Some synergetic therapy strategies for overcoming hypoxia for photodynamic therapy of cancer
Abstract
As an emerging strategy in antitumor therapy, photodynamic therapy (PDT) has garnered significant attention in recent years for the treatment of various malignant tumors. This is due to its low side effects, superior spatial selectivity, and maximum preservation of normal tissue function. However, the hypoxic nature of tumors, continuous oxygen consumption, and microvascular damage associated with PDT treatment have impeded its development. Therefore, the focus of antitumor therapy has shifted towards enhancing the efficacy of PDT by addressing tumor hypoxia. The objective of this review is to assess and summarize the recent advancements in tumor treatment using synergistic therapy strategies (PDT+X, where X represents photothermal therapy, chemodynamic therapy, chemotherapy, immunotherapy, Photoacoustic therapy, etc.) that overcome hypoxia. Additionally, this review aims to outline the advantages and disadvantages of various collaborative methods for improving tumor hypoxia, while also discussing the challenges that lie ahead for future research.
Keywords
INTRODUCTION
Cancer causes millions of deaths every year and thus strongly impacts our society[1-4]. The recent years have witnessed the emergence of diverse tumor treatment modalities with different efficacies and limitations, as exemplified by surgery, radiotherapy, chemotherapy, endocrine therapy, immunotherapy, and molecular targeted therapy[1,5]. Photodynamic therapy (PDT) has become a promising method of cancer treatment, offering the benefits of minimal invasiveness, high effectiveness, high selectivity, and low toxicity[6-8]. PDT has a track record of application to colon[9], lung[10,11], prostate[12], head and neck[13-15], brain[16], skin[17], pancreas[18], and breast[19] cancers.
The general mechanism of PDT involves three primary elements, namely light with a specific wavelength, a photosensitizer (PS), and molecular oxygen[20-23]. Presently, most PDT processes occur only when oxygen is present[24-26]. Nonetheless, PDT can also occur in hypoxic environments even without oxygen[27]. Upon irradiation with specific-wavelength light, PSs generate reactive oxygen species (ROS), which may contribute to cell death (e.g., apoptosis, necrosis, autophagy, ferroptosis, pyroptosis, necroptosis, parthanatos, and mitotic catastrophe), microvascular system destruction, and immune responses, via two (type-I and type-II) routes. Initially, the PS ground state (PS0) absorbs light to afford an unstable and short-lived excited singlet state (1PS) that can easily return to PS0 by releasing light energy (fluorescence) or thermal energy (nonradiative decay). Alternatively, 1PS can experience intersystem crossing to afford a more stable and longer-lived triplet state (3PS) that can return to PS0 by releasing light energy (fluorescence/phosphorescence) or thermal energy. Most importantly, 3PS can react with various substances through type-I and type-II routes to generate ROS. In the type-I route, 3PS engages in electron transfer with the surrounding cellular substrates to form free radicals capable of generating ROS (O2•-, •OH, and H2O2). In the type-II route, 3PS transfers energy to 3O2 and converts it into the highly reactive 1O2. The toxic ROS generated from 3PS exert antitumor effects by promoting various biological processes, mainly tumor cell killing, tumor vessel damage, and tumor immune reactions [Figure 1][5]. Although type-I and type-II routes can occur simultaneously, the latter route is believed to be dominant for clinically proven PSs[28].
Figure 1. Schematic illustration of the photochemical reactions for type I and type II PDT and related antitumor effects.
Solid tumors are intrinsically prone to rapid proliferation, which inevitably results in hypoxic tumor microenvironments (TMEs)[29]. In solid tumors, O2 concentration varies by location, and the deeper the solid tumor site, the lower the oxygen concentration[29]. We know that, in the absence of oxygen, type II-PDT can no longer function to produce ROS for tumor destruction. Additionally, as tumors progress rapidly, continuous oxygen consumption increases tumor hypoxia; therefore, PDT is significantly less effective against tumors[25]. Tumor hypoxia is usually classified as chronic or acute. The more prevalent chronic hypoxia is caused by the increase in the distance of O2 diffusion from tumor vessels during tumor growth[30]. Hypoxia could dramatically decrease the efficacy of PDT, influence the genomic and proteomic changes of tumor cells, and result in tumor progression, tumor invasion, and metastasis[31-33]. Moreover, hypoxia upregulates the expression of hypoxia-inducible factor 1 (HIF-1), which induces autophagy to protect tumor cells and thus results in resistance to PDT[34].
So far, much effort has been directed at overcoming the hypoxia-associated limitations of PDT, as outlined in several reviews that mainly focus on methods of increasing O2 levels (e.g., via the generation of O2 in tumor sites and direct O2 delivery into tumors) or reducing O2 consumption[3,29,35]. Photodynamic therapy (PDT) is subject to severe limitations such as limited tissue penetration depth[36], hyperoxygen dependence[37,38] and phototoxicity[39,40]. In order to overcome the limitation of PDT, many strategies have been proposed, such as the emergence of non-photo-induced photosensitizers, which can effectively overcome the limitation of penetration depth and phototoxicity. PDT combined with anoxic therapy can increase the oxygen content of tumor microenvironment effectively, thus enhancing the effect of PDT. In this review, we concentrate on some strategies that have synergy between different therapies to overcome hypoxia. Next, we use examples to illustrate how these strategies work, and in the last, we discuss the challenges and future prospects associated with these strategies.
SYNERGETIC THERAPY STRATEGIES
Combination of PDT with photothermal therapy
As a non-oxygen-dependent cancer treatment, photothermal therapy (PTT) has been developed to improve antitumor effects via the use of photothermal agents to convert optical energy into thermal energy and thus cause irreversible cell damage[25,41-43]. An increase in local temperature (mild heating) was shown to increase the tumor blood flow and O2 content[44-46]. Therefore, PTT is a favorable method of overcoming tumor hypoxia and enhancing the efficacy of PDT. PTT treatment is known to cause the upregulation of the heat shock protein (HSP), which protects tumor cells[47]. Given that PDT-generated ROS were reported to downregulate HSP[48], PDT can improve the efficacy of PTT. So far, various materials have been designed for synergetic PPT-PDT treatment, including magnetic melanin[49,50], CuS[51,52], Prussian blue nanoparticles[53-57], poly(dopamine) (PDA)[58-60], gold nanospheres[61-63], graphene oxides[64-66], BP[67-69], and WS2 nanosheets[70].
Song et al. created a PTT-PDT self-synergetic nanoplatform (RGD-BPNS@SMFN) based on temperature-dependent CAT-like (Catalase is an enzyme that catalyzes the decomposition of hydrogen peroxide into oxygen and water) effects to eliminate tumor[71] [Figure 2A]. This kind of self-synergetic phenomenon was found due to the PTT-promoted inherent CAT-like activity which increased the O2 concentration of TME and further enhanced PDT efficiency. Photothermal performance is shown in Figure 2B, BPNS@SMFN elevated the temperature under irradiation by 808 nm from 25 °C to 45 °C. Meanwhile, photodynamic performance is shown in Figure 2C, BPNS@SMFN generated ROS inferiorly to the bare BPNS without
Figure 2. (A) The targeted self-synergetic phototherapy process of the nanoplatform as prepared based on the temperature-dependent CAT-like behavior; (B) photothermal curves of BPNS, SMFN and BPNS@SMFN dispersion under 808 nm laser irradiation; (C) Time-dependent absorbance values at 415 nm of the different samples after mixing with DPBF; (D) In vitro cell viabilities of HeLa cells after different treatments with nanomaterials as-synthesized; (E) oxygen generation curves of three materials (SMFN, BPNS, BPNS@SMFN) at different pH (pH 6.5 and 7.4) over time; (F) the oxygen generation curves of different materials under different treatment mixed with fixed H2O2 concentration (500 × 10-3 m) over time tumor volumes variation in different treatment groups with time. Reprinted from ref.[71] with permission. **P < 0.01, Copyright 2022, Wiley-VCH.
Combination of PDT with hypoxia-activated therapy
Given the difficulty of increasing intratumoral oxygen levels and the limitations of monotherapies, several strategies have been developed to overcome the hypoxia limitation of PDT and have achieved marginal benefits. Hypoxia-activated prodrugs (HAPs), which act only under hypoxic conditions and are significantly cytotoxic to hypoxic cells but have little effect on normal cells, hold great promise for the enhancement of PDT efficiency[72].
Several studies combined PSs and HAPs into nanoparticles. He et al. chose banoxantrone (AQ4N), which can be enzymatically converted into toxic AQ4 under hypoxic conditions, as the HAP, and designed a covalent organic framework (COF)-based AQ4N-encapsulating nanoplatform (THPPTK-PEG NPs) composed of a PS (tetra(4-hydroxyphenyl)porphine (THPP)) and a 1O2-cleavable thioketal (TK) linker[73]. After laser irradiation at 660 nm, THPPTK-PEG NPs generated large amounts of cytotoxic ROS with antitumor activity, as the PS consumed large amounts of O2 and then aggravated the hypoxia within the tumor. Simultaneously, PDT-generated ROS cleaved the TK linker to disintegrate the COF and selectively release AQ4N into the tumor. Both in vivo and In vitro experiments showed that the highest cytotoxicity and tumor inhibition efficacy were observed for the THPPTK-PEG + laser group. Furthermore, HIF-1α staining revealed that THPPTK-PEG NPs and AQ4N@THPPTK-PEG NPs may aggravate tumor hypoxia after laser irradiation, resulting in hypoxia-activated cascade chemotherapy in tumors. Therefore, hypoxia-activated therapy and PDT have a synergistic effect on anticancer activity.
Combination of PDT with chemodynamic therapy
chemodynamic therapy (CDT), an oxidation therapy, has attracted much attention due to its ability to generate ROS via Fenton-type and Fenton-like reactions[74,75]. Specifically, CDT uses transition metal (e.g., Fe, Mn, Cu, Ni, and Co) catalysts to convert H2O2 into cytotoxic •OH and thus inflict significant oxidative damage to tumor cells[76-83]. Unlike O2, H2O2 is highly abundant in TMEs, and CDT is therefore not affected by hypoxia[84,85] and may be a promising strategy for enhancing PDT antitumor efficacy.
So far, Fe-based materials are the most suitable catalysts for therapeutic applications, generating •OH radicals and thus inducing lipid peroxidation (LPO) in tumor cells[86]. Fenton-type reactions can be represented as follows[75]:
Fe2+ + H2O2 → Fe3+ + •OH + OH-,
Fe2+ + •OH → Fe2+ + •OOH + H+,
Fe3+ + •OOH → Fe2+ + O2 + H+.
Unlike PDT, which induces apoptosis, Fe-based CDT treatment inflicts iron-dependent LPO-associated oxidative damage that induces regulated cell death, which can also be denoted as ferroptosis[86-89]. The combination of PDT and ferroptosis has been developed into a promising strategy. Ferroptosis-inducing agents can be generally classified as nanoparticle platforms (e.g., inorganic Fe-containing nanoparticles[90] and Fe-organic frameworks such as Fe-MOFs[91]) and protein-based nanocarriers (e.g., ferritin[92]). Several nanoparticles have shown a significant capability for enhancing antitumor efficacy but exhibited some drawbacks related to biocompatibility and tumor-targeting prospects[93].
Wang et al. designed a novel hypoxia-responsive nanoreactor BCFe@SRF for cancer synergistic therapy. They encapsulated the covalently crosslinked Ce6, bovine serum albumin (BSA) and ferritin, together with sorafenib (SRF) inside a protein [Figure 3A][94]. BSA-Ce6 is a prospecting protein-based PDT material. Ferritin acted as ferroptosis inducer, which converted H2O2 into •OH to amplify ROS concentration. SRF not only destroyed the oxidative defense system of tumor but also promoted ferroptosis for further enhancement. Azobenzene (Azo) was used as a cross-linker in the BCFe@SRF, which can be cleaved under hypoxic conditions. Thus, under hypoxic conditions, BCFe@SRF could be degraded to release Ce6 for photodynamic therapy and ferritin for chemodynamic therapy [Figure 3B]. After 670 nm light irradiation, BC-treated cells exhibited a 47.1% viability under hypoxia compared to 41.0% under normoxia, which indicates that hypoxia affects PDT efficiency. Meanwhile, BCFe@SRF treated cells had a 12.4% viability under hypoxia compared to 36.0% viability under normoxic conditions, owing to the degradation of Azo-crosslinked nanosystems which released more Ce6 to enhance therapeutic efficacy [Figure 3C]. Similarly, with the CCK-8 assay, the BCFe@SRF + laser + hypoxia group demonstrated the best ability to kill cancer cells in the live/dead staining assay [Figure 3D]. To verify the hypothetic mechanism of BCFe@SRF, Fer-1 was used as an inhibitor for ferroptosis in the vitro experiments. CCK-8 assay showed that the toxicity was obviously inhibited after adding Fer-1 [Figure 3E] through inhibited ROS generation [Figure 3F]. In vivo performance, compared to single PDT (BC + laser) treated group or ferroptosis group (BCFe@SRF), BCFe@SRF + laser group exhibited greater tumor inhibition and reduction [Figure 3G and H].
Figure 3. (A) Fabrication procedures and antitumor mechanisms of BCFe@SRF nanoreactor for the designed synergistic PDT and ferroptosis therapy; (B) TEM images of a BSA-Ce6, BCFe@SRF, and BCFe@SRF degradation product; (C) A CCK-8 cell viability assay of hepa 1-6 cells treated with BCFe@SRF (Ce6 concentration: 1 μM) mediated PDT (670 nm light, 50 mW·cm-2, 5 min) in normoxic or hypoxia condition; (D) Live/dead staining assay (green: live cells; red: dead cells); (C) CCK-8 cell viability assay; (E) CCK-8 cell viability assay; (F) Fluorescence microscopy images of hepa 1-6 cells treated with different formulations and ROS indicator DCFH-DA with or without laser irradiation in hypoxic condition; (G) Time-dependent tumor growth curves; (H) average weights of the excited tumors at the end of the indicated treatment. Reprinted from ref.[94] with permission. *P < 0.05; ***P < 0.001; ###P < 0.001. Copyright 2022, BMC.
Zhou et al. suggested that the efficacy of ROS is limited by their short half-life and low diffusion radius and speculated that the direct targeting of vital ROS-sensitive organelles such as mitochondria could enhance efficacy[95]. To test this hypothesis, the authors developed a multifunctional nanoplatform targeting and damaging mitochondria through a combination of PDT and Fenton reactions (Fe3O4@Dex/TPP/PpIX/ss-MPEG)[96]. Fe3O4 decomposed and diffused into the cytoplasm to react with H2O2 and generate O2 and •OH. Nondecomposed nanoparticles were localized in mitochondria and then directly generated ROS. Furthermore, the Fenton reaction-produced O2 could also be utilized as a raw material for PDT and thus increase its efficiency. In this way, Fenton reactions combined with PDT can greatly enhance tumor treatment[83,82].
However, the related clinical translation is limited by the insufficient generation of •OH and the low rate of Fe-based Fenton reactions[97]. Cu-based Fenton-like reactions have a greater CDT potential than Fe-based Fenton reactions because of the adaptability to weakly acidic TMEs, high rate of •OH generation, and greater rate of the former. The redox properties of Cu are strikingly similar to those of Fe, e.g., both Cu+ and Cu2+ easily react with H2O2[83,98-100]:
Cu2+ + H2O2 → Cu+ + HO2• + OH-,
Cu+ + H2O2 → Cu2+ + •OH + OH-.
The Cu2+/H2O2 Fenton-like system is applicable over a broader pH range than the Fe3+/H2O2 system in view of the higher solubility[101]. In addition, Cu2+ complexes are more easily decomposed by •OH than Fe3+ complexes, which precludes the deactivation of Fenton reactions[102]. Furthermore, Cu2+ can be reduced by GSH to increase the concentration of redox-active species (Cu+) used to generate •OH (Cu2+ + GSH → Cu+ + GSSG)[103]. Such characteristics highlight the favorable properties of the Cu2+/H2O2 Fenton-like system for enhancing the efficacy of antitumor therapies.
In a study by Li et al., Cu2+-mediated protein self-assembly (C-m-ABs) was developed by integrating copper with photosensitizer (ICG)[104]. Under light irradiation, C-m-Abs activates Photo-Fenton-like reaction to generate large ROS through the reduction of Cu2+ and ICG simultaneously [Figure 4A]. As shown in Figure 4B, ROS generation capacity was measured using 1,3-diphenylisobenzofuran, which is used as a ROS probe. Compared to the control group, DPBF suffered significant degradation after the addition of copper agents, and it should be noted that C-m-Abs + GSH treated group exhibited the fastest degradation rate of DFBF. In vitro cell-killing experiments showed that C-m-Abs exhibited excellent cytotoxicity
Figure 4. (A) C-m-ABs displays Photo-Fenton-like activity in the schematic illustration; (B) An 8-min reaction time was used to deplete DPBF after undergoing different treatments; (C) The viability of MGC-803 cells was analyzed using C-m-ABs containing different levels of DOX at pH 7.4 or 5.0 for 12 h; (D) Cell viability of MGC-803 cells treated with C-m-ABs (no DOX) and ICG under laser irradiation (1.0 W cm-2 or 1.4 W cm-2); (E) To test MGC-803 cell viability, C-m-ABs and DOX + ICG were employed, respectively, under laser irradiation (1.0 W cm-2 or 1.4 W cm-2); (F) Visual images of excised tumor at 15 day after treated with DOX, DOX + ICG + laser
In addition to ROS generation, Cu-based Fenton-like reactions may be accompanied by the oxidation of the diamagnetic Cu+ to the paramagnetic Cu2+ by H2O2, which may be used to develop in situ-generated MRI contrast agents for tumor imaging and diagnosis. Liu et al. reported a nanomaterial for multimodal imaging-guided synergetic therapy (CDT + PTT) acting as a highly efficient CDT agent, photothermal conversion agent (PTT), and self-generated MRI contrast agent[105]. Considering all these properties of Fenton and Fenton-like agents, we believe that future works will promote the development of photo- and chemodynamic therapies.
Combination of PDT with chemotherapy
Chemotherapeutics can improve the sensitivity of tumor cells to ROS, while ROS can enhance the uptake of chemotherapeutics by tumor cells; the combination of PDT with chemotherapy has become one of the most common cancer treatments[106,107]. Given that many PS components are chemotherapeutics, as mentioned above, this approach is not discussed separately herein.
Combination of PDT with immunotherapy
With the use of immune checkpoint inhibitors (ICI), cytokines that stimulate lymphocytes, and CAR-T cells, immunotherapy has transformed cancer treatment[108-115]. However, only 10%-30% of patients gain benefits from ICIs, possibly because of the “cold tumor” phenomenon, which refers to the lack of tumor-infiltrating T-cells and immunosuppressive TMEs[116,117]. Immunogenic cell death (ICD) is currently viewed as a promising strategy for activating the immune TME to enhance the efficacy of immunotherapy. ICD releases tumor-specific antigens and DAMPs to elicit antigen-specific immune reactions[118-120]. Pyroptosis, an ICD-causing PDT, holds great promise for augmenting tumor immunogenicity to overcome tumor immune suppression[121]. Therefore, the synergistic effects of PDT and immunotherapy have attracted much attention in view of the capability of this combination to convert immunosuppression into immunogenic TMEs and thus intensify the efficacy of both PDT and immunotherapy[122-124]. Wan et al. focused on RGX-104, an optimal liver-X nuclear hormone receptor (LXRβ) agonist to eliminate myeloid-derived suppressor cell (MDSC)-caused immunosuppressive activity[125]. The authors copackaged RGX-104 and a PS (Ce6) and designed a pH-responsive size-transformable nanoparticle delivery system (MRC NPs)[126]. RGX-104 reduced MDSCs to propel antitumor immunity, while Ce6 induced pyroptosis to increase tumor immunogenicity and generated ROS in tumor sites. Most recently, X-ray-induced PDT was combined with immunotherapy in cancer treatment with very good outcomes[124].
Combination of PDT with photoacoustic therapy
Photoacoustic therapy (PAT) is relatively different from other tumor therapies, as it inflicts mechanical damage to tumor cells using photoacoustic shockwaves without considering phototoxicity and resistance[127]. The oxygen-independent nature of PAT increases its chances of overcoming hypoxia during PDT[128]. Zhang et al. developed a new photoacoustic/dynamic therapeutic (PADT) agent based on a new PS (Gd(III)-phthalocyanine, GdPc)[129], showing that it can simultaneously generate acoustic cavitation for photophysical damage and 1O2 for photochemical damage upon pulse-wave (PW) laser irradiation. In vitro experiments, after irradiation at 680 nm (0.3 W cm-2), GdPcP-pretreated cells displayed direct mechanical damage and large 1O2 contents. In other In vitro experiments, the activity of HepG2 cells in the PADT group significantly decreased (to 53.5% of the original) under hypoxic conditions, while that in the PDT group decreased only to 80.3%. Under
PDT with microwave radiation
Microwaves can propagate through all types of tissues and stimulate photocatalysis in order to produce ROS oxygen-independently by plasmonic effects caused by microwave radiation[130]. PDT induced by microwaves is an excellent replacement for PDT based on type I[131]. The effect of MW is to dilate the blood vessels, increase blood flow velocity, and, in turn, increase oxygen content while activating photosensitizers in the body[132]. For instance, Copper-cystamine (Cu-Cy), a new type of photosensitizer researched and invented by Chen’s team[133], can be activated by UV[134], microwave[100,135], ultrasound[136] and X-Ray[137-142] and generate large amounts of singlet oxygen for killing tumor cells[100]. As microwave radiation is utilized with Cu-Cy nanoparticles at the tumor site, this will create 1O2[130]. This form of therapy may also be used with other chemicals such as Iodine or Chloride[143]. These methods may provide other options in decreasing tumor size depending on the location.
According to Zhou’s study, through Cu-Cy nanoparticle-mediated microwave dynamic therapy, ferroptosis can be induced as a cancer treatment option[131]. They used RNO-ID and SOSG to verify that ROS could be produced after MW irradiation of Cu-Cy, and the results showed that singlet oxygen increased with the increase of MW irradiation time and Cu-Cy concentration [Figure 5A-C]. In vitro experiments, Human CRC cells HCT15 can be inhibited by Cu-Cy nanoparticles activated with MW. Cu-Cy at 40 μg/mL almost killed all cells after microwave activation [Figure 5D]. Significant inhibition of cell colony formation was observed in Figure 5E and F when MW-activated Cu-Cy nanoparticles were compared to other groups.
Figure 5. (A) ROS were detected by RNO-ID using Cu-Cy aqueous solution (0.2 mg/mL) irradiated with different MWs of different power for 5 min. (B) Cu-Cy aqueous solution (0.2 mg/mL) irradiated with MW at 20 W for different times was detected by the RNO-ID method. (C) A comparison of SOSG fluorescence intensity with different Cu-Cy concentrations after MW irradiation. (D) A live/dead staining image of HCT15 cells treated with Cu-Cy and MW irradiation (20 W, 3 min). (E) MW-induced colony formation in HCT15 cells. (F) A calculation was made to determine the average number of clones. (G) Experiment images showing each group’s results using the xenograft model. (H) Tumor mass changes. (I) Tumor volume changes. (J) An illustration of how Cu-Cy-mediated dynamic microwave therapy (MWDT) induces ferroptosis in colorectal cancer cells. (K) Western blot assay of GPX4 expression. (L) FCM assay of cellular LPO with BODIPY-C11 probe detection. (M) FCM analysis of relative fluorescence intensity of HCT15 stained with the C11 BODIPY probe. Scale bar: 50 μm; *P < 0.05; **P < 0.01; ***P < 0.001; ****P < 0.0001. Reprinted from ref.[131] with permission. Copyright 2022, Elsevier.
More sensitizers have been discovered in recent years, such as graphitic-phase carbon nitride (g-C3N4) quantum dots (QDs)[145], AIEgens (TPEPy-I and TPEPy-PF6)[146] and TiO2[147] nanoparticles. Researchers found that it can activate and produce ROS under MW irradiation, killing tumor cells. MWPDT produces singlet oxygen and increases the oxygen content of the tumor microenvironment, providing a new therapeutic method for tumor treatment. X-ray has been widely used in clinical cancer theranostics, because of its strong penetration ability[148]. X-ray can activate photosensitizers and produce reactive ROS. Existing studies have found that radiokinetic therapy (RDT) is an oxygen-dependent dynamic therapy, so it is not elaborated in this article.
PDT with radiotherapy
Radiotherapy (RT) is an important mode of tumor therapy[149], which mainly uses radiation to damage the DNA of tumor cells to cause cell death, and can also produce ROS to cause cell apoptosis and enhance the therapeutic effect of radiation therapy[150]. The effect of PDT on the killing of tumor cells is dependent on ROS[5]. In tumor microenvironments, ROS production is reduced by hypoxia, which was effectively overcome by RT produced ROS at the tumor site, thus enhancing the tumor therapeutic effect of PDT[137,151-159].
A novel W18O49 nanoparticle with photothermal effect and RT sensitization was synthesized by Wang et al., which can produce ROS in the presence of X-ray[160]. The anthracene endoperoxide derivative compound (abbreviated as EP) can generate ROS when exposed to the NIR. The ROS generated above will strengthen the effect of ROS on inducing apoptosis. So, they covalently connect W18O49 and EP to become W18O49@EP to achieve the purpose of combining PDT and RT [Figure 6A]. They used fluorescent probe H2DCFDA to assess the ability to generate ROS and found that the combination of EP and W18O49 NPs resulted in a large amount of ROS being produced [Figure 6B]. Their In vitro experiments found that almost all cells died after RT and PDT (W18O49@EP + Laser + X-ray), significantly improving the killing effect of the tumor compared to the control group. At the same time, the anoxia state of tumor cells was observed after the anoxia induction factor was labeled. The results showed that the anoxia state of the W18O49@EP + Laser + X-ray group was significantly improved. Ki67 index results also showed that the W18O49@EP + Laser + X-ray group effectively inhibited cell proliferation. The ROS removal experiment proved that W18O49@EPNPs can produce ROS, thus improving the efficacy of RT combined with PDT [Figure 6C-G]. Compared with the group, the tumors in the W18O49@EP + Laser + X-ray group basically disappeared after 2 w treatment, achieving a good therapeutic effect [Figure 6H and I]. Their results demonstrate that W18O49@EP releases ROS under near-infrared light (NIR) to achieve effective PDT without inducing hypoxia.
Figure 6. (A) The mechanism of W18O49@EP under NIR and X-ray. (B) The production of ROS within cells after various treatments. (C) A comparison of cell death following different treatments. Red: dead cells; green: living cells. (D) Hypoxia and intracellular ROS levels after different treatments. (E) Red fluorescence marks HIF-1 expression in cells after different treatments. (F) The expression of Ki67 in cells after different treatments is marked by red fluorescence. (G) Cell survival after different treatments. (H) Tumor-bearing mice’s body weight after different treatments. (I) Volumes of tumors in mice treated differently. Reprinted from ref.[160] with permission. Copyright 2022, frontiers.
Some other photosensitizers, such as AVPt@HP@MNPs[161]and AuNCs-ICG nanozymes[162], have been found to have the characteristics of catalase, catalyzing the decomposition of hydrogen peroxide into oxygen in tumors, effectively solving the problem of hypoxia in the tumor microenvironment, and significantly improving the efficacy of PDT combined with RT. At the same time, AuNCs-ICG nanozymes will gather in large numbers in the tumor area and absorb X-ray, thus increasing the radiation dose in the tumor area and improving the efficacy of radiotherapy.
Summary
PDT is an important treatment for cancer because it is efficient, selective, minimally invasive, and has low toxicity. It damages tumor cells, vessels, and immune responses by creating ROS using energy transfer to oxygen. However, solid tumors often lack oxygen, making them difficult to treat with PDT. We discovered two main ways to generate ROS with PDT: the type-I process and the type-II process. We also identified several synergistic therapy strategies, such as combining PDT with PTT, hypoxia-activated therapy, CDT, immunotherapy, PAT, chemotherapy, Microwave Radiation, and Radiotherapy. The advantages and disadvantages of these strategies are summarized in Table 1. However, deep PDT as a clinical tool is still being explored and evaluated. Computer-aided drug design and target drug delivery techniques are being used to develop more effective photosensitizers for PDT. Additionally, developing PDT materials that can induce multiple tumor cell death modalities simultaneously would improve treatment effectiveness and prevent resistance. Combining type-I PDT with other treatments using multifunctional nanomedicine may be an efficient way to achieve multi-mode collaborative therapy.
The comparison of the strategies to relieve tumor hypoxia
Strategy | Advantages | Disadvantages |
Synergetic therapy | ||
Combined PDT with photothermal therapy (PTT) | (1) In one sense, increasing local temperature (mild heating) increases tumor blood flow and O2 content, but at the same time, ROS generated by PDT can downregulate heat shock protein (HSP) expression | (1) Light penetration of tissues is limited; (2) This strategy needs to provide compelling efficacy and safety benefits |
Combined PDT with hypoxia-activated therapy | (1) Hypoxia-activated prodrugs (HAP), which act only under hypoxic conditions and have significant cytotoxicity in hypoxia cells but little effect on normal cells | (1) It is difficult for this strategy to retain and penetrate due to increased interstitial fluid pressure and a dense extracellular matrix |
Combined PDT with chemodynamic therapy (CDT) | (1) CDT utilizes Fenton or Fenton-like reactions, with transition metals as catalysts, to convert H2O2 to •OH to destroy tumor cells. In contrast with the O2, H2O2 is highly abundant in TME, so that CDT is not affected by hypoxia; (2) In addition to triggering by the endogenous chemical energy, CDT can modulate the hypoxia and immunosuppressive TME | (1) There are still some concerns about the biocompatibility, tumor-targeting capacity of current studies; (2) The therapeutic performance was still far from satisfactory |
Combined PDT with immunotherapy | (1) The synergistic effects of PDT and immunotherapy attracted more attention due to their capability to convert immunosuppression into immunogenic TME to intensify both PDT and immunotherapy efficacy | (1) The biodegradability of inorganic nanomaterials and polymer-based nanomedicines is typically poor, severely limiting their clinical application (2) Combination cancer therapies need more advanced nanomedicines to boost effectiveness and safety |
Combined PDT with photoacoustic therapy (PAT) | (1) Photoacoustic therapy (PAT) can cause the target tumor cells directly mechanical damage via the photoacoustic shockwave without considering the phototoxicity and resistance | (1) Some materials are toxic and have shallow penetration, which restrict their clinical application |
PDT with Microwave Radiation | (1) Microwave Radiation can dilate the blood vessels, increase blood flow velocity, and, in turn, increase oxygen content while activating photosensitizers and producing ROS in the body (2) Microwave Radiation has a strong penetration depth compared to ultraviolet light, and can be used for the treatment of deep tumors | (1) Research on MDT and EDT is still in its infancy |
PDT with Radiotherapy | (1) Radiotherapy (RT) is an important mode of tumor therapy, which mainly uses radiation to damage the DNA of tumor cells to cause cell death, and can also produce ROS to cause cell apoptosis and enhance the therapeutic effect of radiation therapy (2) The penetration force of Radiotherapy is stronger than ultraviolet light, microwave | (1) The disadvantages of radiotherapy, such as side effects |
DECLARATIONS
Acknowledgments
We are grateful for the support by the Natural Science Foundation of Hunan Province (2022JJ30798), the Guiding Project of Hunan Health Commission (202202083650), the extracurricular Scientific Research and Training Program for Medical Students of Central South University (202229KT1824 and 202229KT1845). Additionally, we acknowledge the support from the platform of Clinical Research Center for Medical Imaging in Hunan Province (2020SK4001). Figure 1 was created with BioRender.com.
Authors’ contributions
Searched the information, materials and updates and wrote the drafts: Chai T
Searched the information, materials and updates and wrote the drafts: Li Y (Ya Li)
Helped for editing, checking and formatting: Cai Y
Edited and formatted the figures: Li Y (Yiyang Li)
Formal analysis, checked the English: Temple H
Conceptualization, Instruction, Funding acquisition, and Writing, Visualization, Validation, Supervision: Kenar N, Lim HS, Chen X, Chen W
Availability of data and materials
Not applicable.
Financial support and sponsorship
This work was supported by the Natural Science Foundation of Hunan Province (2022JJ30798), the Guiding Project of Hunan Health Commission (202202083650), the extracurricular Scientific Research and Training Program for Medical Students of Central South University (202229KT1824 and 202229KT1845). Additionally, we acknowledge the support from the platform of Clinical Research Center For Medical Imaging In Hunan Province (2020SK4001).
Conflicts of interest
All authors declared that there are no conflicts of interest.
Ethical approval and consent to participate
Not applicable.
Consent for publication
Not applicable.
Copyright
© The Author(s) 2023.
REFERENCES
1. Chilakamarthi U, Giribabu L. Photodynamic therapy: past, present and future. Chem Rec. 2017;17:775-802.
2. Cheng YJ, Hu JJ, Qin SY, Zhang AQ, Zhang XZ. Recent advances in functional mesoporous silica-based nanoplatforms for combinational photo-chemotherapy of cancer. Biomaterials. 2020;232:119738.
3. Fan W, Huang P, Chen X. Overcoming the Achilles’ heel of photodynamic therapy. Chem Soc Rev. 2016;45:6488-519.
4. Miller KD, Nogueira L, Mariotto AB, et al. Cancer treatment and survivorship statistics, 2019. CA Cancer J Clin. 2019;69:363-85.
5. Chen J, Fan T, Xie Z, et al. Advances in nanomaterials for photodynamic therapy applications: status and challenges. Biomaterials. 2020;237:119827.
6. Sun W, Zhou Z, Pratx G, Chen X, Chen H. Nanoscintillator-mediated X-ray induced photodynamic therapy for deep-seated tumors: from concept to biomedical applications. Theranostics. 2020;10:1296-318.
7. Liu Z, Cao T, Xue Y, et al. Self-amplified photodynamic therapy through the O2 -mediated internalization of photosensitizers from a Ppa-bearing block copolymer. Angew Chem Int Ed. 2020;59:3711-7.
8. Tian J, Zhang W. Synthesis, self-assembly and applications of functional polymers based on porphyrins. Prog Polym Sci. 2019;95:65-117.
9. Yanovsky RL, Bartenstein DW, Rogers GS, Isakoff SJ, Chen ST. Photodynamic therapy for solid tumors: a review of the literature. Photodermatol Photoimmunol Photomed. 2019;35:295-303.
10. Wang K, Yu B, Pathak JL. An update in clinical utilization of photodynamic therapy for lung cancer. J Cancer. 2021;12:1154-60.
11. Zhang Q, Wu L, Liu S, et al. Targeted nanobody complex enhanced photodynamic therapy for lung cancer by overcoming tumor microenvironment. Cancer Cell Int. 2020;20:570.
13. Wu W, Shi L, Duan Y, et al. Nanobody modified high-performance AIE photosensitizer nanoparticles for precise photodynamic oral cancer therapy of patient-derived tumor xenograft. Biomaterials. 2021;274:120870.
14. Lu K, He C, Lin W. Nanoscale metal-organic framework for highly effective photodynamic therapy of resistant head and neck cancer. J Am Chem Soc. 2014;136:16712-5.
15. Rettig EM, D’Souza G. Epidemiology of head and neck cancer. Surg Oncol Clin N Am. 2015;24:379-96.
16. Quirk BJ, Brandal G, Donlon S, et al. Photodynamic therapy (PDT) for malignant brain tumors - where do we stand? Photodiagnosis Photodyn Ther. 2015;12:530-44.
17. Li X, Lovell JF, Yoon J, Chen X. Clinical development and potential of photothermal and photodynamic therapies for cancer. Nat Rev Clin Oncol. 2020;17:657-74.
18. Wang Y, Wang H, Zhou L, et al. Photodynamic therapy of pancreatic cancer: where have we come from and where are we going? Photodiagnosis Photodyn Ther. 2020;31:101876.
19. Zhang Z, Li A, Min X, et al. An ROS-sensitive tegafur-PpIX-heterodimer-loaded in situ injectable thermosensitive hydrogel for photodynamic therapy combined with chemotherapy to enhance the tegafur-based treatment of breast cancer. Biomater Sci. 2021;9:221-37.
20. Li M, Xu Y, Peng X, Kim JS. From low to No O2-dependent hypoxia photodynamic therapy (hPDT): a new perspective. ACC Chem Res. 2022;55:3253-64.
21. Qiu M, Wang D, Huang H, et al. A regioselectively oxidized 2D Bi/BiOx lateral nano-heterostructure for hypoxic photodynamic therapy. Adv Mater. 2021;33:e2102562.
22. Duan Z, Luo Q, Dai X, et al. Synergistic therapy of a naturally inspired glycopolymer-based biomimetic nanomedicine harnessing tumor genomic instability. Adv Mater. 2021;33:e2104594.
23. Nguyen VN, Yan Y, Zhao J, Yoon J. Heavy-atom-free photosensitizers: from molecular design to applications in the photodynamic therapy of cancer. ACC Chem Res. 2021;54:207-20.
24. O’Connor AE, Gallagher WM, Byrne AT. Porphyrin and nonporphyrin photosensitizers in oncology: preclinical and clinical advances in photodynamic therapy. Photochem Photobiol. 2009;85:1053-74.
25. Jin CS, Lovell JF, Chen J, Zheng G. Ablation of hypoxic tumors with dose-equivalent photothermal, but not photodynamic, therapy using a nanostructured porphyrin assembly. ACS Nano. 2013;7:2541-50.
26. Moan J, Sommer S. Oxygen dependence of the photosensitizing effect of hematoporphyrin derivative in NHIK 3025 cells. Cancer Res. 1985;45:1608-10.
27. Li Y, Zhang W, Niu J, Chen Y. Mechanism of photogenerated reactive oxygen species and correlation with the antibacterial properties of engineered metal-oxide nanoparticles. ACS Nano. 2012;6:5164-73.
28. Mattila H, Khorobrykh S, Havurinne V, Tyystjärvi E. Reactive oxygen species: reactions and detection from photosynthetic tissues. J Photochem Photobiol B. 2015;152:176-214.
29. Li X, Kwon N, Guo T, Liu Z, Yoon J. Innovative strategies for hypoxic-tumor photodynamic therapy. Angew Chem Int Ed. 2018;57:11522-31.
30. Sahu A, Kwon I, Tae G. Improving cancer therapy through the nanomaterials-assisted alleviation of hypoxia. Biomaterials. 2020;228:119578.
31. Sahu A, Choi WI, Tae G. Recent progress in the design of hypoxia-specific nano drug delivery systems for cancer therapy. Adv Ther. 2018;1:1800026.
32. LaGory EL, Giaccia AJ. The ever-expanding role of HIF in tumour and stromal biology. Nat Cell Biol. 2016;18:356-65.
34. Rodríguez ME, Catrinacio C, Ropolo A, Rivarola VA, Vaccaro MI. A novel HIF-1α/VMP1-autophagic pathway induces resistance to photodynamic therapy in colon cancer cells. Photochem Photobiol Sci. 2017;16:1631-42.
35. de Souza AL, Marra K, Gunn J, et al. Comparing desferrioxamine and light fractionation enhancement of ALA-PpIX photodynamic therapy in skin cancer. Br J Cancer. 2016;115:805-13.
36. Ding B, Shao S, Yu C, et al. Large-pore mesoporous-silica-coated upconversion nanoparticles as multifunctional immunoadjuvants with ultrahigh photosensitizer and antigen loading efficiency for improved cancer photodynamic immunotherapy. Adv Mater. 2018;30:e1802479.
37. Zhang Y, Bo S, Feng T, et al. A versatile theranostic nanoemulsion for architecture-dependent multimodal imaging and dually augmented photodynamic therapy. Adv Mater. 2019;31:e1806444.
38. Zhu H, Li Q, Shi B, et al. Dual-emissive platinum(II) metallacage with a sensitive oxygen response for imaging of hypoxia and imaging-guided chemotherapy. Angew Chem Int Ed. 2020;59:20208-14.
39. Liu Y, Liu CZ, Wang ZK, et al. Supramolecular organic frameworks improve the safety of clinically used porphyrin photodynamic agents and maintain their antitumor efficacy. Biomaterials. 2022;284:121467.
40. Caruso E, Cerbara M, Malacarne MC, Marras E, Monti E, Gariboldi MB. Synthesis and photodynamic activity of novel non-symmetrical diaryl porphyrins against cancer cell lines. J Photochem Photobiol B. 2019;195:39-50.
41. Jaque D, Martínez Maestro L, del Rosal B, et al. Nanoparticles for photothermal therapies. Nanoscale. 2014;6:9494-530.
42. Chen Q, Xu L, Liang C, Wang C, Peng R, Liu Z. Photothermal therapy with immune-adjuvant nanoparticles together with checkpoint blockade for effective cancer immunotherapy. Nat Commun. 2016;7:13193.
43. Li X, Peng XH, Zheng BD, et al. New application of phthalocyanine molecules: from photodynamic therapy to photothermal therapy by means of structural regulation rather than formation of aggregates. Chem Sci. 2018;9:2098-104.
44. Song CW, Rhee JG, Levitt SH. Effect of hyperthermia on hypoxic cell fraction in tumor. Int J Radiat Oncol Biol Phys. 1982;8:851-6.
45. Brizel DM, Scully SP, Harrelson JM, et al. Radiation therapy and hyperthermia improve the oxygenation of human soft tissue sarcomas. Cancer Res. 1996;56:5347-50.
46. Li W, Wang L, Sun T, et al. Characterization of nanoparticles combining polyamine detection with photodynamic therapy. Commun Biol. 2021;4:803.
47. Wang X, Chen M, Zhou J, Zhang X. HSP27, 70 and 90, anti-apoptotic proteins, in clinical cancer therapy (Review). Int J Oncol. 2014;45:18-30.
48. Sheng D, Liu T, Deng L, et al. Perfluorooctyl bromide & indocyanine green co-loaded nanoliposomes for enhanced multimodal imaging-guided phototherapy. Biomaterials. 2018;165:1-13.
49. Lin J, Wang M, Hu H, et al. Multimodal-imaging-guided cancer phototherapy by versatile biomimetic theranostics with UV and γ-irradiation protection. Adv Mater. 2016;28:3273-9.
50. Ding M, Shao K, Wu L, et al. A NO/ROS/RNS cascaded-releasing nano-platform for gas/PDT/PTT/immunotherapy of tumors. Biomater Sci. 2021;9:5824-40.
51. Li Y, Lu W, Huang Q, Huang M, Li C, Chen W. Copper sulfide nanoparticles for photothermal ablation of tumor cells. Nanomedicine. 2010;5:1161-71.
52. Li L, Rashidi LH, Yao M, et al. CuS nanoagents for photodynamic and photothermal therapies: phenomena and possible mechanisms. Photodign Photody Ther. 2017;19:5-14.
53. Cheng L, Gong H, Zhu W, et al. PEGylated Prussian blue nanocubes as a theranostic agent for simultaneous cancer imaging and photothermal therapy. Biomaterials. 2014;35:9844-52.
54. Cai X, Gao W, Ma M, et al. A prussian blue-based core-shell hollow-structured mesoporous nanoparticle as a smart theranostic agent with ultrahigh pH-responsive longitudinal relaxivity. Adv Mater. 2015;27:6382-9.
55. Sahu A, Lee JH, Lee HG, Jeong YY, Tae G. Prussian blue/serum albumin/indocyanine green as a multifunctional nanotheranostic agent for bimodal imaging guided laser mediated combinatorial phototherapy. J Control Release. 2016;236:90-9.
56. Sun L, Li Q, Hou M, et al. Light-activatable Chlorin e6 (Ce6)-imbedded erythrocyte membrane vesicles camouflaged Prussian blue nanoparticles for synergistic photothermal and photodynamic therapies of cancer. Biomater Sci. 2018;6:2881-95.
57. Yang R, Hou M, Gao Y, et al. Indocyanine green-modified hollow mesoporous Prussian blue nanoparticles loading doxorubicin for fluorescence-guided tri-modal combination therapy of cancer. Nanoscale. 2019;11:5717-31.
58. Zhang D, Wu M, Zeng Y, et al. Chlorin e6 conjugated poly(dopamine) nanospheres as PDT/PTT dual-modal therapeutic agents for enhanced cancer therapy. ACS Appl Mater Interfaces. 2015;7:8176-87.
59. Feng L, Tao D, Dong Z, et al. Near-infrared light activation of quenched liposomal Ce6 for synergistic cancer phototherapy with effective skin protection. Biomaterials. 2017;127:13-24.
60. Zeng W, Zhang H, Deng Y, et al. Dual-response oxygen-generating MnO2 nanoparticles with polydopamine modification for combined photothermal-photodynamic therapy. Chem Eng J. 2020;389:124494.
61. Vijayaraghavan P, Liu CH, Vankayala R, Chiang CS, Hwang KC. Designing multi-branched gold nanoechinus for NIR light activated dual modal photodynamic and photothermal therapy in the second biological window. Adv Mater. 2014;26:6689-95.
62. Li W, Guo X, Kong F, et al. Overcoming photodynamic resistance and tumor targeting dual-therapy mediated by indocyanine green conjugated gold nanospheres. J Control Release. 2017;258:171-81.
63. Yeo ELL, Cheah JU, Lim BY, Thong PSP, Soo KC, Kah JCY. Protein corona around gold nanorods as a drug carrier for multimodal cancer therapy. ACS Biomater Sci Eng. 2017;3:1039-50.
64. Yan X, Hu H, Lin J, et al. Optical and photoacoustic dual-modality imaging guided synergistic photodynamic/photothermal therapies. Nanoscale. 2015;7:2520-6.
65. Ding YF, Kwong CHT, Li S, et al. Supramolecular nanomedicine derived from cucurbit[7]uril-conjugated nano-graphene oxide for multi-modality cancer therapy. Biomater Sci. 2021;9:3804-13.
66. Zhang X, Ong’achwa Machuki J, Pan W, et al. Carbon nitride hollow theranostic nanoregulators executing laser-activatable water splitting for enhanced ultrasound/fluorescence imaging and cooperative phototherapy. ACS Nano. 2020;14:4045-60.
67. Lee G, Lee JH, Choi W, Kim C, Hahn SK. Hyaluronate-black phosphorus-upconversion nanoparticle complex for non-invasive theranosis of skin cancer. Biomacromolecules. 2022;23:3602-11.
68. Li L, Rashidi LH, Yao M, et al. CuS nanoagents for photodynamic and photothermal therapies: phenomena and possible mechanisms. Photodiagnosis Photodyn Ther. 2017;19:5-14.
69. Zhang W, Yu L, Jiang Y, Guo C. Phycocyanin-functionalized black phosphorus quantum dots enhance PDT/PTT therapy by inducing ROS and irreparable DNA damage. Biomater Sci. 2021;9:5302-18.
70. Yong Y, Zhou L, Gu Z, et al. WS2 nanosheet as a new photosensitizer carrier for combined photodynamic and photothermal therapy of cancer cells. Nanoscale. 2014;6:10394-403.
71. Song L, Chen B, Qin Z, et al. Temperature-dependent CAT-like RGD-BPNS@SMFN nanoplatform for PTT-PDT self-synergetic tumor phototherapy. Adv Healthc Mater. 2022;11:e2102298.
72. Feng L, Cheng L, Dong Z, et al. Theranostic liposomes with hypoxia-activated prodrug to effectively destruct hypoxic tumors post-photodynamic therapy. ACS Nano. 2017;11:927-37.
73. He H, Du L, Xue H, Wu J, Shuai X. Programmable therapeutic nanoscale covalent organic framework for photodynamic therapy and hypoxia-activated cascade chemotherapy. Acta Biomater. 2022;149:297-306.
74. Lin LS, Huang T, Song J, et al. Synthesis of copper peroxide nanodots for H2O2 self-supplying chemodynamic therapy. J Am Chem Soc. 2019;141:9937-45.
75. Fan JX, Peng MY, Wang H, et al. Engineered bacterial bioreactor for tumor therapy via fenton-like reaction with localized H2O2 generation. Adv Mater. 2019;31:e1808278.
76. Hu JJ, Chen Y, Li ZH, Peng SY, Sun Y, Zhang XZ. Augment of oxidative damage with enhanced photodynamic process and MTH1 inhibition for tumor therapy. Nano Lett. 2019;19:5568-76.
77. Tang Z, Liu Y, He M, Bu W. Chemodynamic therapy: tumour microenvironment-mediated fenton and fenton-like reactions. Angew Chem Int Ed. 2019;58:946-56.
78. Hwang E, Jung HS. Metal-organic complex-based chemodynamic therapy agents for cancer therapy. Chem Commun. 2020;56:8332-41.
79. Lin H, Chen Y, Shi J. Nanoparticle-triggered in situ catalytic chemical reactions for tumour-specific therapy. Chem Soc Rev. 2018;47:1938-58.
80. Hao YN, Zhang WX, Gao YR, Wei YN, Shu Y, Wang JH. State-of-the-art advances of copper-based nanostructures in the enhancement of chemodynamic therapy. J Mater Chem B. 2021;9:250-66.
81. Wang W, Jin Y, Xu Z, et al. Stimuli-activatable nanomedicines for chemodynamic therapy of cancer. Wiley Interdiscip Rev Nanomed Nanobiotechnol. 2020;12:e1614.
82. Chudal L, Pandey NK, Phan J, Johnson O, Li X, Chen W. Investigation of PPIX-Lipo-MnO2 to enhance photodynamic therapy by improving tumor hypoxia. Mater Sci Eng C. 2019;104:109979.
83. Chudal L, Pandey NK, Phan J, et al. Copper-cysteamine nanoparticles as a heterogeneous fenton-like catalyst for highly selective cancer treatment. ACS Appl Bio Mater. 2020;3:1804-14.
84. Liu Y, Zhen W, Jin L, et al. All-in-one theranostic nanoagent with enhanced reactive oxygen species generation and modulating tumor microenvironment ability for effective tumor eradication. ACS Nano. 2018;12:4886-93.
85. Kim J, Cho HR, Jeon H, et al. Continuous O2-evolving MnFe2O4 nanoparticle-anchored mesoporous silica nanoparticles for efficient photodynamic therapy in hypoxic cancer. J Am Chem Soc. 2017;139:10992-5.
86. Liang H, Guo J, Shi Y, Zhao G, Sun S, Sun X. Porous yolk-shell Fe/Fe3O4 nanoparticles with controlled exposure of highly active Fe0 for cancer therapy. Biomaterials. 2021;268:120530.
87. Shen Z, Song J, Yung BC, Zhou Z, Wu A, Chen X. Emerging strategies of cancer therapy based on ferroptosis. Adv Mater. 2018;30:e1704007.
88. Shen Z, Liu T, Li Y, et al. Fenton-reaction-acceleratable magnetic nanoparticles for ferroptosis therapy of orthotopic brain tumors. ACS Nano. 2018;12:11355-65.
89. Jiang Q, Wang K, Zhang X, et al. Platelet membrane-camouflaged magnetic nanoparticles for ferroptosis-enhanced cancer immunotherapy. Small. 2020;16:e2001704.
90. Yang B, Dai Z, Zhang G, et al. Ultrasmall ternary FePtMn nanocrystals with acidity-triggered dual-ions release and hypoxia relief for multimodal synergistic chemodynamic/photodynamic/photothermal cancer therapy. Adv Healthc Mater. 2020;9:e1901634.
91. Yang B, Ding L, Yao H, Chen Y, Shi J. A metal-organic framework (MOF) fenton nanoagent-enabled nanocatalytic cancer therapy in synergy with autophagy inhibition. Adv Mater. 2020;32:e1907152.
92. Park E, Chung SW. ROS-mediated autophagy increases intracellular iron levels and ferroptosis by ferritin and transferrin receptor regulation. Cell Death Dis. 2019;10:822.
93. Zhao P, Tang Z, Chen X, et al. Ferrous-cysteine-phosphotungstate nanoagent with neutral pH fenton reaction activity for enhanced cancer chemodynamic therapy. Mater Horiz. 2019;6:369-74.
94. Wang X, Wu M, Zhang X, et al. Hypoxia-responsive nanoreactors based on self-enhanced photodynamic sensitization and triggered ferroptosis for cancer synergistic therapy. J Nanobiotechnol. 2021;19:204.
95. Zhou L, Wu Y, Meng X, et al. Dye-anchored MnO nanoparticles targeting tumor and inducing enhanced phototherapy effect via mitochondria-mediated pathway. Small. 2018;14:e1801008.
96. Hou H, Huang X, Wei G, Xu F, Wang Y, Zhou S. Fenton reaction-assisted photodynamic therapy for cancer with multifunctional magnetic nanoparticles. ACS Appl Mater Interfaces. 2019;11:29579-92.
97. Feng W, Han X, Wang R, et al. Nanocatalysts-augmented and photothermal-enhanced tumor-specific sequential nanocatalytic therapy in both NIR-I and NIR-II biowindows. Adv Mater. 2019;31:e1805919.
98. Bokare AD, Choi W. Review of iron-free Fenton-like systems for activating H2O2 in advanced oxidation processes. J Hazard Mater. 2014;275:121-35.
99. Yao M, Ma L, Li L, et al. A new modality for cancer treatment-nanoparticle mediated microwave induced photodynamic therapy. J Biomed Nanotechnol. 2016;12:1835-51.
100. Chen J, Fan T, Xie Z, et al. Advances in nanomaterials for photodynamic therapy applications: status and challenges. Biomaterials. 2020;237:119827.
101. Nieto-Juarez JI, Pierzchła K, Sienkiewicz A, Kohn T. Inactivation of MS2 coliphage in Fenton and Fenton-like systems: role of transition metals, hydrogen peroxide and sunlight. Environ Sci Technol. 2010;44:3351-6.
102. Salazar R, Brillas E, Sirés I. Finding the best Fe2+/Cu2+ combination for the solar photoelectro-fenton treatment of simulated wastewater containing the industrial textile dye disperse blue 3. Appl Catal B Environ. 2012;115-6:107-16.
103. Speisky H, Gómez M, Carrasco-Pozo C, Pastene E, Lopez-Alarcón C, Olea-Azar C. Cu(I)-glutathione complex: a potential source of superoxide radicals generation. Bioorg Med Chem. 2008;16:6568-74.
104. Li T, Zhou J, Wang L, et al. Photo-fenton-like metal-protein self-assemblies as multifunctional tumor theranostic agent. Adv Healthc Mater. 2019;8:e1900192.
105. Liu Y, Wu J, Jin Y, et al. Copper(I) phosphide nanocrystals for in situ self-generation magnetic resonance imaging-guided photothermal-enhanced chemodynamic synergetic therapy resisting deep-seated tumor. Adv Funct Mater. 2019;29:1904678.
106. Wan H, Zhang Y, Zhang W, Zou H. Robust two-photon visualized nanocarrier with dual targeting ability for controlled chemo-photodynamic synergistic treatment of cancer. ACS Appl Mater Interfaces. 2015;7:9608-18.
107. Wang Z, Ma R, Yan L, Chen X, Zhu G. Combined chemotherapy and photodynamic therapy using a nanohybrid based on layered double hydroxides to conquer cisplatin resistance. Chem Commun. 2015;51:11587-90.
108. Su X, Wang WJ, Cao Q, et al. A carbonic anhydrase IX (CAIX)-anchored rhenium(I) photosensitizer evokes pyroptosis for enhanced anti-tumor immunity. Angew Chem Int Ed. 2022;61:e202115800.
109. Wang M, Wu M, Liu X, et al. Pyroptosis remodeling tumor microenvironment to enhance pancreatic cancer immunotherapy driven by membrane anchoring photosensitizer. Adv Sci. 2022;9:e2202914.
110. Guo W, Li Z, Huang H, et al. VB12-sericin-PBLG-IR780 nanomicelles for programming cell pyroptosis via photothermal (PTT)/photodynamic (PDT) effect-induced mitochondrial DNA (mitoDNA) oxidative damage. ACS Appl Mater Interfaces. 2022;14:17008-21.
111. Lu Y, Xu F, Wang Y, et al. Cancer immunogenic cell death via photo-pyroptosis with light-sensitive Indoleamine 2,3-dioxygenase inhibitor conjugate. Biomaterials. 2021;278:121167.
113. Liu X, Bao X, Hu M, et al. Inhibition of PCSK9 potentiates immune checkpoint therapy for cancer. Nature. 2020;588:693-8.
114. Yang B, Gao J, Pei Q, Xu H, Yu H. Engineering prodrug nanomedicine for cancer immunotherapy. Adv Sci. 2020;7:2002365.
115. Chen Q, Chen M, Liu Z. Local biomaterials-assisted cancer immunotherapy to trigger systemic antitumor responses. Chem Soc Rev. 2019;48:5506-26.
117. Smyth MJ, Ngiow SF, Ribas A, Teng MW. Combination cancer immunotherapies tailored to the tumour microenvironment. Nat Rev Clin Oncol. 2016;13:143-58.
118. Kroemer G, Galassi C, Zitvogel L, Galluzzi L. Immunogenic cell stress and death. Nat Immunol. 2022;23:487-500.
119. Zhang Z, Zhang Y, Xia S, et al. Gasdermin E suppresses tumour growth by activating anti-tumour immunity. Nature. 2020;579:415-20.
120. Galluzzi L, Buqué A, Kepp O, Zitvogel L, Kroemer G. Immunogenic cell death in cancer and infectious disease. Nat Rev Immunol. 2017;17:97-111.
121. Xiong H, Ma X, Wang X, et al. Inspired epigenetic modulation synergy with adenosine inhibition elicits pyroptosis and potentiates cancer immunotherapy. Adv Funct Mater. 2021;31:2100007.
122. Han X, Cheng K, Xu Y, et al. Modularly designed peptide nanoprodrug augments antitumor immunity of PD-L1 checkpoint blockade by targeting indoleamine 2,3-dioxygenase. J Am Chem Soc. 2020;142:2490-6.
123. Cheng K, Ding Y, Zhao Y, et al. Sequentially responsive therapeutic peptide assembling nanoparticles for dual-targeted cancer immunotherapy. Nano Lett. 2018;18:3250-8.
124. Zhang Q, Guo X, Cheng Y, et al. Use of copper-cysteamine nanoparticles to simultaneously enable radiotherapy, oxidative therapy and immunotherapy for melanoma treatment. Signal Transduct Target Ther. 2020;5:58.
125. Wan D, Yang Y, Liu Y, et al. Sequential depletion of myeloid-derived suppressor cells and tumor cells with a dual-pH-sensitive conjugated micelle system for cancer chemoimmunotherapy. J Control Release. 2020;317:43-56.
126. Qiu W, Su W, Xu J, et al. Immunomodulatory-photodynamic nanostimulators for invoking pyroptosis to augment tumor immunotherapy. Adv Healthc Mater. 2022;11:e2201233.
127. Zhou F, Wu S, Yuan Y, Chen WR, Xing D. Mitochondria-targeting photoacoustic therapy using single-walled carbon nanotubes. Small. 2012;8:1543-50.
128. Chen H, Wan Y, Cui X, Li S, Lee CS. Recent advances in hypoxia-overcoming strategy of aggregation-induced emission photosensitizers for efficient photodynamic therapy. Adv Healthc Mater. 2021;10:e2101607.
129. Zhang R, Zeng Q, Li X, Xing D, Zhang T. Versatile gadolinium(III)-phthalocyaninate photoagent for MR/PA imaging-guided parallel photocavitation and photodynamic oxidation at single-laser irradiation. Biomaterials. 2021;275:120993.
130. Yang M, Yang T, Mao C. Enhancement of photodynamic cancer therapy by physical and chemical factors. Angew Chem Int Ed. 2019;58:14066-80.
131. Zhou H, Liu Z, Zhang Z, et al. Copper-cysteamine nanoparticle-mediated microwave dynamic therapy improves cancer treatment with induction of ferroptosis. Bioact Mater. 2023;24:322-30.
132. Wu Q, Xia N, Long D, et al. Dual-functional supernanoparticles with microwave dynamic therapy and microwave thermal therapy. Nano Lett. 2019;19:5277-86.
133. Ma L, Chen W, Schatte G, et al. A new Cu-cysteamine complex: structure and optical properties. J Mater Chem C. 2014;2:4239-46.
134. Huang X, Wan F, Ma L, et al. Investigation of copper-cysteamine nanoparticles as a new photosensitizer for anti-hepatocellular carcinoma. Cancer Biol Ther. 2019;20:812-25.
135. Pandey NK, Chudal L, Phan J, et al. A facile method for the synthesis of copper-cysteamine nanoparticles and study of ROS production for cancer treatment. J Mater Chem B. 2019;7:6630-42.
136. Wang P, Wang X, Ma L, et al. Nanosonosensitization by using copper-cysteamine nanoparticles augmented sonodynamic cancer treatment. Part Part Syst Charact. 2018;35:1700378.
137. Ma L, Zou X, Chen W. A new X-ray activated nanoparticle photosensitizer for cancer treatment. J Biomed Nanotechnol. 2014;10:1501-8.
138. Shrestha S, Wu J, Sah B, et al. X-ray induced photodynamic therapy with copper-cysteamine nanoparticles in mice tumors. Proc Natl Acad Sci USA. 2019;116:16823-8.
139. Shi L, Liu P, Wu J, et al. The effectiveness and safety of X-PDT for cutaneous squamous cell carcinoma and melanoma. Nanomedicine. 2019;14:2027-43.
140. Chong LM, Tng DJH, Tan LLY, Chua MLK, Zhang Y. Recent advances in radiation therapy and photodynamic therapy. Appl Phys Rev. 2021;8:041322.
141. Sah B, Wu J, Vanasse A, et al. Effects of nanoparticle size and radiation energy on copper-cysteamine nanoparticles for X-ray induced photodynamic therapy. Nanomaterials. 2020;10:1087.
142. Chen X, Liu J, Li Y, et al. Study of copper-cysteamine based X-ray induced photodynamic therapy and its effects on cancer cell proliferation and migration in a clinical mimic setting. Bioact Mater. 2022;7:504-14.
143. Gawande MB, Goswami A, Felpin FX, et al. Cu and Cu-based nanoparticles: synthesis and applications in catalysis. Chem Rev. 2016;116:3722-811.
144. Wu T, Liang X, Liu X, et al. Induction of ferroptosis in response to graphene quantum dots through mitochondrial oxidative stress in microglia. Part Fibre Toxicol. 2020;17:30.
145. Chu X, Li K, Guo H, et al. Exploration of graphitic-C3N4 quantum dots for microwave-induced photodynamic therapy. ACS Biomater Sci Eng. 2017;3:1836-44.
146. Pandey NK, Xiong W, Wang L, et al. Aggregation-induced emission luminogens for highly effective microwave dynamic therapy. Bioact Mater. 2022;7:112-25.
147. Chu X, Mao L, Johnson O, et al. Exploration of TiO2 nanoparticle mediated microdynamic therapy on cancer treatment. Nanomedicine. 2019;18:272-81.
148. Wang S, Tian R, Zhang X, et al. Beyond photo: xdynamic therapies in fighting cancer. Adv Mater. 2021;33:e2007488.
149. Allen C, Her S, Jaffray DA. Radiotherapy for cancer: present and future. Adv Drug Deliv Rev. 2017;109:1-2.
150. Srinivas US, Tan BWQ, Vellayappan BA, Jeyasekharan AD. ROS and the DNA damage response in cancer. Redox Biol. 2019;25:101084.
151. Chen W, Zhang J. Using nanoparticles to enable simultaneous radiation and photodynamic therapies for cancer treatment. J Nanosci Nanotechnol. 2006;6:1159-66.
152. Chen W. Nanoparticle fluorescence based technology for biological applications. J Nanosci Nanotechnol. 2008;8:1019-51.
153. Zou X, Yao M, Ma L, et al. X-ray-induced nanoparticle-based photodynamic therapy of cancer. Nanomedicine. 2014;9:2339-51.
154. Liu Z, Xiong L, Ouyang G, et al. Investigation of copper cysteamine nanoparticles as a new type of radiosensitiers for colorectal carcinoma treatment. Sci Rep. 2017;7:9290.
155. Liu F, Chen W, Wang SP, Joly AG. Investigation of water-soluble X-ray luminescence nanoparticles for photodynamic activation. Appl Phys Lett. 2008;92:43901.
156. Homayoni H, Sahi S, Ma L, et al. X-ray excited luminescence and persistent luminescence of Sr2MgSi2O7:Eu2+, Dy3+ and their associations with synthesis conditions. J Lumin. 2018;198:132-7.
157. Ma L, Zou X, Chen W. A new X-ray activated nanoparticle photosensitizer for cancer treatment. J Biomed Nanotechnol. 2014;10:1501-8.
158. Chen X, Liu J, Li Y, et al. Study of copper-cysteamine based X-ray induced photodynamic therapy and its effects on cancer cell proliferation and migration in a clinical mimic setting. Bioact Mater. 2022;7:504-14.
159. Shrestha S, Wu J, Sah B, et al. X-ray induced photodynamic therapy with copper-cysteamine nanoparticles in mice tumors. Proc Natl Acad Sci USA. 2019;116:16823-8.
160. Wang J, Hao L, Li X, Liu S. W18O49@EP nanoparticles improve the anti-tumor effect of radiotherapy and photodynamic therapy by avoiding the limitation of hypoxia. Front Bioeng Biotechnol. 2022;10:1060467.
161. Gong L, Zhang Y, Zhao J, et al. All-in-one biomimetic nanoplatform based on hollow polydopamine nanoparticles for synergistically enhanced radiotherapy of colon cancer. Small. 2022;18:2205198.
Cite This Article
How to Cite
Download Citation
Export Citation File:
Type of Import
Tips on Downloading Citation
Citation Manager File Format
Type of Import
Direct Import: When the Direct Import option is selected (the default state), a dialogue box will give you the option to Save or Open the downloaded citation data. Choosing Open will either launch your citation manager or give you a choice of applications with which to use the metadata. The Save option saves the file locally for later use.
Indirect Import: When the Indirect Import option is selected, the metadata is displayed and may be copied and pasted as needed.
About This Article
Copyright
Data & Comments
Data
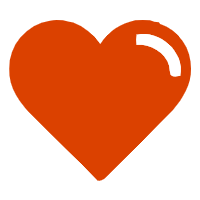
Comments
Comments must be written in English. Spam, offensive content, impersonation, and private information will not be permitted. If any comment is reported and identified as inappropriate content by OAE staff, the comment will be removed without notice. If you have any queries or need any help, please contact us at [email protected].