[86], these analogs have been reported to possibly promote cell invasion in non-small cell lung cancer (NSCLC)[44]; these inhibitors include SL0101 5a-carbasugar analogs[87], C6″-substituted 5a-carbasugar analogs[88], C-4″ carbamate, and a C-6″ n-Pr substituted cyclitol analog[89]. Moreover, there have been observations of compound instability and low activity in vivo[76].
BI-D1870 is a comprehensive RSK inhibitor and exhibits particularly potent inhibition of activated RSK3 and RSK4[77]. By exploiting structural differences in the NTKD between RSK isoforms, isoform-selective RSK inhibitors can be designed. For example, exposure of A549 lung adenocarcinoma cells to BI-D1870 led to decreased RSK1 protein expression, which was negatively associated with cell migration and proliferation[90]. Nevertheless, BI-D1870 shows irreparable drawbacks, including high clearance, poor drug stability, and a short plasma half-life[78].
LJH308 and LJH685, which are derivatives of BI-D1870, have been designed[79]. They exert substantially fewer off-target effects than BI-D1870[79] but also show the disadvantage of poor pharmacokinetics[80]. Recent studies revealed that the combination of LJH-685 and FF-10101, the FLT3 inhibitor, exhibited antileukemic effects on AML cell lines with mutations of FLT3-ITD through the inhibition of the MAPK/RSKs/YB-1 pathway and suggested novel targets for future therapy, especially for AML patients with FLT3-ITD mutations[91].
Whether BIX02565, shown to target RSK2[81], can inhibit other RSK isoforms is unknown. Targeting NTKD kinase activity, BIX02565 significantly inhibited pS703-NHE1 and increased cardiac function after ischemia/reperfusion in mouse hearts[92]. Nevertheless, it showed poor selectivity in that BIX02565 was also found to inhibit CLK2, FLT3, LRRK2, PDGFR, and RET[82].
FMK establishes a covalent bond with a cysteine residue located in the CTKDs of RSK4, RSK2, and RSK1[83]. However, FMK at higher concentrations inhibited S6K1, EphA2, Src, and Lck activity[84]. In addition, FMK can inhibit only RSK activation since the activity of NTKD does not require the activation of the CTKD. Due to this limitation, even though RSK shows relatively high potency and specificity, the use of FMK has been limited[84].
DISCUSSION
CRC is the third most prevalent cancer, and patients with this cancer have the fourth highest mortality among those with the most prevalent and highest-incidence cancers worldwide[1,93]. Despite patients with old age being more susceptible to severe postoperative complications, there is not a unanimous agreement that age significantly influences survival rate. Prognosis among patients with old age might be influenced by various factors such as variations in the stage at presentation, preexisting comorbidities, tumor site, and the type of treatment administered[94]. In recent decades, although considerable advancements in surgery, radiotherapy, chemotherapy, immunotherapy, and targeted therapy have been shown, we have not seen revolutionary improvement in the prognosis of CRC, which has a low 5-year survival rate[1]. Recent studies have focused on specific molecular targets. Immune cell PD-L1 expression shows a significant increase in MSI-H CRC in comparison to tumors with MSI-L, without discernible distinctions among the various MSI-H molecular subtypes[95]. The suggested screening method for defective DNA mismatch repair involves immunohistochemistry (IHC) and/or MSI testing. Nevertheless, consolidating the technical and biological diversity inherent in MSI testing into actionable information presents challenges. Literature reports indicate that IHC testing of the mismatch repair machinery might yield varying results for a specific germline mutation, potentially attributed to somatic mutations[96]. Bevacizumab and cetuximab, which target vascular endothelial growth factor (VEGF) and EGFR, respectively, have shown a certain level of effectiveness and have been employed in first-line treatment for advanced CRC[97,98]. However, these drugs have been associated with adverse effects. A study on the impact of incorporating cetuximab into a combination consisting of capecitabine, oxaliplatin, and bevacizumab for metastatic CRC treatment revealed that overall survival was not significantly prolonged in the cetuximab group. The combination of cetuximab with capecitabine, oxaliplatin, and bevacizumab, in fact, led to significantly shorter progression-free survival (PFS). In addition, cetuximab seemed to be related to adverse cutaneous effects, leading to a considerable number of Grade 3 or 4 adverse events in CRC patients[99]. Therefore, further research needs to be undertaken to determine the pathogenesis and molecular targets of CRC.
In recent years, researchers have concentrated on the function of the Raf/MEK/ERK signaling pathway in CRC cells, as this pathway is crucial to cell proliferation, survival, and differentiation[100]. RSK serves as a downstream target and thus has gained much attention[3]. There are four isoforms in the human RSK family[4]. Upon stimulation, RSK1-3 undergo translocation from the cytoplasm to the nucleus, promoting cell growth, proliferation, and differentiation[63]. In contrast, RSK4 is found primarily in the cytoplasm and does not appear to be translocated into the nucleus under conditions similar to those that drive RSK1-3 translocation[16]. RSK4 may be a tumor suppressor that inhibits tumor cell invasion, proliferation, and metastasis[13,38,67].
Since the discovery that the RSK-regulating signaling pathway influences CRC progression, studies have been conducted to investigate the regulation and function of the RSK family in CRC. We suggest that several milestone events have accelerated the rapid advancements initiated by these studies. Despite a number of studies illustrating the function of the RSK family in cancers, few experiments have demonstrated their effects in patients with CRC. RSK2 action in EGF pathway regulation through RSK2/CREB/c-Fos signaling[66] led us to conclude that the RSK family is related to CRC. Afterward, a series of studies demonstrated that RSK family proteins exert comprehensive impacts on the biological mechanism underlying CRC. Another notable finding is the correlation between low RSK4 expression in CRC cells and poor prognosis[15]. RSK4 expression level was initially related to the clinical parameters of CRC patients, and incorporation of RSK4 among independent factors of CRC inspired the study of other RSK isoforms (RSK1, RSK2, and RSK3), and factors collaborating with RSK4 were correlated with clinical parameters of CRC patients, such as TNM stage and lymph node metastasis.
Despite the recent advancements mentioned above, deficiencies in RSK targeting have been uncovered. First, although evidence suggests a connection between the RSK family and CRC, the biological mechanisms and regulatory pathways remain incompletely understood. Further research is required to illustrate the roles of RSK isoforms and their interactions with other signaling molecules in the context of CRC. For example, although RSK4 may act as a potential tumor suppressor, there is a possibility that RSK4 has a dual role in CRC, similar to its role in breast cancer, and this hypothesis requires testing. Additionally, RSK2 and RSK4 have been reported to play specific roles in CRC, but there is currently limited information regarding the regulation and functions of RSK1 and RSK3 in this context. Furthermore, the RSK1 and RSK3 expression levels in CRC tissues remain unknown, which may guide the exploration of the connections between the RSK family proteins and signaling pathways in CRC, as well as their correlations with various clinical parameters. Second, CRC is a heterogeneous disease characterized by various molecular subtypes and genetic alterations. The roles of RSK family proteins in different subtypes of CRC and their interactions with signaling pathways may lead to disparate outcomes, making targeted therapies a challenging task. Third, there has been more research on other malignant tumors, such as breast cancer and lung cancer, compared to the few studies on CRC. Given the escalating interest in the RSK family in the CRC context, we anticipate an increasing number of studies on this topic in the future. Finally, clinical translation of RSK inhibitors has been limited to date. Although some studies have identified RSKs as potential therapeutic targets for CRC, the identified RSK inhibitors have had limited impacts to date. Therefore, the translation of RSK-targeted therapies into clinical applications is still in the early stages, and further efforts to develop effective RSK-targeted therapies for CRC patients are encouraged.
Based on the abovementioned points, the significance of exploring future research directions is self-evident. Future research may be concentrated on developing more effective RSK inhibitors and other RSK-related signaling molecules involved in CRC. In addition, the development of personalized medicine for CRC patients may involve identifying specific signaling pathways and gene mutations. RSK isoforms and other signaling molecules may be used as diagnostic or prognostic markers to guide treatment decisions. Advancements in experimental techniques such as CRISPR‒Cas9 gene editing and single-cell sequencing may provide novel perspectives into the regulation and function of RSK family proteins in CRC. This study offers researchers clues to identify new targets for therapeutic interventions and develop more effective treatments for CRC patients.
CONCLUSION
In summary, the RSK family is of paramount importance as a downstream target in the Raf/MEK/ERK signaling pathway. RSK family proteins play a role in the regulation of cell proliferation, motility, growth, survival, and invasion. Several RSK isoforms have been found to participate in the development of CRC. In future studies, the investigation of the biological regulation and function of RSK family proteins in CRC and RSK-specific small-molecule inhibitors are promising research directions. In light of the aforementioned considerations, it is evident that future research directions hold great promise for advancing our understanding and treatment of CRC, and further in-depth research will inspire new efforts to discover novel treatments for CRC, increase patient survival, and improve the prognosis for the long term.
DECLARATIONS
Authors’ Contribution
Conceptualized and designed the study: Wang Y, Guo L
Wrote, reviewed, and revised the manuscript: Wang Z, Wang Y
Developed the methodology and analyzed the data: Han X
Sorted out the tables: Lei Z, Shi C
Supervised the study: Guo L
Availability of data and materials
Not applicable.
Financial support and sponsorship
This work was supported by Suzhou Basic Research Key Project (No. SKY2023009), Suzhou health youth backbone talent “national tutorial system” training project (Qngg2023005) and Beijing Xisike Clinical Oncology Research Foundation (Y-tongshu2021/qn-0366).
Conflicts of interest
All authors declared that there are no conflicts of interest.
Ethical approval and consent to participate
Not applicable.
Consent for publication
Not applicable.
Copyright
© The Author(s) 2024.
REFERENCES
1. Siegel RL, Wagle NS, Cercek A, Smith RA, Jemal A. Colorectal cancer statistics, 2023. CA Cancer J Clin. 2023;73:233-54.
2. Yu GH, Li SF, Wei R, Jiang Z. Diabetes and colorectal cancer risk: clinical and therapeutic implications. J Diabetes Res. 2022;2022:1747326.
3. Poomakkoth N, Issa A, Abdulrahman N, Abdelaziz SG, Mraiche F. p90 ribosomal S6 kinase: a potential therapeutic target in lung cancer. J Transl Med. 2016;14:14.
4. Carriere A, Ray H, Blenis J, Roux PP. The RSK factors of activating the Ras/MAPK signaling cascade. Front Biosci. 2008;13:4258-75.
5. Jones SW, Erikson E, Blenis J, Maller JL, Erikson RL. A Xenopus ribosomal protein S6 kinase has two apparent kinase domains that are each similar to distinct protein kinases. Proc Natl Acad Sci USA. 1988;85:3377-81.
6. Houles T, Roux PP. Defining the role of the RSK isoforms in cancer. Semin Cancer Biol. 2018;48:53-61.
7. Erikson E, Maller JL. A protein kinase from Xenopus eggs specific for ribosomal protein S6. Proc Natl Acad Sci USA. 1985;82:742-6.
8. Alcorta DA, Crews CM, Sweet LJ, Bankston L, Jones SW, Erikson RL. Sequence and expression of chicken and mouse rsk: homologs of Xenopus laevis ribosomal S6 kinase. Mol Cell Biol. 1989;9:3850-9.
9. Moller DE, Xia CH, Tang W, Zhu AX, Jakubowski M. Human rsk isoforms: cloning and characterization of tissue-specific expression. Am J Physiol. 1994;266:C351-9.
10. Yntema HG, van den Helm B, Kissing J, et al. A novel ribosomal S6-kinase (RSK4; RPS6KA6) is commonly deleted in patients with complex X-linked mental retardation. Genomics. 1999;62:332-43.
11. Deak M, Clifton AD, Lucocq LM, Alessi DR. Mitogen- and stress-activated protein kinase-1 (MSK1) is directly activated by MAPK and SAPK2/p38, and may mediate activation of CREB. EMBO J. 1998;17:4426-41.
12. Smith JA, Poteet-Smith CE, Xu Y, Errington TM, Hecht SM, Lannigan DA. Identification of the first specific inhibitor of p90 ribosomal S6 kinase (RSK) reveals an unexpected role for RSK in cancer cell proliferation. Cancer Res. 2005;65:1027-34.
13. Thakur A, Sun Y, Bollig A, et al. Anti-invasive and antimetastatic activities of ribosomal protein S6 kinase 4 in breast cancer cells. Clin Cancer Res. 2008;14:4427-36.
14. Abdulrahman N, Jaballah M, Poomakkoth N, et al. Inhibition of p90 ribosomal S6 kinase attenuates cell migration and proliferation of the human lung adenocarcinoma through phospho-GSK-3β and osteopontin. Mol Cell Biochem. 2016;418:21-9.
15. Cai J, Ma H, Huang F, et al. Low expression of RSK4 predicts poor prognosis in patients with colorectal cancer. Int J Clin Exp Pathol. 2014;7:4959-70.
16. López-Vicente L, Armengol G, Pons B, et al. Regulation of replicative and stress-induced senescence by RSK4, which is down-regulated in human tumors. Clin Cancer Res. 2009;15:4546-53.
17. Kang S, Elf S, Lythgoe K, et al. p90 ribosomal S6 kinase 2 promotes invasion and metastasis of human head and neck squamous cell carcinoma cells. J Clin Invest. 2010;120:1165-77.
18. Bignone PA, Lee KY, Liu Y, et al. RPS6KA2, a putative tumour suppressor gene at 6q27 in sporadic epithelial ovarian cancer. Oncogene. 2007;26:683-700.
19. Romeo Y, Zhang X, Roux PP. Regulation and function of the RSK family of protein kinases. Biochem J. 2012;441:553-69.
20. Watanabe D, Nogami A, Okada K, Akiyama H, Umezawa Y, Miura O. FLT3-ITD activates RSK1 to enhance proliferation and survival of AML cells by activating mTORC1 and eIF4B cooperatively with PIM or PI3K and by inhibiting bad and BIM. Cancers. 2019;11:1827.
21. Jin G, Yan M, Liu K, et al. Discovery of a novel dual-target inhibitor against RSK1 and MSK2 to suppress growth of human colon cancer. Oncogene. 2020;39:6733-46.
23. Torchiaro E, Lorenzato A, Olivero M, et al. Peritoneal and hematogenous metastases of ovarian cancer cells are both controlled by the p90RSK through a self-reinforcing cell autonomous mechanism. Oncotarget. 2016;7:712-28.
24. M Hajj GN, da Silva FF, de Bellis B, et al. Aberrant expression of RSK1 characterizes high-grade gliomas with immune infiltration. Mol Oncol. 2020;14:159-79.
25. Zhao H, Martin TA, Davies EL, et al. The Clinical implications of RSK1-3 in human breast cancer. Anticancer Res 2016;36:1267-74. Avaliable from: https://ar.iiarjournals.org/content/36/3/1267 [Last accessed on 26 Jan].
26. Li LY, Chen XS, Wang KS, et al. RSK2 protects human breast cancer cells under endoplasmic reticulum stress through activating AMPKα2-mediated autophagy. Oncogene. 2020;39:6704-18.
27. Lee CJ, Lee MH, Yoo SM, et al. Magnolin inhibits cell migration and invasion by targeting the ERKs/RSK2 signaling pathway. BMC Cancer. 2015;15:576.
28. Katsuragawa-Taminishi Y, Mizutani S, Kawaji-Kanayama Y, et al. Triple targeting of RSK, AKT, and S6K as pivotal downstream effectors of PDPK1 by TAS0612 in B-cell lymphomas. Cancer Sci. 2023;114:4691-705.
29. van Jaarsveld MT, Blijdorp IC, Boersma AW, et al. The kinase RSK2 modulates the sensitivity of ovarian cancer cells to cisplatin. Eur J Cancer. 2013;49:345-51.
30. Yao K, Peng C, Zhang Y, et al. RSK2 phosphorylates T-bet to attenuate colon cancer metastasis and growth. Proc Natl Acad Sci USA. 2017;114:12791-6.
31. Kumari A, Gesumaria L, Liu YJ, et al. mTOR inhibition overcomes RSK3-mediated resistance to BET inhibitors in small cell lung cancer. JCI Insight. 2023;8:e156657.
32. Lin H, Morin PJ. A novel homozygous deletion at chromosomal band 6q27 in an ovarian cancer cell line delineates the position of a putative tumor suppressor gene. Cancer Lett. 2001;173:63-70.
33. Fan L, Li P, Yin Z, et al. Ribosomal s6 protein kinase 4: a prognostic factor for renal cell carcinoma. Br J Cancer. 2013;109:1137-46.
34. Chen L, Xu T, Jia Q, Wang X, Li M, Liang G. RSK4: a new prognostic factor in glioma. Pathol Res Pract. 2020;216:153020.
35. Pang F, Zhang L, Li M, et al. Ribosomal S6 protein kinase 4 promotes resistance to EZH2 inhibitors in glioblastoma. Cancer Gene Ther. 2023;30:1636-48.
36. Jiang Y, Ye X, Ji Y, et al. Aberrant expression of RSK4 in breast cancer and its role in the regulation of tumorigenicity. Int J Mol Med. 2017;40:883-90.
37. Li A, Liu D, Liu Y, Zhou Y, Du Z, Song J. A pilot study of RSK4 expression in patients with human non-small cell lung carcinoma. Ann Clin Lab Sci. 2018;48:484-9.
38. Ye Q, Wang X, Jin M, et al. Effect of RSK4 on biological characteristics of colorectal cancer. World J Surg Oncol. 2018;16:240.
39. Rafiee M, Keramati MR, Ayatollahi H, et al. Down-regulation of ribosomal S6 kinase RPS6KA6 in acute myeloid leukemia patients. Cell J. 2016;18:159-64.
40. Li MY, Fan LN, Han DH, et al. Ribosomal S6 protein kinase 4 promotes radioresistance in esophageal squamous cell carcinoma. J Clin Invest. 2020;130:4301-19.
41. Arechavaleta-Velasco F, Zeferino-Toquero M, Estrada-Moscoso I, et al. Ribosomal S6 kinase 4 (RSK4) expression in ovarian tumors and its regulation by antineoplastic drugs in ovarian cancer cell lines. Med Oncol. 2016;33:11.
42. Jiang X, Du W, Yang C, et al. TBX21 attenuates colorectal cancer progression via an ARHGAP29/RSK/GSK3β dependent manner. Cell Oncol. 2023;46:1269-83.
43. Yang Q, Feng M, Ma X, Li H, Xie W. Gene expression profile comparison between colorectal cancer and adjacent normal tissues. Oncol Lett. 2017;14:6071-8.
44. Lara R, Seckl MJ, Pardo OE. The p90 RSK family members: common functions and isoform specificity. Cancer Res. 2013;73:5301-8.
45. Davies AH, Reipas K, Hu K, et al. Inhibition of RSK with the novel small-molecule inhibitor LJI308 overcomes chemoresistance by eliminating cancer stem cells. Oncotarget. 2015;6:20570-7.
46. Zeniou M, Ding T, Trivier E, Hanauer A. Expression analysis of RSK gene family members: the RSK2 gene, mutated in Coffin-Lowry syndrome, is prominently expressed in brain structures essential for cognitive function and learning. Hum Mol Genet. 2002;11:2929-40.
47. Dümmler BA, Hauge C, Silber J, et al. Functional characterization of human RSK4, a new 90-kDa ribosomal S6 kinase, reveals constitutive activation in most cell types. J Biol Chem. 2005;280:13304-14.
48. Anjum R, Blenis J. The RSK family of kinases: emerging roles in cellular signalling. Nat Rev Mol Cell Biol. 2008;9:747-58.
49. Smith JA, Poteet-Smith CE, Malarkey K, Sturgill TW. Identification of an extracellular signal-regulated kinase (ERK) docking site in ribosomal S6 kinase, a sequence critical for activation by ERK in vivo. J Biol Chem. 1999;274:2893-8.
50. MacKenzie SJ, Baillie GS, McPhee I, Bolger GB, Houslay MD. ERK2 mitogen-activated protein kinase binding, phosphorylation, and regulation of the PDE4D cAMP-specific phosphodiesterases. The involvement of COOH-terminal docking sites and NH2-terminal UCR regions. J Biol Chem. 2000;275:16609-17.
51. Roux PP, Richards SA, Blenis J. Phosphorylation of p90 ribosomal S6 kinase (RSK) regulates extracellular signal-regulated kinase docking and RSK activity. Mol Cell Biol. 2003;23:4796-804.
52. Dimitri CA, Dowdle W, MacKeigan JP, Blenis J, Murphy LO. Spatially separate docking sites on ERK2 regulate distinct signaling events in vivo. Curr Biol. 2005;15:1319-24.
53. Dalby KN, Morrice N, Caudwell FB, Avruch J, Cohen P. Identification of regulatory phosphorylation sites in mitogen-activated protein kinase (MAPK)-activated protein kinase-1a/p90rsk that are inducible by MAPK. J Biol Chem. 1998;273:1496-505.
54. Bjørbaek C, Zhao Y, Moller DE. Divergent functional roles for p90rsk kinase domains. J Biol Chem. 1995;270:18848-52.
55. Vik TA, Ryder JW. Identification of serine 380 as the major site of autophosphorylation of Xenopus pp90rsk. Biochem Biophys Res Commun. 1997;235:398-402.
56. Ghoda L, Lin X, Greene WC. The 90-kDa ribosomal S6 kinase (pp90rsk) phosphorylates the N-terminal regulatory domain of IkappaBalpha and stimulates its degradation in vitro. J Biol Chem. 1997;272:21281-8.
57. Xu S, Bayat H, Hou X, Jiang B. Ribosomal S6 kinase-1 modulates interleukin-1beta-induced persistent activation of NF-kappaB through phosphorylation of IkappaBbeta. Am J Physiol Cell Physiol. 2006;291:C1336-45.
58. David JP, Mehic D, Bakiri L, et al. Essential role of RSK2 in c-Fos-dependent osteosarcoma development. J Clin Invest. 2005;115:664-72.
59. Rolfe M, McLeod LE, Pratt PF, Proud CG. Activation of protein synthesis in cardiomyocytes by the hypertrophic agent phenylephrine requires the activation of ERK and involves phosphorylation of tuberous sclerosis complex 2 (TSC2). Biochem J. 2005;388:973-84.
60. Hsiao KM, Chou SY, Shih SJ, Ferrell JE Jr. Evidence that inactive p42 mitogen-activated protein kinase and inactive rsk exist as a heterodimer in vivo. Proc Natl Acad Sci USA. 1994;91:5480-4.
61. Zhao Y, Bjorbaek C, Moller DE. Regulation and interaction of pp90(rsk) isoforms with mitogen-activated protein kinases. J Biol Chem. 1996;271:29773-9.
62. Calvo N, Carriere P, Martin MJ, Gentili C. RSK activation via ERK modulates human colon cancer cells response to PTHrP. J Mol Endocrinol. 2017;59:13-27.
63. Clark DE, Errington TM, Smith JA, Frierson HF Jr, Weber MJ, Lannigan DA. The serine/threonine protein kinase, p90 ribosomal S6 kinase, is an important regulator of prostate cancer cell proliferation. Cancer Res. 2005;65:3108-16.
64. Méant A, Gao B, Lavoie G, et al. Proteomic analysis reveals a role for RSK in p120-catenin phosphorylation and melanoma cell-cell adhesion. Mol Cell Proteomics. 2020;19:50-64.
65. Zhao Y, Bjørbaek C, Weremowicz S, Morton CC, Moller DE. RSK3 encodes a novel pp90rsk isoform with a unique N-terminal sequence: growth factor-stimulated kinase function and nuclear translocation. Mol Cell Biol. 1995;15:4353-63.
66. Peng C, Zhu F, Wen W, et al. Tumor necrosis factor receptor-associated factor family protein 2 is a key mediator of the epidermal growth factor-induced ribosomal S6 kinase 2/cAMP-responsive element-binding protein/Fos protein signaling pathway. J Biol Chem. 2012;287:25881-92.
67. López-Vicente L, Pons B, Coch L, et al. RSK4 inhibition results in bypass of stress-induced and oncogene-induced senescence. Carcinogenesis. 2011;32:470-6.
68. Boussemart L, Malka-Mahieu H, Girault I, et al. eIF4F is a nexus of resistance to anti-BRAF and anti-MEK cancer therapies. Nature. 2014;513:105-9.
69. Lito P, Rosen N, Solit DB. Tumor adaptation and resistance to RAF inhibitors. Nat Med. 2013;19:1401-9.
70. Roth AD, Tejpar S, Delorenzi M, et al. Prognostic role of KRAS and BRAF in stage II and III resected colon cancer: results of the translational study on the PETACC-3, EORTC 40993, SAKK 60-00 trial. J Clin Oncol. 2010;28:466-74.
71. Barras D, Missiaglia E, Wirapati P, et al. BRAF V600E mutant colorectal cancer subtypes based on gene expression. Clin Cancer Res. 2017;23:104-15.
72. Boussios S, Ozturk MA, Moschetta M, et al. The developing story of predictive biomarkers in colorectal cancer. J Pers Med. 2019;9:12.
73. Sueda T, Sakai D, Kawamoto K, et al. BRAF V600E inhibition stimulates AMP-activated protein kinase-mediated autophagy in colorectal cancer cells. Sci Rep. 2016;6:18949.
74. Morris EJ, Jha S, Restaino CR, et al. Discovery of a novel ERK inhibitor with activity in models of acquired resistance to BRAF and MEK inhibitors. Cancer Discov. 2013;3:742-50.
75. Sun Y, Tang L, Wu C, Wang J, Wang C. RSK inhibitors as potential anticancer agents: discovery, optimization, and challenges. Eur J Med Chem. 2023;251:115229.
76. Hilinski MK, Mrozowski RM, Clark DE, Lannigan DA. Analogs of the RSK inhibitor SL0101: optimization of in vitro biological stability. Bioorg Med Chem Lett. 2012;22:3244-7.
77. Sapkota GP, Cummings L, Newell FS, et al. BI-D1870 is a specific inhibitor of the p90 RSK (ribosomal S6 kinase) isoforms in vitro and in vivo. Biochem J. 2007;401:29-38.
78. Pambid MR, Berns R, Adomat HH, et al. Overcoming resistance to sonic hedgehog inhibition by targeting p90 ribosomal S6 kinase in pediatric medulloblastoma. Pediatr Blood Cancer. 2014;61:107-15.
79. Aronchik I, Appleton BA, Basham SE, et al. Novel potent and selective inhibitors of p90 ribosomal S6 kinase reveal the heterogeneity of RSK function in MAPK-driven cancers. Mol Cancer Res. 2014;12:803-12.
80. Jain R, Mathur M, Lan J, et al. Discovery of potent and selective RSK inhibitors as biological probes. J Med Chem. 2015;58:6766-83.
81. Kirrane TM, Boyer SJ, Burke J, et al. Indole RSK inhibitors. Part 2: optimization of cell potency and kinase selectivity. Bioorg Med Chem Lett. 2012;22:738-42.
82. Boyer SJ, Burke J, Guo X, et al. Indole RSK inhibitors. Part 1: discovery and initial SAR. Bioorg Med Chem Lett. 2012;22:733-7.
83. Cohen MS, Zhang C, Shokat KM, Taunton J. Structural bioinformatics-based design of selective, irreversible kinase inhibitors. Science. 2005;308:1318-21.
84. Cohen MS, Hadjivassiliou H, Taunton J. A clickable inhibitor reveals context-dependent autoactivation of p90 RSK. Nat Chem Biol. 2007;3:156-60.
85. Bain J, Plater L, Elliott M, et al. The selectivity of protein kinase inhibitors: a further update. Biochem J. 2007;408:297-315.
86. Ludwik KA, Campbell JP, Li M, et al. Development of a RSK inhibitor as a novel therapy for triple-negative breast cancer. Mol Cancer Ther. 2016;15:2598-608.
87. Li M, Li Y, Mrozowski RM, et al. Synthesis and structure-activity relationship study of 5a-carbasugar analogues of SL0101. ACS Med Chem Lett. 2015;6:95-9.
88. Li M, Li Y, Ludwik KA, Sandusky ZM, Lannigan DA, O'Doherty GA. Stereoselective synthesis and evaluation of C6″-substituted 5a-carbasugar analogues of SL0101 as inhibitors of RSK1/2. Org Lett. 2017;19:2410-3.
89. Li Y, Sandusky ZM, Vemula R, et al. Regioselective synthesis of a C-4'' carbamate,C-6'' n-Pr substituted cyclitol analogue of SL0101. Org Lett. 2020;22:1448-52.
90. Abdulrahman N, Siveen KS, Joseph JM, et al. Inhibition of p90 ribosomal S6 kinase potentiates cisplatin activity in A549 human lung adenocarcinoma cells. J Pharm Pharmacol. 2020;72:1536-45.
91. Zhang S, Liu J, Lu ZY, et al. Combination of RSK inhibitor LJH-685 and FLT3 inhibitor FF-10101 promoted apoptosis and proliferation inhibition of AML cell lines. Cell Oncol. 2022;45:1005-18.
92. Shi X, O'Neill MM, MacDonnell S, Brookes PS, Yan C, Berk BC. The RSK inhibitor BIX02565 limits cardiac ischemia/reperfusion injury. J Cardiovasc Pharmacol Ther. 2016;21:177-86.
93. Chen W, Zheng R, Baade PD, et al. Cancer statistics in China, 2015. CA Cancer J Clin. 2016;66:115-32.
94. Osseis M, Nehmeh WA, Rassy N, et al. Surgery for T4 colorectal cancer in older patients: determinants of outcomes. J Pers Med. 2022;12:1534.
95. Luchini C, Bibeau F, Ligtenberg MJL, et al. ESMO recommendations on microsatellite instability testing for immunotherapy in cancer, and its relationship with PD-1/PD-L1 expression and tumour mutational burden: a systematic review-based approach. Ann Oncol. 2019;30:1232-43.
96. Adeleke S, Haslam A, Choy A, et al. Microsatellite instability testing in colorectal patients with Lynch syndrome: lessons learned from a case report and how to avoid such pitfalls. Per Med. 2022;19:277-86.
97. Grothey A, Sugrue MM, Purdie DM, et al. Bevacizumab beyond first progression is associated with prolonged overall survival in metastatic colorectal cancer: results from a large observational cohort study (BRiTE). J Clin Oncol. 2008;26:5326-34.
98. Cunningham D, Humblet Y, Siena S, et al. Cetuximab monotherapy and cetuximab plus irinotecan in irinotecan-refractory metastatic colorectal cancer. N Engl J Med. 2004;351:337-45.
99. Tol J, Koopman M, Cats A, et al. Chemotherapy, bevacizumab, and cetuximab in metastatic colorectal cancer. N Engl J Med. 2009;360:563-72.
Cite This Article

How to Cite
Download Citation
Export Citation File:
Type of Import
Tips on Downloading Citation
Citation Manager File Format
Type of Import
Direct Import: When the Direct Import option is selected (the default state), a dialogue box will give you the option to Save or Open the downloaded citation data. Choosing Open will either launch your citation manager or give you a choice of applications with which to use the metadata. The Save option saves the file locally for later use.
Indirect Import: When the Indirect Import option is selected, the metadata is displayed and may be copied and pasted as needed.
About This Article
Special Issue
Copyright
Data & Comments
Data
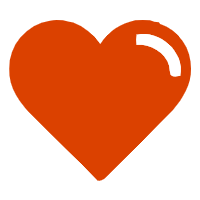
Comments
Comments must be written in English. Spam, offensive content, impersonation, and private information will not be permitted. If any comment is reported and identified as inappropriate content by OAE staff, the comment will be removed without notice. If you have any queries or need any help, please contact us at [email protected].