Promising future: a review of clinical applications of biopolymers in renal cell carcinoma
Abstract
Renal cell carcinoma (RCC), being the most common type of renal malignancy, accounting for 85% of its incidence, poses a significant threat to human health. Although progress has been made in RCC diagnosis and management, problems of delayed detection and treatment failure remain in traditional methods. In the development of material research, biopolymers have emerged as significant players in medical practices, demonstrating considerable potential in the management of kidney cancer. Specifically, bioactive polymers have been proven to have numerous advantages over traditional methods, finding prosperous applications in diverse areas such as imaging detection, chemotherapy carrier, and prognostic estimation. However, the clinical value of some biopolymers still needs to be fully recognized in the RCC clinical pathway. This review summarizes recent studies about bioactive polymers’ application in RCC and further discusses its significance and constraints in clinical translational medicine and clinical trials. As our understanding of biopolymers grows, more advancements can be carried out in clinical practices.
Keywords
INTRODUCTIONs
Globally, kidney cancer constitutes approximately 5% of all malignancies and ranks as the sixth most prevalent cancer among males[1]. Given recent advancements in diagnostic methodologies, malignancies originating from unknown primary sites (CUP), constituting approximately 1%-3% of all malignant neoplasms, are occasionally managed as renal cell carcinoma (RCC), which is the main type of kidney cancer. This practice could potentially contribute to the observed rise in kidney cancer incidence rates[2]. It also accounts for 3% of malignancies and ranks as the tenth most prevalent cancer among women[1]. It has been reported that pregnancy-related hormonal changes, especially high estrogen levels, may stimulate renal cell proliferation either directly or through paracrine growth factors, thereby promoting malignancy. Therefore, gynecologists should be alert to the possibility of malignancy in renal masses found during pregnancy[3]. Kidney cancer is the eighth most common new tumor in women and the fifth most common new tumor in males. According to the Global Cancer Statistics in 2023, the incidence of kidney cancer in males and females was 23.5 per 10,000 and 12 per 10,000 during 2015-2019, while the mortality from
A growing number of academics and businesses are undertaking in-depth investigations into medical polymer materials and integrating them into clinical practice due to the rise of polymer materials in medical applications. Meanwhile, nanoparticles are at the forefront of current medical polymer research investigating RCC. Nanobiotechnology facilitates precise molecular-level material manipulation to produce biocompatible products targeted for desired tissues and locations. Since the last century, there have been reports exploring the use of biopolymers, such as natural polymers, as drug carriers in disease treatment[5]. However, the application and development of biopolymers have not yet been widely acknowledged. With the continuous development of polymer materials and nanotechnology, in 2017, Nizioł et al. utilized gold nanoparticles to enhance target binding matrix-assisted laser desorption/ionization (MALDI) imaging mass spectrometry, enabling rapid visualization of differences between RCC and normal kidney tissue without the need for pathology[6]. This research has ignited interest and enthusiasm in the application of biopolymers for the diagnosis and treatment of RCC. In the context of RCC management, biopolymers demonstrate great potential over traditional solutions. Carefully designed bioactive nanoparticles can cross traditional biological barriers in vivo and bind selectively to biomarkers[7]. In addition, two or more therapeutic agents can be carried for synergistic effects and can be modulated to provide suitable circulation durations[8]. Those features are greatly effective in the therapy and diagnosis of kidney cancer. However, challenges persist, such as controlled drug release and cytotoxicity[9]. We present this review in the hope that it will serve as a guide for future clinical research in RCC.
Biopolymer materials in renal cell carcinoma research
Polymeric nanoparticles, lipid-based nanoparticles, inorganic nanoparticles, and mega polymers (such as plastics) are the most often employed biopolymer materials in RCC research[10]. In recent years, perioperative adjuvant therapy for high-risk RCC and advanced treatment have been hot topics in clinical research. The choice of immune-based combination therapy is increasingly supported in first-line treatment. Notably, the downregulation of lactotransferrin, an important protein in the innate immune system, promotes clear cell RCC metastasis. This downregulation made clear cell RCC tumor cells more sensitive to mTOR inhibitors, suggesting that it might serve as a predictor of the efficacy of immune-targeted therapies[11,12]. Combining basic cancer signaling research with biopolymer material studies to guide and develop novel diagnosis and treatment systems in RCC is a substantial challenge to all researchers in this field. Here, we present a summary of the studies investigating the role of different classes of biopolymers in the diagnosis and management of RCC. A brief illustration is given in Figure 1.
Figure 1. For renal cell carcinoma, all four major classes of polymers play a role in its clinical pathway. The giant polymer materials can also serve as a separate branch due to their remarkable properties. Polymers are used not only for laboratory diagnostics and diagnostic imaging, but also for radiation therapy, surgical markers, and so on. More innovatively, polymeric materials are also involved in gene therapy.
POLYMERIC NANOPARTICLES IN RENAL CELL CARCINOMA
Introduction of polymeric nanoparticles
Polymeric nanoparticles include a class of multifunctional polymer materials polymerized with organic substances, biologically active macromolecules (antibodies, nucleic acids), organic small molecules (vitamins), and other substances. Due to their diverse synthetic route options, polymeric nanoparticles possess easily modifiable and selectable physicochemical properties. These include various naturally occurring and synthetic materials, monomers or pre-formed polymers, and simple formulation parameters. They make excellent contenders for drug delivery as they possess traits such as biodegradability, water solubility, biocompatibility, biomimicry, storage stability, and ease of surface modification for further targeting. Furthermore, they have the capability to transport payloads of different molecular sizes, spanning from small compounds to biological macromolecules, including proteins and vaccines[13-15].
Polymeric NPs in targeted drug delivery
The hypoxic microenvironment and associated signaling pathways within RCC significantly influence their advancement, metastatic potential, and resistance to therapy. Extensive evidence suggests that within the hypoxic core of tumors, there exist highly aggressive, drug-resistant stem-like cells capable of surviving conventional drug treatments, infiltrating surrounding normal tissues, and establishing secondary tumors at distant locations. Vascular abnormalities, which are an important component of the tumor microenvironment, largely contribute to malignant phenotype, immune system evasion, and drug resistance in RCC carcinoma. Thus, targeting the RCC tumor microenvironment, such as current VEGFR-targeted therapies, holds great promise in preventing metastasis, overcoming acquired drug resistance, and improving efficacy. Monotherapy with tyrosine kinase inhibitors (TKIs) continues to be a suitable initial treatment for a significant subset of patients who are ineligible for immunotherapy, and the findings from the STAR study are directly relevant to this population. For other patients who do not undergo TKI therapy as a first-line option, it may be considered as monotherapy for second-line treatment. While treatment interruptions for these individuals might be contemplated, caution is warranted as they typically experience shorter progression-free survival compared to those receiving TKIs as initial therapy[16].
However, many patients’ tumors develop resistance to VEGFR-targeted drugs, resulting in poorer outcomes. How to solve the problem of drug resistance has become a hot issue in the treatment of RCC. The intractable reaction to anti-VEGFR treatment in most RCC patients is ultimately attributed to inadequate vascularization within the tumor core, which is linked to tumor hypoxia[17]. Carbonic anhydrase IX (CA IX) is a transmembrane protein that exhibits heightened expression on the surface of numerous cancerous cells within hypoxic conditions[18]. CA IX has a favorable distribution in RCC and is esteemed as a superb candidate for diagnostic applications and potentially for precision-targeted therapeutic modalities[19,20]. Alsaab et al. utilized a nanoparticle (NP) loaded with CFM 4.16 (C4.16), an apoptosis inducer, termed CA IX-C4.16, in conjunction with targeted tumor hypoxia delivery[21]. This NP was designed to transport the payload specifically to the hypoxic core of tumors, thereby inducing enhanced cellular death in both parental and Everolimus-resistant RCC cells, while selectively modulating tumor causing M2 macrophages. The simple procedure of this experiment is described briefly in Figure 2. For conjugation, "click" chemistry without copper linked SMA-TPGS with Acetazolamide, specifically targeting CA IX. Furthermore, the NP was labeled with a clinically approved near-infrared dye (S0456) to evaluate penetration into the hypoxic tumor cores and organ distributions. Imaging studies of tumor spheroids treated with NIR dye-labeled CA IX-SMA-TPGS demonstrated significant penetration into the tumor core, facilitated by CA IX which is targeting in hypoxic A498 cells. The synergistic combination of CA
Figure 2. Synopsis of tumor hypoxia-targeted nanotherapy combined with Sorafenib, demonstrating manifold advantages against cancer, including overcoming drug resistance, triggering apoptosis, and reshaping macrophage function[21].
Chen et al. innovatively developed H-NPs, an oxygen nanocarrier based on hemoglobin, to address hypoxia-induced drug resistance in RCC when used in conjunction with DAC[22]. These H-NPs, as shown in Figure 3, characterized by their hemoglobin-based composition, were crafted with meticulous attention using poly (lactic-co-glycolic acid) (PLGA) to encapsulate hemoglobin via hydrophobic interactions. Additionally, a specialized coating of low molecular weight hydroxyethyl chitosan was applied to facilitate renal targeting, thus enhancing the nanocarrier’s therapeutic efficacy in the context of RCC. Western blotting analysis of ENT1, which was treated with oxygen nanocarriers in 769-P under hypoxia, demonstrated a reduction in The hypoxia-induced downregulation phenotype of ENT1. This serves as evidence for the capacity of H-NPs to mitigate the diminution of DAC efficacy and enhance the sensitivity of RCC cells to consecutive combination therapy involving DAC and oxaliplatin. The above findings established an important base of clinic translational research and treatment studies in RCC.
Figure 3. Diagram illustrating the fabrication process and architecture of hemoglobin-derived nanocarriers[22]. PLGA: poly (lactic-co-glycolic acid).
Sunitinib represents an orally administered, multitargeted receptor tyrosine kinase inhibitor (TKI) that specifically inhibits the signaling pathways of vascular endothelial growth factor and platelet-derived growth factor receptors. As a representative of targeted therapy, sunitinib remains the recommended treatment option for patients with metastatic renal cell carcinoma (mRCC). However, approximately 60%-70% of patients with mRCC exhibit inherent resistance to sunitinib therapy, and even responding patients may relapse after 14 months of treatment. Zeng et al. synthesized an amphipathic conjugate known as sialic acid-poly-ibuprofen, which is capable of self-assembling into nanomicelles and encapsulating sunitinib in a watery solution[23]. Ibuprofen is a nonsteroidal anti-inflammatory drug and it is usually utilized to decrease COX-2 manufacturing. Notably, COX-2 has been implicated in both tumorigenesis and metastasis, and ibuprofen has shown some effectiveness in cancer treatment. Sialic acid (SA), another constituent of the conjugate, is a derivative of neuraminic acid that specifically targets E-selectin receptors. To evaluate the biodistribution of SPI nanomicelles, DiR-laden SPI nanomicelles and free DiR were administered intravenously to tumor-bearing mice. In vivo infrared imaging was conducted at specific time intervals (3, 6, 12, 24 h) post-administration to observe the biodistribution of the nanomicelles. The fluorescence signal of SPI/DiR nanomicelles within tumoral tissues was significantly stronger than that of the free DiR, indicating the superior tumor-targeting capability of SPI nanomicelles. Following this, the tumor treatment effects of sunitinib-loaded SA-PEG-IBU and control groups were evaluated in tumor-bearing nude mice models. Every group, including free sunitinib, free ibuprofen, SPI/SU, and PI/SU, exhibited a tumor suppression rate exceeding half. Remarkably, SPI/SU nanomicelles exhibited the most robust suppression of tumor proliferation, achieving an average inhibition rate of 80%. While the group of free sunitinib displayed tumoral inhibition, it was accompanied by significant body weight loss, suggesting systemic side effects or cachexia induced by free sunitinib. In contrast, body weight loss was not observed in the SPI/SU group during the 21-day treatment period, indicating minimal adverse effects. The remarkable efficacy in inhibiting tumor growth and reducing side effects may be attributed to the specific targeting of E-selectin and the synergistic effects of ibuprofen conjugation.
We believe that the discovery of these new signaling pathways or targets has important guiding significance for the development of future polymer-related therapies. However, how to link these targets or signals with polymers-targeted therapies is still a key problem in translational medicine research. In this case, seeking a more accurate polymers-based targeted delivery system is particularly important and, for the major biopolymers in Figure 4, has great development prospects.
Figure 4. The main classifications of nanopolymers are divided into two categories based on their organic and inorganic properties; inorganic NPs are mainly named based on their compounds, such as silicon NPs, iodine oxides NPs, and so on, while organic NPs can be divided into many categories. In this article, organic NPs are classified into two categories: Polymeric and Lipid-based. Polymeric mainly includes Polymersome, Dendrimer, Micelle, and Nanoball. For Lipid-based, they mainly include liposomes, lipids, nanoparticles, emulsions, etc.
LIPID-BASED NPS IN RENAL CELL CARCINOMA
Introduction of lipid-based NPs
Though lipid-based NPs encompass various component configurations, lipid-based NPs are spherical platforms comprising at least one lipid bilayer surrounding at least one internal aqueous compartment[24]. Lipid-based NPs have many benefits as drug delivery vehicles, including formulation simplicity, self-assembly, biocompatibility, high bioavailability, ability to carry large payloads, and various physicochemical characteristics that can be controlled to vary their biological features[25]. Figure 5 demonstrates surface modifications of lipid-based NPs, as well as internal and external stimulatory factors.
Figure 5. Lipid-based NPs have flexible surface modification options, including cell coating, Vitamin, etc.; they can also change the surface toughness to suit different drug delivery needs for lipid-based. Meanwhile, lipid-based NPs can receive intrinsic or extrinsic stimuli to produce a response (usually rupture), with intrinsic motivations including various enzyme levels, metabolic changes, and other precision regulation, and extrinsic stimuli including physical stimuli such as sound, light, and electricity.
Lipid-based NPs in gene therapy
The primary characteristic of liposomal polymeric materials is the presence of bipolar molecules that are both hydrophilic and lipophilic. These molecules can self-assemble into spherical polymer nanoparticles, encapsulating medicines, biologically active components like RNA and DNA, and metal particles. Additionally, to act as specific medication carriers, their surfaces can be altered with pertinent antibodies, vitamins, and other guiding molecules[26]. Retroviruses, adenoviruses, and lentiviruses are among the viral vectors employed in gene therapy as delivery systems[27]. However, due to safety issues such as carcinogenic potentials, immunological detection of the viral capsid proteins, and induction of diverse immune responses in vivo, viral vectors have limited their clinical applicability[28].
Fortunately, non-viral carriers are increasingly widely utilized for secure and effective delivery of nucleic acids, such as a prominent subset of lipid-based NPs known as lipid nanoparticles (LNPs) - liposome-like structures. LNPs typically consist of four main parts: cationic or ionizable lipids, phospholipids for particle structure, cholesterol for stability and membrane fusion, and PEGylated lipids to enhance stability and circulation. These lipids interact with genetic material that has a negative charge to aid in the escape of endosomes[29,30]. Ionizable LNPs, which have nearly neutral charges at physiological pH but become set in acidic endosomal compartments, are excellent for intracellularly delivering nucleic acid therapy. LNPs are particularly crucial in implementations related to tailored genetic therapy due to their nucleic acid delivery effectiveness and their straightforward manufacturing, diminutive size, and serum stability.
The condensation of plasmid DNA with positively charged substances (polycations or liposomes) has been a prevalent strategy ever since the initial documentation of effective plasmid DNA (pDNA) transfection using a positively charged lipoplex[31]. This approach is founded on the premise that the positively charged attribute plays a pivotal role in cellular interaction, facilitated by the negatively charged heparan sulfate present on the cell membrane surface[32]. Akita et al. developed a plasmid DNA (pDNA)-encapsulating liposomal nanoparticle (LNP) as a gene vector platform for targeting RCC[33]. The critical component is a SS-cleavable and pH-activated lipid-like material (ssPalm) that mounts dual sensing motifs (tertiary amines and disulfide bonds) to respond to various intracellular environments and surface-modified with polyethylene glycol to enhance its blood circulation stability. The study also highlighted the use of vitamin E as a hydrophobic scaffold, which showed synergistic anti-tumor function with encoded pDNA. According to reports, LNPssPalmE had a much more significant anti-tumor effect than LNPssPalmM while only slightly outperforming LNPssPalmM in transfection activity. Hama et al. demonstrated that the apoptotic activity against cancer cells of α-tocopherol succinate, derived from the degradation of ssPalmE, is noteworthy[34]. Additionally, tumor growth inhibition was pronounced upon administration of completely CpG-free pDNA encoding the soluble form of VEGFR (fms-like tyrosine kinase-1: sFlt-1), especially when delivered through lipid nanoparticle (LNP) formulations containing ssPalmE (LNPssPalmE). Hence, the PEG-modified LNP ssPalmE emerges as a promising vehicle for gene therapy directed at RCC.
DNA immunization presents another auspicious avenue for tumor immunotherapy. Following intramuscular injection, plasmid DNA can prompt the expression of the encoded antigen within the human system, thus eliciting both cellular and humoral immune responses. He et al. selected Nesprin-2 L4492R (Nes2LR) from 20 novel epitopes and packaged it in immunogenic liposomes[35]. In a murine model of RCC, their findings indicated that Nes2LR vaccination combined with immune checkpoint blockade effectively mitigated or reversed the disease progression across subcutaneous, experimental lung metastasis, and orthotopic tumor models. Additionally, the Nes2LR neoepitope holds promise for preclinical investigations pertaining to immunotherapeutic interventions against RCC.
Lipid-based NPs in targeted drug delivery
Liposomes are a distinct subset of lipid-based nanoparticles with the most members. They are commonly made up of phospholipids, which can form unilamellar and multilamellar vesicular structures. Therefore, liposomes can transport and encapsulate hydrophilic, hydrophobic, and lipophilic medications[36]. However, liposome carriers confront challenges including multiple defense systems of the human body, especially reticulo-endothelial system (RES) leading to shortened liposome circulation time and tumor accumulation[37] and low drug-loading capacity[25]. Improving the effectiveness of liposomes in drug delivery is a major focus of research in this field.
A hydrophobically modified chitosan (HMC) coat can increase particle stability and overall circulation time. Additionally, it enhances tumor cell uptake due to the interaction force of differently charged surfaces of the HMC shell and tumor cell[38]. Liu et al. conducted a comparative analysis of various biocompatible nanoparticle formulations incorporating Sorafenib, a targeted agent for RCC[39]. The nanoparticle models under scrutiny included poly (lactic & glycolic) acid, liposomes, and HMC-coated liposomes. Flow cytometry data comparing DiI-Lip and flu-HMC DiI-Lip treatments showed that cells treated with flu-HMC DiI-Lip exhibited higher DiI intensity than those treated with DiI-Lip alone or unstained cells, indicating that additional HMC coating improved the delivery of the RCC drug Sorafenib by liposomes.
On the other hand, combining therapies could be a valuable approach to address the challenge of low liposomal drug-loading capacity. Pal et al. employed phospholipids, cholesterol, DSPE-(PEG)2000-Ome, and a proprietary tumor-targeting peptide (TTP)-conjugated lipopeptide to encapsulate two distinct medications, everolimus and vinorelbine, within a tumor-directed liposomal formulation[8]. Figure 6 illustrates the process of preparation of this tumor-directed liposome formulation. The combination therapy comprising everolimus and vinorelbine successfully inhibited in vitro proliferation and in vivo tumor growth and lung metastasis. In the single mouse trial, eight-week-old male SCID mice were subcutaneously injected with 5 × 106 786-O cells on their right flanks. The tumors could grow until they reached an average size of ~400-500 mm3. Afterward, drug-loaded liposomes were administered to the mice (one mouse per treatment group) thrice a week for three weeks. Tumor dimensions were assessed on a weekly basis, and their respective growth curves were plotted accordingly. In both experimental settings, it was noted that liposomes loaded with two drugs exhibited noteworthy inhibition, surpassing the efficacy observed with liposomes carrying only a single drug, a trend consistent with the outcomes observed in A498 xenografts. Notably, this tumor suppression was accomplished at reduced dosages of the drugs (everolimus at
Figure 6. Illustration depicting the synthesis process of drug-incorporated liposomes[8]. DOPC: 1,2-dioleoyl-sn-glycero-3-phosphocholine; TTP: tumor targeting peptide; DSPE-PEG2000-Ome: 1,2-distearoyl-sn-glycero-3-phosphoethanolamine-N-[methoxy(polyethylene glycol)-2000] (ammonium salt).
In addition, doxorubicin, one of the important chemotherapeutic agents for RCC, was packaged as a model cargo following the process shown in Figure 7 in a MITO-Porter to make DOX-MITO-Porter by
Figure 7. Schematic diagram showing the preparation of the DOX-MITO-Porter using a pH loading method[40]. DOX: Doxorubicin; IB: internal buffer; EB: external buffer; ASS: assay buffer.
Liposome-loaded drug delivery and antigen-antibody targeted conjugation for enhanced ultrasound imaging of RCC xenografts for differentiation of non-cancerous and cancerous lesions in renal masses. The G250 antigen represents a transmembrane protein abundantly expressed in the majority of RCCs while remaining absent in healthy renal tissue[20]. Yu et al. formulated targeted nanobubbles (anti-G250 NTNs) functionalized with anti-G250 nanobodies by coupling them with lipid nanobubbles[41]. Their study validated the targeting specificity and binding efficacy of these nanobubbles to RCC cells expressing the G250 antigen, as well as their ability to enhance ultrasound imaging of RCC xenografts[41]. In vitro experiments demonstrated the specific attachment of anti-G250 NTNs to G250-positive 786-O and HeLa cells, while no binding was observed with G250-negative ACHN cells. In vivo investigations exhibited a significant improvement in ultrasound imaging of xenograft tumors originating from 786-O and HeLa cells following the administration of anti-G250 NTNs compared to control nanobubbles. However, there was no discernible enhancement in tumors derived from ACHN cells. Immunofluorescence imaging of the tumor tissue sections confirmed the infiltration of anti-G250 nanobodies into tissues through the tumor blood vessels, where they selectively adhered to tumor cells. Overall, the use of anti-G250 nanobodies holds excellent promise as a solution to distinguishing between benign and malignant changes in kidney masses, as they specifically bind to G250-positive RCC cells and enhance the ultrasound imaging of G250-positive masses.
As a means of drug transportation, lipid-based nanoparticles (NPs) present numerous benefits, such as straightforward formulation, self-assembly, biocompatibility, elevated bioavailability, extensive cargo capacity, and a diverse array of physicochemical attributes that can be modulated to modify their biological properties. Lipid-based NPs are one of the most widely used and recognized delivery systems in targeted therapy or gene therapy. However, as a delivery system, lipid-based NPs also have some unavoidable shortcomings due to their characteristics, such as inaccurate load, difficulties in ensuring the uniform physical size of the delivery body, and difficulty in implementing multiple target modifications for various targets.
INORGANIC NPS IN RENAL CELL CARCINOMA
Introduction of inorganic polymer NPs
Inorganic polymer nanoparticles have been used not only as contrast agents in computed tomography (CT) and magnetic resonance imaging (MRI) in clinical practice, but also as probes in laboratory tests and pathological examinations to detect tumor biomarkers due to their improved electrical conductivity, biophilicity, photothermal effect, X-ray blocking effect, and magnetic resonance effect. Inorganic polymer nanoparticles have also been used to help surgeons define the tumor site[42].
Inorganic materials such as gold, iron, and silica have been utilized in the fabrication of nanostructured materials for various applications, including drug delivery and imaging. Inorganic nanoparticles precisely manipulate their size, structure, and geometry. Primarily, inorganic NPs possess distinctive physical, electrical, magnetic, and optical characteristics attributed to the material’s inherent properties. For instance, gold nanoparticles have unbound electrons on their surface that constantly vibrate at a frequency that relies on their size and shape, conferring upon them photothermal characteristics[43].
Inorganic NPs in surface-assisted laser desorption ionization method
MALDI MSI has been successfully employed to analyze the spatial distribution of proteins and lipids within the kidney tissue of patients with RCC[44,45]. However, the use of low molecular weight organic acids as matrices by conventional methods is limited due to the generation of numerous high background peaks in the low mass range (up to m/z 800), low mass determination accuracy due to the large thickness of the sample and the sweet spot effect. Due to various limitations and subjective evaluations, MALDI is not the optimal technique for identifying, analyzing, and imaging low-molecular-weight compounds, including cellular metabolites. This method offers a promising alternative for low-molecular-weight biomarker research. To address these constraints, a novel matrix-free laser desorption-ionization approach has been introduced based on the principle basis of the MALDI assay in Figure 8, which utilizes a steel platform coated with positively charged silver or gold nanoparticles (AgNPs/AuNPs)[6,46]. The latter represents a surface-assisted laser desorption ionization method (SALDI) that, unlike MALDI, is matrix-free.
Figure 8. The MALDI technique is based on a series of matrix substances surrounding the particles to be detected, after which the laser irradiates the detection plane, and the matrix-wrapped particles to be seen are desorbed from the overall material. Then, the particles to be seen and the surrounding matrix particles undergo desolvation and ionization, whereby the matrix substances transfer positive charges to the particles to be caught to form a Particle for Mass Spectrometry.
Inorganic NPs in vitro detection
Regarding diagnosis methods, renal mass biopsy may have some benefits but is at risk of sampling error, invasiveness, and morbidity. Consequently, it is crucial to explore alternative, non-intrusive techniques, such as those employing unique chemical compounds found in body fluids that could reveal the presence of a tumor. Regrettably, significant efforts have been invested over the past few decades to pinpoint microscopic molecule markers peculiar to RCC. However, no reliable markers guide more effective therapies, diagnosis, or tumoral prognosis. Over the past few decades, there has been a significant increase in the use of metabolomics applications in cancer research. Urine has become a preferred biospecimen source for the examination of kidney cancer using metabolomics because of its strong association with the disease’s origin. Metabolic profiling was conducted on urine samples collected from fifty patients diagnosed with kidney cancer and fifty healthy people. This profiling employed high-resolution proton nuclear magnetic resonance spectroscopy and silver-109 nanoparticle-enhanced steel target laser desorption/ionization mass spectrometry[47]. Four metabolites with significantly altered levels in both cancer patients and non-cancer controls were putatively identified using 109AgNPET LDI MS metabolite profiling. The study has revealed the capacity of mass spectrometry to detect informative urinary biomarkers for RCC. In conclusion, monitoring alterations in urinary metabolite concentrations could emerge as a valuable approach for screening and monitoring the progression of RCC in a less invasive manner.
Inorganic NPs in photodynamic therapy and photothermal therapy
Photodynamic therapy (PDT) and photothermal therapy (PTT) are effective means of cancer treatment. Ever since the discovery of the photocatalytic properties of titanium dioxide in 1972, TiO2-based materials have garnered extensive research attention as photosensitizers in photodynamic therapy[48]. Under irradiation, TiO2 can generate ROS by reacting with water to cause cell damage. Yang et al. developed a core-shell structured formulation consisting of titanium dioxide@red phosphorus nanorods
Figure 9. Illustration depicting the synthesis process of the TiO2@RP heterostructure[49]. TiO2: Titanium dioxide; RP: red phosphorus.
Above all, numerous studies have investigated the potential applications of nanoparticles in cancer management, mainly when employed as drug delivery systems. These investigations have yielded significant findings indicating that nanoparticles can offer reduced toxicity and improved pharmacokinetics over traditional cancer therapies. Additionally, due to their adjustable structures that provide the possibility of encapsulating the drug or conjugating it on the surface and their unique sizes, they can effectively target cancer cells and deliver therapeutic payloads with high precision and efficacy. In recent years, significant developments in the clinical application of nanoparticles, including biomimetic nanocarriers and metal-organic frameworks, have emerged to tackle existing challenges. Notably, given the ever-increasing number of different formulations and the vast amount of research on nanomaterials, the number of candidates reaching clinical trials or commercialization is minimal[50]. Thus, further research is required to fully understand the clinical potential of nanoparticle-based cancer therapies.
MEGA-POLYMERS IN RENAL CELL CARCINOMA-RELATED CLINIC STUDIES
While the application of biopolymers in RCC has focused on nanomaterials, we will present some noteworthy studies involving the clinical applications of mega-polymers. As their natural analogs, the favorable biological, physical, and chemical properties of mega-polymers have led to their use in many fields, including tissue engineering materials, and therapeutic and diagnostic applications. In vitro,
RCC has a biological predisposition to direct vascular invasion, with 4% to 10% involving the venous system, of which 22% to 70% involve the inferior vena cava (IVC). The median life expectancy of patients with untreated RCC with inferior vena cava thrombosis is 5 months, with a 1-year disease-specific survival rate of 29%[52]. The applications of mega-polymers provide worthwhile new ideas and methods for this situation. Using a circular polytetrafluoroethylene (PTFE) cannula, Ciancio et al. graft-reconstructed the inferior vena cava of a patient with RCC with tumor thrombus (TT) invasion of the IVC, with simultaneous placement of an IVC filter[53]. The novelty of this approach is that an IVC filter can be deployed before completing the distal IVC anastomosis. During the postoperative follow-up period, thrombosis did not occur in any of the patients’ IVC grafts and the PTFE graft in each patient was patent at last follow-up. This implies that PTFE, a synthetic vascular substitute made of polymer, offers a positive clinical outlook and serves as a safe and viable vascular channel in RCC’s treatment.
DISCUSSION
Currently, renal cell carcinoma faces challenges such as late diagnosis and poor prognosis, particularly in patients with metastatic RCC. Surgical procedures like direct resection, radiofrequency ablation, and interventional embolization are common treatments for localized RCC. However, treatment decisions must consider individual patient health and the balance between postoperative benefits and risks. Since sorafenib’s approval for metastatic RCC treatment in 2005, targeted therapy has revolutionized treatment. Nevertheless, dose-dependent side effects remain a concern. Moreover, increasing early screening and diagnosis rates for kidney cancer is essential to reduce the burden of advanced stages. Significant progress has been made in developing biopolymers, especially nanomedicines, offering potential solutions to these challenges in clinical diagnosis and treatment.
In clinical diagnosis, Table 1 summarizes some of the uses of biopolymers for laboratory liquid tests, pathology, and imaging tests. Notably, they hold vast potential in liquid biopsy, as demonstrated in ongoing collaborative studies. These studies explore using single-molecule biomarkers to nucleate and assemble large-scale polymers, enhancing detection signals. Biopolymers’ characteristics enable early screening of tumors like RCC with subtle symptoms. Further research aims to unlock the full potential of this method. Additionally, biopolymers can enhance the accuracy and visibility of pathological and imaging detection by serving as delivery carriers.
Polymers for clinical diagnosis
Diagnostic type | Target substance | Diagnostic agent | Experiment type | Dominance |
Laboratory examination of bodily fluids | Circulating tumor cells | CELLection™ Dynabeads®[54] | In vitro | Circulating tumor cells in metastatic RCC |
Laboratory examination of bodily fluids | Soluble biomarker | Borosilicate micropipettes and complementary γ-peptide nucleic acid (γ-PNA) probes linked to polystyrene beads[55] | In vitro | Safely detect miR-204 and miR-210 associated with ccRCC |
Laboratory examination of bodily fluids | Soluble biomarker | Gold nanoparticles-assisted laser desorption/ionization mass spectrometry[56] | In vitro | Compared the LOD and the LMW of different analytical methods |
Laboratory examination of bodily fluids | Soluble biomarker | Silver-109 nanoparticle enhanced steel target laser desorption/ ionization mass spectrometry[57] | In vitro | Prospects for clinical diagnosis/differential diagnosis |
Laboratory examination of bodily fluids | Circulating tumor cells | PPy-Ag@BSA[58] | Human study | Efficient capture of circulating tumor cells in RCC |
Pathology | Circulating tumor cells | Shell-isolated nanoparticle- -enhanced Raman spectroscopy in microfluidic device[59] | In vitro | High diagnostic accuracy of circulating tumor cells |
Pathology | Patient tissue | AgNPET/Laser desorption/ ionization MS imaging[60] | In vitro | Facilitated swift visualization of the disparities between ccRcc and the healthy segment of kidney tissues |
Pathology | Patient tissue | Biotin-streptavidin binding and fluorescence active magnetic nanocarriers[61] | Human study | The detection of trace miRNA 15a |
Pathology | Patient tissue | Peptide-coated gold clusters exhibiting inherent red fluorescence and a distinct mass signal[62] | In vitro & vivo & human study | Prognosis evaluation |
Pathology | patient tissue | EVs identified through nanoparticle tracking analysis[63] | In vitro & human study | The detection of CA9/CD70/CD147 |
Imaging | Tumor cells | mAb G250-SPIO molecular nanoprobe[64] | In vitro | Contrast agent in specific tumor cells |
Imaging | Lymph node | Lymphotropic nanoparticle- enhanced magnetic resonance imaging-monocrystalline iron oxide nanoparticle[65] (ferumoxtran-10 Combidex],AMAG harmaceuticals, Inc.) | Human study | Contrast agent for lymph node |
Imaging | Lymph node | 99mTc-nanocolloid[66] | Human study | Mapping of sentinel lymph nodes |
Imaging | Whole tumor | Fe3O4 @mSiO2 /PDDA/ BSA-Gd2O3 nanocomplex[67] | Human study | Being a T1-T2 dual-mode contrast agent |
Imaging | Tumor cells | AS1411 Aptamer Modified Mn-MoS2 QDs[7] | In vitro & in vivo | Fluorescently label RCC cells and enhance MRI signal |
Imaging | Lymph node | Hydrophilic manganese oxide nanoparticles modified AS1411 aptamer[67] | In vitro & in vivo | High T1 relaxivity |
Imaging | Tumor cells | Anti-G250 nanobody- functionalized targeted nanobubbles for ultrasound[41] | In vitro & in vivo | Specific contrast agent for G250(+) RCC cells |
Imaging | Whole tumor & tumor cells | Targeted nanobubbles carrying CAIX polypeptides/aptamer[68] | In vitro & in vivo | Specific contrast agent in CAIX(+) tumor tissues |
Nanomedicine carriers, with their excellent physical and chemical properties, offer promise in improving the efficacy of chemotherapeutic drugs. Targeted nanoparticles, for instance, can precisely deliver drugs to tumor cores, minimizing toxicity to normal tissues. Table 2 demonstrates the in vivo and in vitro applications of some of the polymers. However, transitioning these advancements to the clinical stage presents challenges. Current tumor animal models may not accurately reflect drug pharmacokinetics and the tumor microenvironment’s complexity, hindering in-depth investigations into drug release control[82].
Polymers research for therapy in vitro and vivo
Category | Polymers | Therapeutic strategies | Dominance | Experiment type | |
Nanodrugs | Lipid-based NPs | DOX-MITO-Porter[40] | Chemotherapy | Suppress mitochondria functions | In vitro |
Tumor-targeted liposomal formulation[8] | Chemotherapy | Dual drug-loaded and targeting ligand-decorated liposome | In vitro & vivo (subcutaneous) | ||
Hydrophobically modified chitosan-coated liposomes[39] | Chemotherapy | HMC coat promotes more interaction between cell membrane and particle | In vitro | ||
Liposomes[35] | Tumor vaccine | Enhancement of anti-tumor immunity | In vitro & vivo | ||
LNPssPalmE[33] | Gene therapy | Collaborative action of gene products encoded in plasmid DNA (pDNA) and LNPssPalmE | In vitro & vivo (subcutaneous) | ||
Polymeric NPs | Chlorambucil-conjugated PI polyamide[69] | Chemotherapy | Induce proliferation arrest depending on p21 expression | In vitro | |
CA IX-SMA-TPGS (combined with Sor)[21] | Chemotherapy | Target hypoxia core using CA IX (tumor core penetration) | In vitro & vivo | ||
Sialic acid-poly-ibuprofen[23] | Chemotherapy | Synergistic anti-tumor effect of ibuprofen due to COX-2 reduction | In vitro & vivo (subcutaneous) | ||
Poly acid[39] | Chemotherapy | A highly effective drug transport system facilitating gradual and uniform drug release while suspended | In vitro | ||
Poly acid[70] | Chemotherapy | Notable preclinical antimetastatic impacts on lung metastatic cells of RCC | In vivo (orthotopic) | ||
PH1/pHGFK1[71] | Gene therapy | HGFK1 could counteract sorafenib-induced stemness of RCC, thus alleviating Sor resistance | In vitro & vivo | ||
CH-CA-Spe nanogel[72] | Gene therapy | Enable successful transfection of siRNA | In vitro & vivo (subcutaneous) | ||
PLGA-HB-NPs[22] | Adjuvant therapy | Marginally attenuate the resistance of RCC cells to oxaliplatin under hypoxic conditions | In vitro | ||
Sunitinib-loaded micellar nanocomplex[73] | Chemotherapy | Combined anticancer efficacy of SU and the carrier containing PEG-EGCG exhibited synergistic effects | In vitro & vivo (subcutaneous) | ||
Magnetic-core-based silibinin nanopolymeric carriers[74] | Chemotherapy | show a greater inhibitory effect compared to free SLB | In vitro & vivo | ||
CRLX101[75] | Chemotherapy | No additive toxicity of the combination was observed | Clinical trial | ||
Purified glycogen Polycationic derivatives[76] | Gene therapy | Gene carrier | In vitro & vivo | ||
P1-DBCO combined doxorubic[77,78] | Chemotherapy | Antitumor and metastasis | In vitro & vivo | ||
Polyion complex-loaded miRNA-143#12[79] | Gene therapy | Inhibition KRAS | In vitro & vivo | ||
H1/pAIM2[80] | Gene therapy | Enhance the inflammasome pathway to suppress malignancies associated with RCC | In vitro & vivo (subcutaneous) | ||
Inorganic NPs | MIL-101(Fe) NPs[81] | Chemotherapy | Apart from supplying iron ions to cancer cells, MIL-101(Fe) nanoparticles can function as a delivery platform for RSL3 | In vitro & vivo (subcutaneous) | |
Megapolymers | Chitosan oligosaccharide[82] | Chemotherapy | Repress human renal carcinoma growth and induce apoptosis via ROS-dependent ER stress pathways | In vitro & vivo (orthotopic) | |
Pegilodecakin[83] | Chemotherapy | Induces intratumoral antigen-specific CD8(+) T cells and upregulates IFN γ and MHC I & II | Clinical trial | ||
Interventional and surgical agents | Polymeric NPs | tLyP-1/PR-619/Fe3O4@PCM (tPF@PCM)[84] | Photothermal therapy | Induce ERS and cause apoptosis | In vitro & vivo |
Gold nanorod encapsulated albumin[85] | Photothermal therapy | Synergistic tumor necrosis | In vitro & vivo | ||
Inorganic NPs | 99mTc-nanocolloid[66,86] | Image-guided surgery | Localization of sentinel lymph nodes | Human body (clinical trial) | |
X-ray irradiation and quantum dots composed of black phosphorus[87] | Radiotherapy | Enhance apoptotic of tumor cells | In vitro & vivo | ||
TiO2@red phosphorus nanorods (TiO2@RP NRs)[49] | Photothermal therapy | Antitumor thermal effect | In vitro & vivo | ||
Mesoporous silica nanoparticles[88] | Photothermal therapy | Enhance antiproliferative effect | In vitro & vivo | ||
Gold nanorods[85] | Photothermal therapy | Simultaneous observation of cell death as a photothermal therapy drug | In vitro |
Recent research by Yamamoto et al. highlights the importance of considering the tumor microenvironment in nanoparticle delivery. They found that inhibiting VEGFR2 enhances macrophage infiltration, improving nanoparticle delivery in highly vascularized RCC[89]. This underscores the necessity of targeting both cancer cells and the tumor microenvironment in treatment strategies. Additionally, the role of extracellular vesicles in cancer treatment is significant, influencing various aspects like angiogenesis and metastasis[90]. Extracellular vesicles are small vesicles found in the extracellular space, consisting of a lipid bilayer membrane structure. They are actively excreted by both normal cells and cancer cells, containing proteins, nucleic acids, lipids, and various other bioactive compounds. These proteins play a crucial role in cell-to-cell communication by liberating bioactive compounds that either merge with receptor cell membranes or adhere to receptors on the cell surface, facilitating intercellular information exchange[91-94].
Patient-derived xenograft (PDX) models offer a promising approach to address challenges in cancer research. The PDX model is established by transplanting fresh tumor tissue, cut from human cancers, into mice[95]. In contrast, it more accurately reflects cancer characteristics and can simulate the progression and evolution of tumors in human patients. PDX models have produced the most compelling preclinical results and are regarded as one of the most promising models for addressing challenges faced by clinicians. Consequently, PDX models, with their aforementioned advantages, have gained increasing attention and development in recent years. We are actively developing a PDX model system for kidney cancer, aiming to further validate key aspects such as the efficiency and safety of biopolymer drug delivery, which is the focal point of our research efforts.
Several nanomedicines have been approved for clinical use in tumoral treatment, but their indications need expansion. Some novel nanomedicines have entered clinical trials, demonstrating feasibility and effectiveness in clinical settings[75,96-98]. However, they also present a typical drawback compared to traditional drugs: low drug loading, resulting in poor efficacy, necessitating increased doses and/or dosing frequencies, thereby increasing systemic toxicity[73]. To prolong drug circulation time and accumulation in tumors or reduce drug doses, various strategies have been proposed, including tumor-targeting ligands[21], surface modification[39], dual-drug delivery[8], and constructing drug carriers with synergistic therapeutic effects[23,73]. To the best of our knowledge, there is currently no FDA-approved liposome formulation utilizing active targeting ligands[8]. This suggests that translating these methods from theory into clinical practice will require extensive clinical trials and exploration of new carrier development.
The tremendous potential demonstrated by biopolymers in various fields fuels our anticipation for their further development and application. Meanwhile, in the face of the aforementioned challenges, we aim to connect a plethora of studies exploring significant new biomarkers and mechanistic pathways of RCC. Recent research has identified CD133+/CD24+ cell subsets in RCC specimens with self-renewal ability and clonal multipotentiality[99]. Previously, we reported the phenomenon of “slimming”[100] in RCC cells, characterized by tumor cell volume reduction and lipid titration. Through related work, the mechanisms of action of HIF2a and its downstream regulatory molecule NUDT1 have been explored[101,102]. The potential of biopolymers in targeting these signaling pathways offers a new approach to RCC treatment beyond traditional therapies. Additionally, advancements such as DNA Origami provide a fresh perspective for biopolymer delivery systems. These are areas that require our focused attention and efforts in the future.
CONCLUSION
In this review, we focus on the advanced progress of applying biopolymers in the treatment of RCC, particularly in material classification and clinical pathways. With the rapid development of biomedical engineering, research into the adjunctive use of biopolymers alongside current frontline clinical therapies and diagnostics offers new hope. Although the clinical application may still be at a distance, the future intersection of medical science with biology and chemistry will become increasingly important. We believe that by leveraging the design and biophysical and chemical properties of biopolymers, in conjunction with extensive foundational work on classical targets and pathway signaling molecules in RCC, as well as the construction of PDX models, we can accelerate the clinical translation of biopolymers for RCC, thereby achieving effective diagnosis and treatment for RCC patients as soon as possible.
DECLARATIONS
Authors’ contributions
Made substantial contributions to the design and completion of the study and performed the illustrative drawings: Zhou X, Xue K, Xu R
Performed literature acquisition and data analysis: Huang F, Li Q, Huang Y
Performed academic guidance: Xiao W, Wang K, Zhang X
Availability of data and materials
Not applicable.
Financial support and sponsorship
This study was supported by the National Natural Scientific Foundation of China (Grant No. 82303033, 82000512), Hubei Province Natural Scientific Foundation (2023AFB416), Shenzhen Medical Research Fund (Grant no. B2302054).
Conflicts of interest
Xiao W is an Editorial Board member of the journal Journal of Cancer Metastasis and Treatment. while the other authors have declared that they have no conflicts of interest.
Ethical approval and consent to participate
Not applicable.
Consent for publication
Not applicable.
Copyright
© The Author(s) 2024.
REFERENCES
1. Siegel RL, Miller KD, Wagle NS, Jemal A. Cancer statistics, 2023. CA Cancer J Clin 2023;73:17-48.
2. Rassy E, Parent P, Lefort F, Boussios S, Baciarello G, Pavlidis N. New rising entities in cancer of unknown primary: is there a real therapeutic benefit? Crit Rev Oncol Hematol 2020;147:102882.
3. Ronchi E, Pizzocaro G, Miodini P, Piva L, Salvioni R, Di Fronzo G. Steroid hormone receptors in normal and malignant human renal tissue: relationship with progestin therapy. J Steroid Biochem 1984;21:329-35.
4. Zheng R, Zhang S, Zeng H, et al. Cancer incidence and mortality in China, 2016. J Nat Cancer Center 2022;2:1-9.
5. Kramer PA. Letter: albumin microspheres as vehicles for achieving specificity in drug delivery. J Pharm Sci 1974;63:1646-7.
6. Nizioł J, Ossoliński K, Ossoliński T, et al. Surface-transfer mass spectrometry imaging of renal tissue on gold nanoparticle enhanced target. Anal Chem 2016;88:7365-71.
7. Zheng S, Zhang M, Bai H, et al. Preparation of AS1411 aptamer modified Mn-MoS2 QDs for targeted MR imaging and fluorescence labelling of renal cell carcinoma. Int J Nanomed 2019;14:9513-24.
8. Pal K, Madamsetty VS, Dutta SK, Mukhopadhyay D. Co-delivery of everolimus and vinorelbine via a tumor-targeted liposomal formulation inhibits tumor growth and metastasis in RCC. Int J Nanomed 2019;14:5109-23.
9. Wu R, Wang K, Gai Y, et al. Nanomedicine for renal cell carcinoma: imaging, treatment and beyond. J Nanobiotechnol 2023;21:3.
10. Pontes O, Oliveira-Pinto S, Baltazar F, Costa M. Renal cell carcinoma therapy: current and new drug candidates. Drug Discov Today 2022;27:304-14.
11. Chiu IJ, Hsu YH, Chang JS, Yang JC, Chiu HW, Lin YF. Lactotransferrin downregulation drives the metastatic progression in clear cell renal cell carcinoma. Cancers 2020;12:847.
12. Zheng JQ, Lin CH, Lee HH, et al. Lactotransferrin downregulation serves as a potential predictor for the therapeutic effectiveness of mTOR inhibitors in the metastatic clear cell renal cell carcinoma without PTEN mutation. Biomedicines 2021;9:1896.
13. Caldorera-Moore M, Vela Ramirez JE, Peppas NA. Transport and delivery of interferon-α through epithelial tight junctions via pH-responsive poly(methacrylic acid-grafted-ethylene glycol) nanoparticles. J Drug Target 2019;27:582-9.
14. Strand MS, Krasnick BA, Pan H, et al. Precision delivery of RAS-inhibiting siRNA to KRAS driven cancer via peptide-based nanoparticles. Oncotarget 2019;10:4761-75.
15. Liu X, Li C, Lv J, et al. Glucose and H2O2 Dual-responsive polymeric micelles for the self-regulated release of insulin. ACS Appl Bio Mater 2020;3:1598-606.
16. Brown JE, Royle KL, Gregory W, et al. Temporary treatment cessation versus continuation of first-line tyrosine kinase inhibitor in patients with advanced clear cell renal cell carcinoma (STAR): an open-label, non-inferiority, randomised, controlled, phase 2/3 trial. Lancet Oncol 2023;24:213-27.
17. Hillen F, Griffioen AW. Tumour vascularization: sprouting angiogenesis and beyond. Cancer Metastasis Rev 2007;26:489-502.
18. Mcdonald PC, Dedhar S. Carbonic anhydrase IX (CAIX) as a mediator of hypoxia-induced stress response in cancer cells. Subcell Biochem 2014;75:255-69.
19. Krall N, Pretto F, Mattarella M, Müller C, Neri D. A 99mTc-labeled ligand of carbonic anhydrase IX selectively targets renal cell carcinoma in vivo. J Nucl Med 2016;57:943-9.
20. Liao SY, Aurelio ON, Jan K, Zavada J, Stanbridge EJ. Identification of the MN/CA9 protein as a reliable diagnostic biomarker of clear cell carcinoma of the kidney. Cancer Res 1997;57:2827-31.
21. Alsaab HO, Sau S, Alzhrani RM, et al. Tumor hypoxia directed multimodal nanotherapy for overcoming drug resistance in renal cell carcinoma and reprogramming macrophages. Biomaterials 2018;183:280-94.
22. Chen L, Wang Z, Xu Q, et al. The failure of DAC to induce OCT2 expression and its remission by hemoglobin-based nanocarriers under hypoxia in renal cell carcinoma. Theranostics 2020;10:3562-78.
23. Zeng X, Teng Y, Zhu C, et al. Combined ibuprofen-nanoconjugate micelles with E-selectin for effective sunitinib anticancer therapy. Int J Nanomed 2022;17:6031-46.
24. Mitchell MJ, Billingsley MM, Haley RM, Wechsler ME, Peppas NA, Langer R. Engineering precision nanoparticles for drug delivery. Nat Rev Drug Discov 2021;20:101-24.
25. Sercombe L, Veerati T, Moheimani F, Wu SY, Sood AK, Hua S. Advances and challenges of liposome assisted drug delivery. Front Pharmacol 2015;6:286.
26. Heng DY. The next 10 years: challenges for the future and overcoming resistance to targeted therapies for renal cell carcinoma. Can Urol Assoc J 2016;10:S256-8.
27. Chen J, Gao P, Yuan S, et al. Oncolytic adenovirus complexes coated with lipids and calcium phosphate for cancer gene therapy. ACS Nano 2016;10:11548-60.
28. Santiago-Ortiz JL, Schaffer DV. Adeno-associated virus (AAV) vectors in cancer gene therapy. J Control Release 2016;240:287-301.
29. Kulkarni JA, Witzigmann D, Leung J, Tam YYC, Cullis PR. On the role of helper lipids in lipid nanoparticle formulations of siRNA. Nanoscale 2019;11:21733-9.
30. Cheng X, Lee RJ. The role of helper lipids in lipid nanoparticles (LNPs) designed for oligonucleotide delivery. Adv Drug Deliv Rev 2016;99:129-37.
31. Felgner PL, Gadek TR, Holm M, et al. Lipofection: a highly efficient, lipid-mediated DNA-transfection procedure. Proc Natl Acad Sci USA 1987;84:7413-7.
32. Mislick KA, Baldeschwieler JD. Evidence for the role of proteoglycans in cation-mediated gene transfer. Proc Natl Acad Sci USA 1996;93:12349-54.
33. Akita H, Ishiba R, Togashi R, et al. A neutral lipid envelope-type nanoparticle composed of a pH-activated and vitamin E-scaffold lipid-like material as a platform for a gene carrier targeting renal cell carcinoma. J Control Release 2015;200:97-105.
34. Hama S, Kogure K. Nanoparticles consisting of tocopheryl succinate are a novel drug-delivery system with multifaceted antitumor activity. Biol Pharm Bull 2014;37:196-200.
35. He X, Zhou S, Dolan M, et al. Immunization with short peptide particles reveals a functional CD8+ T-cell neoepitope in a murine renal carcinoma model. J Immunother Cancer 2021;9:e003101.
36. Sarfraz M, Afzal A, Yang T, et al. Development of dual drug loaded nanosized liposomal formulation by A reengineered ethanolic injection method and its pre-clinical pharmacokinetic studies. Pharmaceutics 2018;10:151.
37. Poste G, Papahadjopoulos D, Vail WJ. Lipid vesicles as carriers for introducing biologically active materials into cells. Methods Cell Biol 1976;14:33-71.
38. Liu R, Gan L, Yang X, Xu H. Chitosan as a condensing agent induces high gene transfection efficiency and low cytotoxicity of liposome. J Biosci Bioeng 2011;111:98-103.
39. Liu J, Boonkaew B, Arora J, et al. Comparison of sorafenib-loaded poly (lactic/glycolic) acid and DPPC liposome nanoparticles in the in vitro treatment of renal cell carcinoma. J Pharm Sci 2015;104:1187-96.
40. Yamada Y, Munechika R, Kawamura E, Sakurai Y, Sato Y, Harashima H. Mitochondrial delivery of doxorubicin using MITO-porter kills drug-resistant renal cancer cells via mitochondrial toxicity. J Pharm Sci 2017;106:2428-37.
41. Yu Z, Wang Y, Xu D, et al. G250 antigen-targeting drug-loaded nanobubbles combined with ultrasound targeted nanobubble destruction: a potential novel treatment for renal cell carcinoma. Int J Nanomed 2020;15:81-95.
43. Wang J, Potocny AM, Rosenthal J, Day ES. Gold nanoshell-linear tetrapyrrole conjugates for near infrared-activated dual photodynamic and photothermal therapies. ACS Omega 2020;5:926-40.
44. Seeley EH, Oppenheimer SR, Mi D, Chaurand P, Caprioli RM. Enhancement of protein sensitivity for MALDI imaging mass spectrometry after chemical treatment of tissue sections. J Am Soc Mass Spectrom 2008;19:1069-77.
45. Hájek R, Lísa M, Khalikova M, et al. HILIC/ESI-MS determination of gangliosides and other polar lipid classes in renal cell carcinoma and surrounding normal tissues. Anal Bioanal Chem 2018;410:6585-94.
46. Nizioł J, Rode W, Laskowska B, Ruman T. Novel monoisotopic 109AgNPET for laser desorption/ionization mass spectrometry. Anal Chem 2013;85:1926-31.
47. Nizioł J, Ossoliński K, Tripet BP, Copié V, Arendowski A, Ruman T. Nuclear magnetic resonance and surface-assisted laser desorption/ionization mass spectrometry-based metabolome profiling of urine samples from kidney cancer patients. J Pharm Biomed Anal 2021;193:113752.
48. Fujishima A, Honda K. Electrochemical photolysis of water at a semiconductor electrode. Nature 1972;238:37-8.
49. Yang C, Zhu Y, Li D, et al. Red phosphorus decorated TiO2 nanorod mediated photodynamic and photothermal therapy for renal cell carcinoma. Small 2021;17:e2101837.
50. Soprano E, Polo E, Pelaz B, Del Pino P. Biomimetic cell-derived nanocarriers in cancer research. J Nanobiotechnol 2022;20:538.
51. von Rundstedt FC, Scovell JM, Agrawal S, Zaneveld J, Link RE. Utility of patient-specific silicone renal models for planning and rehearsal of complex tumour resections prior to robot-assisted laparoscopic partial nephrectomy. BJU Int 2017;119:598-604.
52. Li H, Yang C, Wang K, et al. Grading and surgical treatment of renal cell carcinoma combined with inferior vena cava carcinoma thrombosis. J Min Invas Urol 2022;11:342-6.
53. Ciancio G. Inferior Vena cava reconstruction using a ringed polytetrafluoroethylene interposition graft and inferior vena cava filter placement following resection of renal cell carcinoma with a tumor thrombus directly infiltrating the inferior vena cava. Vasc Endovascular Surg 2022;56:5-10.
54. Gradilone A, Iacovelli R, Cortesi E, et al. Circulating tumor cells and “suspicious objects” evaluated through CellSearch® in metastatic renal cell carcinoma. Anticancer Res 2011;31:4219-21.
55. Zhang Y, Rana A, Stratton Y, Czyzyk-Krzeska MF, Esfandiari L. Sequence-specific detection of MicroRNAs related to clear cell renal cell carcinoma at fM concentration by an electroosmotically driven nanopore-based device. Anal Chem 2017;89:9201-8.
56. Arendowski A, Ossoliński K, Ossolińska A, Ossoliński T, Nizioł J, Ruman T. Serum and urine analysis with gold nanoparticle-assisted laser desorption/ionization mass spectrometry for renal cell carcinoma metabolic biomarkers discovery. Adv Med Sci 2021;66:326-35.
57. Nizioł J, Sunner J, Beech I, et al. Localization of metabolites of human kidney tissue with infrared laser-based selected reaction monitoring mass spectrometry imaging and silver-109 nanoparticle-based surface assisted laser desorption/ionization mass spectrometry imaging. Anal Chem 2020;92:4251-8.
58. Zhang L, Yu C, Gao R, et al. An impedimetric biosensor for the diagnosis of renal cell carcinoma based on the interaction between 3-aminophenyl boronic acid and sialic acid. Biosens Bioelectron 2017;92:434-41.
59. Niciński K, Krajczewski J, Kudelski A, et al. Detection of circulating tumor cells in blood by shell-isolated nanoparticle - enhanced Raman spectroscopy (SHINERS) in microfluidic device. Sci Rep 2019;9:9267.
60. Arendowski A, Nizioł J, Ossoliński K, et al. Laser desorption/ionization MS imaging of cancer kidney tissue on silver nanoparticle-enhanced target. Bioanalysis 2018;10:83-94.
61. Renner AM, Derichsweiler C, Ilyas S, Gessner I, Fries JWU, Mathur S. High efficiency capture of biomarker miRNA15a for noninvasive diagnosis of malignant kidney tumors. Biomater Sci 2022;10:1113-22.
62. Zhang X, Liu R, Yuan Q, et al. The precise diagnosis of cancer invasion/metastasis via 2D laser ablation mass mapping of metalloproteinase in primary cancer tissue. ACS Nano 2018;12:11139-51.
63. Himbert D, Zeuschner P, Ayoubian H, Heinzelmann J, Stöckle M, Junker K. Characterization of CD147, CA9, and CD70 as Tumor-specific markers on extracellular vesicles in clear cell renal cell carcinoma. Diagnostics 2020;10:1034.
64. Lu C, Li J, Xu K, et al. Fabrication of mAb G250-SPIO molecular magnetic resonance imaging nanoprobe for the specific detection of renal cell carcinoma in vitro. PLoS One 2014;9:e101898.
65. Guimaraes AR, Tabatabei S, Dahl D, McDougal WS, Weissleder R, Harisinghani MG. Pilot study evaluating use of lymphotrophic nanoparticle-enhanced magnetic resonance imaging for assessing lymph nodes in renal cell cancer. Urology 2008;71:708-12.
66. Kuusk T, De Bruijn R, Brouwer OR, et al. Lymphatic drainage from renal tumors in vivo: a prospective sentinel node study using SPECT/CT imaging. J Urol 2018;199:1426-32.
67. Li J, Wu C, Hou P, Zhang M, Xu K. One-pot preparation of hydrophilic manganese oxide nanoparticles as T1 nano-contrast agent for molecular magnetic resonance imaging of renal carcinoma in vitro and in vivo. Biosens Bioelectron 2018;102:1-8.
68. Zhu L, Wang L, Liu Y, Xu D, Fang K, Guo Y. CAIX aptamer-functionalized targeted nanobubbles for ultrasound molecular imaging of various tumors. Int J Nanomed 2018;13:6481-95.
69. Funasaki S, Mehanna S, Ma W, et al. Targeting chemoresistance in Xp11.2 translocation renal cell carcinoma using a novel polyamide-chlorambucil conjugate. Cancer Sci 2022;113:2352-67.
70. Lee HW, Seo HS, Yeom SY, et al. Cabozantinib-loaded PLGA nanoparticles: a potential adjuvant strategy for surgically resected high-risk non-metastatic renal cell carcinoma. Int J Mol Sci 2022;23:12634.
71. Gao X, Jiang P, Zhang Q, et al. Peglated-H1/pHGFK1 nanoparticles enhance anti-tumor effects of sorafenib by inhibition of drug-induced autophagy and stemness in renal cell carcinoma. J Exp Clin Cancer Res 2019;38:362.
72. Fujii H, Shin-Ya M, Takeda S, et al. Cycloamylose-nanogel drug delivery system-mediated intratumor silencing of the vascular endothelial growth factor regulates neovascularization in tumor microenvironment. Cancer Sci 2014;105:1616-25.
73. Yongvongsoontorn N, Chung JE, Gao SJ, et al. Carrier-enhanced anticancer efficacy of sunitinib-loaded green tea-based micellar nanocomplex beyond tumor-targeted delivery. ACS Nano 2019;13:7591-602.
74. Takke A, Shende P. Magnetic-core-based silibinin nanopolymeric carriers for the treatment of renal cell cancer. Life Sci 2021;275:119377.
75. Keefe SM, Hoffman-Censits J, Cohen RB, et al. Efficacy of the nanoparticle-drug conjugate CRLX101 in combination with bevacizumab in metastatic renal cell carcinoma: results of an investigator-initiated phase I-IIa clinical trial. Ann Oncol 2016;27:1579-85.
76. Racaniello GF, Laquintana V, Vergnaud J, et al. Development of purified glycogen derivatives as siRNA nanovectors. Int J Pharm 2021;608:121128.
77. Takara K, Hatakeyama H, Kibria G, Ohga N, Hida K, Harashima H. Size-controlled, dual-ligand modified liposomes that target the tumor vasculature show promise for use in drug-resistant cancer therapy. J Control Release 2012;162:225-32.
78. Kibria G, Hatakeyama H, Ohga N, Hida K, Harashima H. The effect of liposomal size on the targeted delivery of doxorubicin to Integrin αvβ3-expressing tumor endothelial cells. Biomaterials 2013;34:5617-27.
79. Takai T, Tsujino T, Yoshikawa Y, et al. Synthetic miR-143 exhibited an anti-cancer effect via the downregulation of K-RAS networks of renal cell cancer cells in vitro and in vivo. Mol Ther 2019;27:1017-27.
80. Chai D, Liu N, Li H, et al. H1/pAIM2 nanoparticles exert anti-tumour effects that is associated with the inflammasome activation in renal carcinoma. J Cell Mol Med 2018;22:5670-81.
81. Ni W, Li Y, Liang L, et al. Tumor microenvironment-responsive nanodrug for clear-cell renal cell carcinoma therapy via triggering waterfall-like cascade ferroptosis. J Biomed Nanotechnol 2022;18:327-42.
82. Zhai X, Yuan S, Yang X, et al. Chitosan oligosaccharides induce apoptosis in human renal carcinoma via reactive-oxygen-species-dependent endoplasmic reticulum stress. J Agric Food Chem 2019;67:1691-701.
83. Tannir NM, Papadopoulos KP, Wong DJ, et al. Pegilodecakin as monotherapy or in combination with anti-PD-1 or tyrosine kinase inhibitor in heavily pretreated patients with advanced renal cell carcinoma: final results of cohorts A, G, H and I of IVY phase I study. Int J Cancer 2021;149:403-8.
84. Cai B, Hou M, Zhang S, et al. Dual targeting of endoplasmic reticulum by redox-deubiquitination regulation for cancer therapy. Int J Nanomed 2021;16:5193-209.
85. Kannadorai RK, Chiew GGY, Luo KQ, Liu Q. Dual functions of gold nanorods as photothermal agent and autofluorescence enhancer to track cell death during plasmonic photothermal therapy. Cancer Lett 2015;357:152-9.
86. Bex A, Vermeeren L, de Windt G, Prevoo W, Horenblas S, Olmos RA. Feasibility of sentinel node detection in renal cell carcinoma: a pilot study. Eur J Nucl Med Mol Imaging 2010;37:1117-23.
87. Lang Y, Tian X, Dong HY, et al. Black phosphorus quantum dots enhance the radiosensitivity of human renal cell carcinoma cells through inhibition of DNA-PKcs kinase. Cells 2022;11:1651.
88. Chen J, Ren F, Cao W, et al. Photothermal therapy enhance the anti-mitochondrial metabolism effect of lonidamine to renal cell carcinoma in homologous-targeted nanosystem. Nanomedicine 2021;34:102370.
89. Yamamoto S, Kato A, Sakurai Y, Hada T, Harashima H. Modality of tumor endothelial VEGFR2 silencing-mediated improvement in intratumoral distribution of lipid nanoparticles. J Control Release 2017;251:1-10.
90. Whiteside TL. Tumor-derived exosomes and their role in cancer progression. Adv Clin Chem 2016;74:103-41.
91. Mao W, Wang K, Wu Z, Xu B, Chen M. Current status of research on exosomes in general, and for the diagnosis and treatment of kidney cancer in particular. J Exp Clin Cancer Res 2021;40:305.
92. Kahlert C, Kalluri R. Exosomes in tumor microenvironment influence cancer progression and metastasis. J Mol Med 2013;91:431-7.
93. Mathieu M, Martin-Jaular L, Lavieu G, Théry C. Specificities of secretion and uptake of exosomes and other extracellular vesicles for cell-to-cell communication. Nat Cell Biol 2019;21:9-17.
94. Kalluri R, LeBleu VS. The biology, function, and biomedical applications of exosomes. Science 2020;367:eaau6977.
95. Liu Y, Wu W, Cai C, Zhang H, Shen H, Han Y. Patient-derived xenograft models in cancer therapy: technologies and applications. Signal Transduct Target Ther 2023;8:160.
96. Voss MH, Hussain A, Vogelzang N, et al. A randomized phase II trial of CRLX101 in combination with bevacizumab versus standard of care in patients with advanced renal cell carcinoma. Ann Oncol 2017;28:2754-60.
97. Boorjian SA, Milowsky MI, Kaplan J, et al. Phase 1/2 clinical trial of interferon alpha2b and weekly liposome-encapsulated all-trans retinoic acid in patients with advanced renal cell carcinoma. J Immunother 2007;30:655-62.
98. Johannsen M, Spitaleri G, Curigliano G, et al. The tumour-targeting human L19-IL2 immunocytokine: preclinical safety studies, phase I clinical trial in patients with solid tumours and expansion into patients with advanced renal cell carcinoma. Eur J Cancer 2010;46:2926-35.
99. Lasorsa F, Rutigliano M, Milella M, et al. Cancer stem cells in renal cell carcinoma: origins and biomarkers. Int J Mol Sci 2023;24:13179.
100. Xiong Z, Xiao W, Bao L, et al. Tumor cell “slimming” regulates tumor progression through PLCL1/UCP1-mediated lipid browning. Adv Sci 2019;6:1801862.
101. Xiong Z, Xiong W, Xiao W, et al. NNT-induced tumor cell “slimming” reverses the pro-carcinogenesis effect of HIF2a in tumors. Clin Transl Med 2021;11:e264.
Cite This Article
How to Cite
Download Citation
Export Citation File:
Type of Import
Tips on Downloading Citation
Citation Manager File Format
Type of Import
Direct Import: When the Direct Import option is selected (the default state), a dialogue box will give you the option to Save or Open the downloaded citation data. Choosing Open will either launch your citation manager or give you a choice of applications with which to use the metadata. The Save option saves the file locally for later use.
Indirect Import: When the Indirect Import option is selected, the metadata is displayed and may be copied and pasted as needed.
About This Article
Copyright
Data & Comments
Data
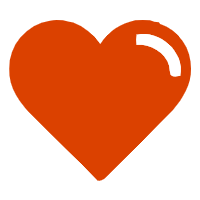
Comments
Comments must be written in English. Spam, offensive content, impersonation, and private information will not be permitted. If any comment is reported and identified as inappropriate content by OAE staff, the comment will be removed without notice. If you have any queries or need any help, please contact us at [email protected].