Crosstalk between cell fate and survival pathways during uterine cervical carcinoma progression: a molecular and clinical perspective
Abstract
The development of uterine cervical cancer is primarily attributed to infection by high-risk human papillomaviruses (HR-HPVs). E5, E6 and E7, the three early oncoproteins of HR-HPVs, have been implicated in initiation and progression of cervical cancer. The intricate molecular mechanisms that orchestrate aberrant cellular transformations to establish carcinoma of the cervical epithelium following viral infections are poorly understood. Here, we discuss how deregulation of three major cell fate regulatory pathways, Hedgehog, Wnt and Notch, and cell survival strategies involving EGFR signaling and G1/S checkpoint contribute towards cervical cancer development and progression. Further exploration of protein interaction database has revealed several genes that are involved in cervical cancer initiation and progression, and the two crucial "driver” genes, MYC and CTNNB1
Keywords
INTRODUCTION
Uterine cervical cancer is a type of gynecological cancer that is virtually preventable by implementing highly effective primary (HPV vaccine) and secondary (Papanicolaou screening test) preventive measures. Despite significant improvements, these measures have not been appropriately implemented across all countries. As a result, cervical cancer is still the fourth most frequently diagnosed cancer amongst women worldwide, ranking only after breast, lung, and colorectal cancer[1]. In 2020, an estimated 604,127 new cases of cervical cancer (3.1% of all cancers) were reported globally, while approximately 341,831 deaths (3.3% of all cancers) ranked it as the fourth leading cause of cancer-associated death in women[1] [IARC Cervix uteri, source: Globocan 2020, Available from: https://gco.iarc.fr/]. Age-related cervical cancer incidence varied widely between countries and was estimated at 13.1 per 100,000 women worldwide. Globally, the average age at diagnosis of cervical cancer and death was 53 and 59 years, respectively[2]. In thirty-six countries, it is the top-ranked leading cause of cancer-related death, and the most commonly diagnosed female cancer in twenty-three underdeveloped or developing countries in sub-Saharan Africa, Melanesia, South America, and South-Eastern Asia including India[1].
Twelve oncogenic human papillomaviruses (HPV16, 18, 31, 33, 35, 39, 45, 51, 52, 56, 58 and 59), designated as high-risk human papillomavirus (HR-HPV), are classified as group 1 carcinogens by the International Agency for Research on Cancer Monographs and are essential but not enough cause for cervical cancer development[3]. Other significant associated factors include sexually transmittable infections (Human immunodeficiency virus type 1 and Chlamydia trachomatis), exposure to diethylstilbestrol (in utero), tobacco smoking, a high parity, and long-term use of estrogen–progestogen (combined) oral contraceptives[1]. Some recent studies evaluated the possible connections between the vaginal microbiome (VMB) and cervical cancer. The presence of a large number of anaerobic bacterial species such as Gardnerella vaginalis, Sneathia, Megasphaera, and Prevotella in vaginal microbiota is also associated with cervical cancer development[4].
Apart from all the above etiological and epidemiological factors, different molecular, cellular and signaling pathways and their deregulations are directly associated with the development of cervical cancer. In 2013, Vogelstein et al. developed a generalized theory of tumorigenesis by categorizing all the driver genes into twelve cellular pathways that regulate three core cellular processes: cell fate, cell survival, and genome maintenance[5]. Various genetic and epigenetic alterations of these drivers in relation to the development of cervical cancer have also been extensively studied. In this review, we have attempted to understand the association of cell fate and cell survival regulatory pathways and their crosstalk in the initiation and progression of cervical cancer.
CELL FATE REGULATORY PATHWAYS AND THEIR ASSOCIATION WITH CERVICAL CANCER DEVELOPMENT
Several genetic alterations during cancer development perturb the balance between differentiation and proliferation, favoring the latter. This causes a selective growth advantage as differentiating cells eventually die or become quiescent and alters the cell fate program during tumor progression[5]. Pathways that function through this mechanism include Hedgehog, Wnt/APC/β-catenin and Notch signaling cascades. All these pathways are well-studied self-renewal pathways that, through asymmetric cell division, maintain proper stemness and differentiation status of the cell and control mammalian cellular fate[6,7].
Persistent infection by HR-HPVs in the cervical epithelial basal layer disrupts the otherwise normal homeostasis of cervix[8]. Diverse molecular and dynamic communications among the various cells and their microenvironment are essential for maintaining this normal homeostasis[9,10]. The homeostasis is disrupted by carcinogenesis that involves aberrant cellular transformations through sustained perturbations of genomic and epigenomic landscapes, generating tumors comprising heterogeneous cell populations. Such tumors negatively impact the local homeostasis to fulfil their metabolic demands for growth and uncontrolled proliferation, eventually escaping the immune surveillance to invade or metastasize, initiating secondary tumors in distal tissues and organs, thus affecting systemic homeostasis and overall normal physiology[11,12]. Tumor malignancy induces homeostatic distress primarily through the release of a wide array of cytokines, neurohormonal and immune mediators, affecting body and brain functions[12]. Normal cervical epithelial homeostasis is maintained through the precise balance between basal cell proliferation, which increases cell density, and basal layer delamination and epithelial differentiation, which reduces basal cell density. After HR-HPV infection, enhanced basal cell proliferation followed by increased cell density disrupts the normal regulation of epithelial delamination and differentiation, establishing early stages of cervical cancer[8]. During this development, HR-HPVs first establish their infection through the cervical transformation zone (TZ), which comprises ectocervix, squamocolumnar junction, reserve cells and endocervical crypts. Then, damage to the ectocervix, a multilayered stratified squamous epithelium, enables HR-HPVs to reach the basal stem cells[8]. This passage leads to the expression of diverse genes to avoid immune surveillance and favors the permanent establishment of viruses in the basal layer. E6, an HR-HPV oncoprotein, is associated with the deregulation of normal cervical epithelial homeostasis and initial perturbation of the differentiation program, which is otherwise tightly regulated by different stem cell regulatory pathways involving Wnt and Notch signaling systems[8,13]. Deregulation of these pathways by genetic and epigenetic instabilities also disrupts normal cellular differentiation, leading to the erosion of barriers against dedifferentiation[7]. This phenomenon eventually generates cancer stem cells (CSCs) and tumor cell heterogeneity that contribute to the development and progression of cervical cancer[14].
Hedgehog signaling
Hedgehog (HH) signaling is one of the major self-renewal pathways during early embryogenesis. Canonical HH signaling pathway consists of three ligands, Indian hedgehog (IHH), Desert hedgehog (DHH) and Sonic hedgehog (SHH), a 12 transmembrane receptor called Patched 1 (PTCH1), a class F G protein-coupled receptor (GPCR) as well as signal transducer called Smoothened (SMO), a cytoplasmic protein named SUFU and three transcription factors (GLI1, GLI2 and GLI3)[15]. In the absence of ligands, GLI2/3 are attached to the SUFU by KIF7. Glycogen synthase kinase (GSK-3β), protein kinase A (PKA) and CK1α then phosphorylate GLI2/3, a phenomenon triggered by cyclic AMP but initiated by G protein-coupled receptor 161 (Gpr161). As a result, Cullin 1 (CUL1) and β transducin repeat-containing protein (β-TrCP) remove the C-terminal transcriptional activation domain of GLI2/3, converting them into repressors (GLI2R and GLI3R) [Figure 1A][16]. However, binding of ligands to the receptors and coreceptors initiates HH signaling cascade that promotes degradation of SUFU and translocation of activated GLI (GLIA), either full-length GLI1 or truncated GLI2/3 into the nucleus [Figure 1B][17,18]. GLI2 acts as the major activator to trigger the expression of GLI1, the principal effector molecule and a transcription factor, which leads to the hyper-activation of several target genes associated with cell differentiation, proliferation, cell cycle progression, apoptosis, tissue patterning, DNA damage, angiogenesis, vascularization and cell-cell adhesion[17,19]. All these signaling components and cellular processes are manipulated by tumor cells during carcinogenesis as well as metastasis[20].
Figure 1. Cell fate regulatory canonical Hedgehog, Wnt and Notch signaling pathways in cervical cancer. (A) "OFF STATE” describes the cytoplasmic stabilization of GSK3β followed by proteasomal degradation or cytoplasmic restriction of the effector molecules; GLIA,
Both upregulation and downregulation of HH pathway components are frequently associated with various types of cancer, including cervical cancer. Chaudary et al. observed elevated expression of SHH (89%), IHH (85%), GLI1 (79%) and SMO (33%) in 85 human cervical carcinoma samples[21]. Addition of SHH ligand is associated with cell proliferation and migration of cervical cancer cells, while inhibition of SMO and GLI reduced cell numbers significantly and induced apoptosis by upregulating pro-apoptotic protein cleaved caspase 3[22]. Immunohistochemical analysis of 102 human uterine cervical cancer samples showed that reduced expression of PTCH1 was concordant with its genetic and epigenetic alterations due to deletion/promoter methylation during cervical cancer development[23]. The same study also correlated the high nuclear expression of GLI1 with reduced PTCH1 expression[23]. The landscape of genomic alterations in cervical carcinomas revealed an association of high expression of GLI2 with its copy-number gain[24]. In the presence of HPVE6, an elevated expression of SMO/GLI and loss of negative regulator SUFU further suggested a possible crosstalk between this etiological factor and signaling pathway, which facilitates cancer progression[25]. A recent study reported that SUFU is also involved in the suppression of tumor cell migration and DNA damage repair by inhibiting the activity of a transcriptional factor, ZNF281[26].
Notch signaling
Notch signaling is another pathway associated with cell division, differentiation, and survival[27]. Deregulation of both canonical and non-canonical Notch signaling plays diverse essential roles during cervical cancer progression. In canonical Notch pathway, transmembrane Notch receptors NOTCH1, NOTCH2, NOTCH3, and NOTCH4 of one cell interact with DSL (Delta, Serrate, and Lag2) ligands (Jagged 1, 2 and Delta-like 1, 3 and 4) present in the adjacent cells [Figure 1A][28]. This interaction triggers two sequential proteolytic cleavages in the NOTCH receptor. The first cleavage is a type of S2 cleavage, which is catalyzed by ADAM10 or TACE (TNF-α-converting enzyme; also known as ADAM17) metalloproteases to produce a substrate for S3 cleavage. This second cleavage is mediated by γ-secretase, an enzyme complex that contains presenilin, nicastrin, PEN2 and APH1, and releases active Notch intracellular domain (NICD)[28,29]. Subsequently, NICD is transported to the nucleus and heterodimerizes with DNA binding protein RBP-J (recombination signal sequence-binding protein Jκ), also known as CSL (CBF1, Su(H) and
Notch pathway was previously reported for its differentiation-promoting role during cervical cancer development from normal cervical epithelium[32]. This pathway plays both oncogenic and tumor suppressor functions in cervical cancers. In HPV16-induced primary human keratinocytes, activated Notch1 cooperates with HPV16 oncogenes E6 and E7, and promotes cellular transformation[33]. The RT-PCR analysis of over-expressed Notch1/JAGD1 revealed its association with tumor progression in human cervical cancer and poor patient survival[34]. A recent study further supported this proliferation of cervical cancer cells through DARS-AS1/miR-628-5p/JAG1 axis that activates Notch signaling pathway[35]. Both NOTCH1 mRNA and protein overexpression in cervical cancer cells were involved in tumorigenesis and suppression of apoptosis[36]. This pathway also facilitates anoikis resistance followed by cell migration and epithelial-to-mesenchymal transition when transfected with E6/E7 in an Akt-dependent manner[37,38]. The Cancer Genome Atlas (TCGA) project found that 15% of cases of primary squamous cell cervical carcinoma were associated with the loss of function alteration (deep deletion, truncating mutation, missense mutation) of FBXW7, a negative regulator of Notch pathway[24]. TCGA data also revealed copy number variations (CNVs) of NOTCH target genes, including HES1, CCND1, and Myc[24,27]. Overexpression of both HES1 and HES5 was associated with late-stage cervical cancer cases, but the poor prognosis was also observed in patients with early-stage cervical cancer[39]. NOTCH1 signaling modulates tumorigenic properties in cervical cancer cells through RhoC[40]. During its tumor suppressor functions, decreased NOTCH1 expression was found to be correlated with an increase in NUMB expression in cervical cancer compared to cervical intraepithelial neoplasia[41]. In vitro transient overexpression of Intracellular Notch (ICN1, 2, 3, 4) was associated with decreased cell proliferation[42]. A recent study revealed that low mRNA expressions of NOTCH1 and NOTCH3 were concordant with their high promoter methylation (92% and 61%, respectively)[43]. Further, Rodrigues et al. showed that meta-analysis of TCGA data was consistent with altered expression of Notch pathway components in > 80% of primary cervical cancer samples[27].
Wnt signaling
During embryonic development, critical regulation of cellular physiology involving proliferation, differentiation, adhesion, and polarity is associated with Wnt signaling pathway[44]. Depending on the particular combination of receptor and ligand, Wnt pathways are of three types: canonical, non-canonical planar cell polarity (PCP) pathway and non-canonical Wnt/calcium pathway. In canonical pathway, canonical Wnt ligands (Wnt1, Wnt2, Wnt3, Wnt3A, Wnt7 and Wnt8) bind to canonical Frizzled receptors (FZD1-5, FZD7-8 and FZD10) as well as LRP5/6 coreceptors (low-density lipoprotein receptor-related protein 5/6). These initiate intracellular signaling by sequestering the β-catenin degradation complex composed of the tumor suppressor adenomatous polyposis coli (APC), the scaffolding protein Axin, two kinases: CK1α (casein kinase 1α), GSK3β (glycogen synthase kinase 3β), and a phosphatase Protein phosphatase 2A (PP2A)[40]. This event helps in the stabilization of β-catenin in the cytoplasm by sequential phosphorylation at the
Aberrant canonical Wnt signaling pathway contributes as a key player in stimulating the clonal selection of cancer stem cells (CSCs), and initiation, maintenance and development of various cancers, including cervical cancer[44,47]. Aberrant activation of Wnt pathway has been suggested as the second hit in HPV-immortalized human keratinocyte transformation during cervical cancer development[48]. Increased nuclear β-catenin expression is frequent in cervical cancer patients, whereas β-catenin mutation was a rare event here[48,49]. Frequent promoter hypermethylation of SFRP1, SFRP2, SFRP4, and SFRP5 during cervical cancer development were reported by different studies[50,51]. Ectopic expression of SFRP5 was involved in low expression of Wnt/beta-catenin downstream genes that suppressed colony formation and invasion of cervical cancer cells in vitro[51]. Rescued expression of SFRP1 and SFRP2 in CaSki cells suppressed nuclear accumulation of beta-catenin and reduced cancer cell growth. The same study also revealed that ectopic expressions of both proteins were associated with epithelial marker E-cadherin overexpression, and lower expression of SLUG, TWIST and SNAIL, the three musketeers of EMT[52]. Promoter of another negative regulator DKK1 was also hypermethylated, and its low expression during cervical cancer development is inversely correlated to the high TWIST expression[53,54]. AXIN and APC, two other critical components of β-catenin destruction complex, were rich in CpG islands in their promoters and were found to be methylated in cervical cancer[53]. Upstream activation of the Wnt/β-catenin pathway also resulted from overexpression of pathway activators such as Wnt ligands (WNT6, WNT10B and WNT14), frizzled receptors (FZD7. FZD10), and dishevelled (DVL1) in cervical cell lines[55-59]. A study also revealed that low expression of APC is associated with its deletion or promoter hypermethylation during the development of uterine cervical cancer. This study also revealed that overexpression of WNT3 and medium to high (74%-95%) expression of β-catenin/p-β-catenin (Y654) were related to the poor overall survival of patients[60]. Recently, it was reported that GSK3β was transcriptionally repressed by DAX1[61]. As a result, reduced proteasomal degradation, as well as increased nuclear accumulation of β-catenin, was observed. This phenomenon additionally promoted cell growth, tumorigenicity, and tumorsphere formation through activation of the Wnt/β-catenin pathway[61]. Further, TCGA data showed that 83% of all cervical cancer samples showed at least one mutation within the Wnt pathway components[62]. Feng et al. reported LGR6-mediated activation of Wnt/β-catenin pathway through the overexpression of TCF7L2 leading to β-catenin/TCF7L2/LGR6 feedback loop in LGR6high cervical cancer stem cells[63]. A recent Gene set enrichment analysis (GSEA) confirmed the interaction between OTX1 and Wnt9b[64]. This study showed that OTX1 silencing inhibited the overexpression of the Wnt antagonists such as APC, GSK3β and AXIN2 and increased levels of Wnt9b and β-catenin during cervical cancer progression[64].
CELL SURVIVAL REGULATORY PATHWAYS AND THEIR ASSOCIATION WITH CERVICAL CANCER DEVELOPMENT
Cell cycle checkpoints, especially G1/S checkpoint, and EGFR signaling pathway are the two interesting aspects of cell survival regulation. Both were reported to be involved in the development of diverse human cancers, including cervical cancer. Two important tumor suppressor genes (TSGs) RB1 and p53, also the guardians of cell cycle, control the G1/S checkpoint in normal cells [Figure 2][65]. HR-HPV infection is the most important etiological factor in cervical cancer development. The two main oncoproteins of HR-HPV, E6 and E7, play a crucial role in the progression of this disease[66]. E6 and E7 can deregulate all the major hallmarks of cancer and can achieve uncontrolled cell growth and proliferation by deactivating growth suppressors[67]. In HR-HPV-infected cells, E6 and E7 interact with p53 and RB1, respectively, and inhibit their normal tumor-suppressing activities [Figure 2][66]. HR-HPV E6 first interacts with host E6-associated protein (E6-AP), an E3 ubiquitin ligase, through their conserved LXXLL motif. This pair again forms a heterotrimeric complex with p53 (E6/E6-AP/p53 complex) that ultimately leads to the proteasomal degradation of p53, thereby preventing p53-mediated apoptosis[66,67]. E6 also inhibits apoptosis and increases chromosomal instability through the degradation of pro-apoptotic proteins, BAX and BAK[66]. This oncoprotein is further associated with the activation of reverse transcriptase telomerase (hTERT) that prevents telomere shortening, contributes to persistent proliferation and promotes immortalization[66,67]. E6 oncoprotein also inhibits the degradation of SRC-family kinases that are involved in the growth stimulation of HR-HPV-infected cells[66].
Figure 2. Cell survival regulatory pathways in cervical cancer. (A) In healthy cervical epithelial cells, RB1 normally associates with LIMD1 to sequester E2F during early G1 phase. Initially, CCND1/D2/D3-CDK4/6 complex phosphorylates RB1, which is further phosphorylated by CCNE1-CDK2 complex in late G1. Free E2F then only impacts the expression of cell cycle regulatory genes. Cyclin-CDK complexes are also negatively controlled by p15, p16, p21 (positively regulated by p53) and p27. EGFR positively regulates the CCND’s expression but in a controlled manner. Phosphorylated RB1, also dephosphorylated by RBSP3, further complexes with E2F, resulting in normal control of cell cycle progression. (B) In the presence of HR-HPV oncoproteins, E5, E6 and E7, normally functioning cellular systems are completely jeopardized. E5 gets involved in constitutive activation of EGFR that helps in overexpression of CCNDs. On the other hand, E6 and E7 are associated with the degradation of p53 and RB1, respectively. E7 also deregulates p15, p16, p21 and p27. LIMD1 and RBSP3 are inactivated by genetic and/or epigenetic alterations. CCND1/D2/D3-CDK4/6 and CCNE1-CDK2 complexes constitutively phosphorylate RB1. Thus, E2F is free to transcribe cell cycle regulators abnormally, and the cell cycle loses its brake. Created with BioRender.com.
In HR-HPV-infected cells, another oncoprotein, E7, is involved in the inactivation of RB1 that controls
Apart from this mechanism, RB1 was downregulated through its deletion and/or promoter methylation during cervical cancer progression[68]. The same study also showed that alterations (deletion/promoter methylation) of RBSP3 were associated with overexpression of phosphorylated-RB1 (p-RB1) in cervical cancer tumor samples and poor prognosis of patients[68]. A tumor suppressor candidate, LIMD1, was associated with RB1 and inhibited the growth and metastases of cancer cells[69]. Both RBSP3 and LIMD1 are associated with the unphosphorylated state of RB1 and the negative regulator of cell cycle progression [Figure 2]. Chakraborty et al. showed that reduced protein expression of LIMD1 was associated with progression of cervical cancer development and correlated to its deletion/promoter methylation status[70].
EGFR is frequently overexpressed in cervical dysplasia and cancers[71]. Immunohistochemical analyses in 53 primary cervical cancers showed overexpression (64%) of EGFR protein that corresponded with lymph node metastasis[72]. EGFR amplification might be critical for cervical squamous cell carcinoma progression and strongly correlated with clinical parameters in addition to protein expression[73]. However, in cervical cancer, EGFR activating mutations were uncommon[73,74]. TCGA data analysis identified novel recurrent focal amplification (17%) at EGFR locus (7p11.2)[62]. High HPV16 E6 mRNA expression was associated with elevated membrane expression of EGFR[75]. It was also suggested that another less explored HPV oncoprotein, E5, immediately interacted with EGFR post-infection, and activated the downstream Ras-Raf-MAP kinase pathway or PI3K-Akt pathway that led to altered cell proliferation, angiogenesis, and apoptotic signaling in cervical cancer cells[76,77]. A recent study established a feedback loop between the JNK-dependent activation of EGFR signaling pathway and HPV E6/E7 expression that promotes cell proliferation, survival and EMT[78].
In Figure 3, we have summarized the cellular and molecular mechanisms that aberrantly transform the normal cervical epithelium to initiate cervical cancer. Stabilization of membrane EGFR by E5 oncoprotein of HR-HPVs along with degradation of p53 and RB1 by E6 and E7 oncoproteins of HR-HPVs, respectively, are involved in cell cycle deregulation. On the other hand, genetic and epigenetic alterations of different cell fate regulatory genes and/or proteins stabilize their effector molecules in the cell nuclei. These transcription factors then drive the expression of different genes, which further control cell survival strategies and deregulate cell cycle progression [Figure 3]. Together, these major events are critically linked to uterine cervical cancer initiation and progression.
Figure 3. Summary of deregulation of cell fate and cell survival regulatory pathways under the influence of HR-HPVs during cervical cancer initiation and development. The cell regulatory systems are generally destabilized by HR-HPV oncoproteins, while the cell fate regulators are disrupted primarily by genetic and/or epigenetic alterations. Both systems cooperate to aberrantly transform healthy cervical cells and deregulate cell cycle progression. Created with BioRender.com.
HOW DO THESE PATHWAYS CROSSTALK?
The information we have gathered until now tells us about cell cycle progression through deregulation of cell fate and cell survival strategies adopted by tumor cells. However, how easily can they maintain this relationship amidst the complex milieu of diverse cellular functions? Ongoing research is behind this Holy Grail.
Hedgehog, Notch and Wnt pathways act through their effector molecule(s), GLI1/2/3, RBPJ, also known as CSL (CBF1, Su(H) and LAG-1) and β-catenin (CTNNB1), respectively. Functionally, all these effectors are transcription factor(s) and they work with other transcriptional co-factors to transcribe their downstream genes. These sets of genes drive cell proliferation and growth progression. The Hedgehog pathway effector(s), GLI proteins, can directly bind to the promoter of its target genes, regulating their expression[79]. GLI1 and GLI3 proteins recognize the 5'-GACCACCCA-3' sequence, while GLI2 binds at the 5'-GAACCACCCA-3' sequence[79]. The major target genes of Hh signaling pathway include PTCH1, PTCH2, and GLI1 itself; while others are HHIP, proliferation-promoting genes (CCND1 and MYC), cell cycle regulators (CCND2, CCNE1), apoptosis regulator (BCL2), epithelial-to-mesenchymal transition genes (SNAIL), stem cell self-renewal (NANOG and SOX2), Notch pathway ligand (JAG1), and different members of Wnt signaling pathway[79,80]. The target genes for canonical Notch signaling are largely governed by the DNA binding Su (H) motif of CSL (RBPJ), and recognize the consensus 5'-CGTGGGAA-3'[81,82]. A nuclear complex consisting of CSL, NICD, Mastermind, p300 and HAT induces the transcriptional activation of downstream targets of Notch pathway that include HES1, HES5, HES7, HEY1, HEY2 and HEYL[83]. Additionally, MYC, CCND1 and p21/Waf1 are also targets of Notch signaling pathway[29]. Activation of the canonical Wnt pathway during the development of cancer leads to nuclear translocation of Y654-p β-catenin. There it interacts with TCF/LEF, its co-transcriptional activator, that binds to 5'-CCTTTGATC-3' sequence and transcribes target genes (PDK1, MTC1, MYC, CCND1, COX2, AXIN2, EGFR and CD44)[84]. Nuclear β-catenin further interacts with NICD and controls the expression of Notch ligand genes, including DLL1, DLL3, DLL4, JAG1 and JAG2, due to the presence of TCF/LEF-binding site within the promoter region of these genes[83].
Activation of Hedgehog and Wnt signaling pathways accelerates nuclear accumulation of GLIs and β-catenin, respectively, while both pathways are connected by molecules such as GSK3β, CK1α, SUFU, p53, PTEN, SMO and KRAS[85]. Effector molecules GLIs and β-catenin are involved in the activation of Notch pathway ligands, cell cycle regulators and EGFR. GLI1 is involved in the transcription of G1/S cyclins (CCND1 and CCNE1)[80]. CCND1 is also a target gene of β-catenin[84]. Deregulated expression of these cyclins with their respective CDKs can influence aberrant cell cycle progression during tumorigenic development. RB1, along with LIMD1 and E2F, controls cell cycle progression through G1/S phase
The network
Using the STRING database, we predicted functional interactions among the cell fate and cell survival regulatory pathway proteins and constructed a protein-protein interaction (PPI) network based on both known and predicted PPIs [Figure 4][88]. Further, hub genes of the PPI network were identified using Cytoscape (version 3.9.1) with additional CytoHubba plug-in[89]. Nested network style and yFiles radial layout algorithm (yFiles Organic Layout and yFiles Remove Overlaps) were used to redraw the network [Figure 4]. We then calculated node degrees for each protein to determine the most influential proteins in the network. MYC, CTNNB1, GSK3B, SHH, GLI2, AXIN1, EGFR, GLI3, NOTCH1 and RBPJ were sequentially identified as the top 10 hub proteins using the Bottleneck method in this network [Figure 4][90]. Among these proteins, the genes encoding MYC, CTNNB1, AXIN1, EGFR and NOTCH1 proteins are classified as the potent "driver” genes, while the rest are "passengers”[5]. This observation suggests that hub proteins might play precise but critical roles in cervical cancer progression. Furthermore, these proteins might also be potential therapeutic targets in the future, being extracted by Bottleneck method[91]. Here, our discussion primarily focuses on the top three proteins in the analyzed network according to their connectivity levels.
Figure 4. Protein-protein interaction (PPI) network with known genes of cell fate and cell survival regulatory pathways. MYC, CTNNB1, GSK3B, SHH, GLI2, AXIN1, EGFR, GLI3, NOTCH1 and RBPJ are identified as the top 10 hub proteins using the Bottleneck method. Among these proteins, MYC, CTNNB1 and GSK3B appeared as the most connected hub proteins in the network. Color bar indicates high (red) to low (yellow) connectivity of the protein hubs.
DISCUSSION
From our analyses, MYC, CTNNB1 and GSK3β appeared as the most crucial and connected proteins in the network [Figure 4]. GSK3β is established as the first level of crosstalk molecule because of its involvement in regulating all three cell fate pathways. It is an unconventional serine/threonine protein kinase (STK) that generally requires pre-phosphorylated substrates for phosphorylation. Cytoplasmic stabilization of GSK3β is associated with the "OFF STATE” of Hedgehog, Notch and Wnt signaling systems [Figure 1A]. In this state, all the effector molecules of these pathways, GLI, NICD and CTNNB1, are trapped in the cytoplasm by their respective destruction complex and are unavailable for nuclear translocation. As a result, no downstream target genes of these pathways are expressed to regulate cellular fate. In the "ON STATE”, the signaling dynamics of the three pathways change completely [Figure 1B]. HR-HPVE5 induced EGFR/MAPK pathway phosphorylates GSK3β at its Serine9 position[92]. This phosphorylated GSK3β cannot hold back GLI, NICD and CTNNB1 in the cytoplasm further. As a result, nuclear translocation of these transcription factors induce the expression of their respective target genes, which initiate the next level of deregulation in cells. Thus, GSK3β appears as the "non-hub–bottleneck node” protein in this network as it is involved in connecting all the three major cell fate regulatory pathways [Figure 5].
Figure 5. Crosstalk between cell fate and cell survival pathways during cervical cancer development. GSK3β, CTNNB1 and MYC co-operatively mediate crosstalk between cell fate and cell survival regulatory mechanisms. GSK3β, a "non-hub-bottleneck node” protein in this network, acts as the crosstalk molecule between all three major cell fate regulatory pathways. MYC is the most connected protein and the "hub-bottleneck node” in this network, as it controls cell survival strategies along with cell cycle progression. CTNNB1, another "hub-bottleneck node” of this network, is the connector between GSK3β and MYC. Thus, GSK3β/CTNNB1/MYC regulatory axis concurrently controls cell fate and cell survival strategies during cervical cancer initiation, development and progression. Created with BioRender.com.
Among the three effector molecules, CTNNB1 or β-catenin established itself as the second-level hub protein in this network [Figure 4]. Even though GLI and NICD function as transcription factors of several target genes that are associated with deregulation of cell growth and survival, CTNNB1 is the key player. After phosphorylation of GSK3β at Serine9, dissociation from the E-cadherin (CDH1) pool and cytoplasmic stability of CTNNB1 increase due to Akt-mediated phosphorylation of β-catenin at S552[93]. Next, EGFR/Src mediated S333 and Y654 phosphorylation facilitates nuclear translocation of this protein. Finally, Protein kinase A (PKA) phosphorylates the C-terminal Serine675 that promotes transcriptional activity of β-catenin[93]. In the nucleus, phosphorylated (C-terminal) and hyperactivated CTNNB1 drives aberrant upregulation of CCND1, MYC, EGFR, MMP7, COX-2, CD44 and many epithelial-to-mesenchymal transition (EMT) related proteins[84,94]. Expression of these diverse proteins then sets a cascade of deregulation in cells. CCND1 and MYC give cells growth advantages positively supported by EGFR signaling cascade[95]. EFGR also provides a positive feedback loop to CTNNB1 for its nuclear translocation[93]. β-catenin is involved in the expression of SNAIL1, an essential EMT marker, overexpression of which is directly associated with loss of cell-cell junction as well as cell invasion and metastasis in cancer[96]. CTNNB1 can be projected as the junctional hub or the first "hub-bottleneck node” protein as it is controlled under the cell fate pathways and, on the other hand, has the ability to control different regulatory proteins associated with diverse cellular processes, including cell growth and differentiation during cancer development [Figure 5].
The highest level of connectivity was observed through MYC protein in this network [Figure 4]. MYC has been identified as the target protein of effector molecules of all three cell fate regulatory pathways[79,80,84,97]. MYC, acting as a transcription factor, regulates ~15% of all genes involved in diverse cellular mechanisms, including cellular proliferation and differentiation, and cell cycle progression[98,99]. Deregulation of MYC is reported in ~70% of all human cancers and its aberrant expression is directly correlated with tumor initiation and maintenance[99]. Further, this protein has the ability to regulate all the hallmarks of cancer[99]. MYC tethers with MYC-associated factor X (Max) and binds to E-boxes with the consensus (sometimes non-consensus) core sequence 5'-CACA/GTG-3' and impacts transcriptional activation of several target genes involved in cell cycle regulation[100]. The major target proteins in this category are CCND1, CCND2, CCND3, CCNE1, CCNE2, CCNA2, CCNB1, CDK1, CDK2, CDK4, CDK6, CDK7 (a subunit of cyclin-dependent kinase (CDK) activating kinase (CAK)), CDC25A and E2F1[101].
This clearly indicates that MYC controls the expression of all the major cell cycle regulators. Sequential phosphorylation of RB1 by various cyclin-CDK complexes throughout different phases of cell cycle allows the activation and accumulation of E2F transcription factors[87,101,102]. This phenomenon induces the expression of S phase-related proteins that are involved in DNA replication[101]. Finally, MYC-regulated CCNB1/B2-CDK1 complex helps the cells during the M-phase transition [Figure 5][102-104]. MYC also functions as a transcriptional repressor while binding with MYC-interacting zinc finger protein-1
CONCLUDING REMARKS AND FUTURE DIRECTIONS
From the above discussion, it is clear that cell fate and regulatory pathways work together via the three important crosstalk molecules, GSK3β, CTNNB1 and MYC. These three proteins are involved through a common axis to regulate cells’ fate followed by their survival [Figure 5]. GSK3β can directly regulate the nuclear translocation of CTNNB1 as well as CTNNB1-dependent expression of MYC[105]. On the other hand, GSK3β is also involved in the degradation of MYC by phosphorylating it at Threonine58[106,107]. MYC further stimulates cell cycle progression and proliferation through both overexpression and under-expression of positive and negative regulators of cell cycle, respectively [Figure 5]. GSK3β, CTNNB1 and MYC are collectively associated with multiple "driver genes” such as CCND1, CDH1, CDKN2A, CDKN2C and many others, which are critical for the regulation of cell cycle progression, cellular differentiation and survival[5]. CTNNB1 and MYC were identified as functionally altered proteins in the integrated cell circuit, which were genetically reprogrammed to manifest the six hallmarks of cancer and dictate malignant growth[108]. MYC was also identified as one of the four original "Yamanaka factors” (collectively known as OSKM)[109]. It can manipulate cell fate to generate induced pluripotent stem cells (iPSCs) from somatic cells. Self-renewal properties and associated signaling pathways, along with reprogramming potency of iPSCs by the influence of Yamanaka factors, are also mimicked by cancer cells to produce stemness among themselves[110]. GSK3β, CTNNB1 and MYC have the ability to influence diverse cellular functions, thus affecting cell physiology to initiate and develop cancer.
GSK3β, CTNNB1 and MYC are directly associated with the progression of cervical cancer. Inactivation of GSK3β enhances cervical cancer progression and invasion, and induces drug resistance[111]. Nath et al. also showed that activation of GSK3β with nimbolide and iAkt (Akt inhibitor-VIII) can induce apoptosis, and these two molecules can be further exploited for therapeutic interventions of cervical cancer[111]. The oncogenic character of CTNNB1 in cervical carcinogenesis was deciphered years ago[112,113]. Suppression of β-catenin expression is associated with anti-proliferative activity and tumor inhibition in cervical cancer[114]. CTNNB1-mediated inhibition of cervical cancer cell proliferation was also reported via NHERF1-induced suppression of alpha-actinin-4 expression[115]. On the other hand, LGR5-induced overexpression of β-catenin was associated with cervical cancer growth, further indicating its oncogenic character during cervical cancer development[116]. CTNNB1 is also an EMT marker for cervical cancer[114]. All these oncogenic roles make β-catenin a potent "druggable” target, although this cannot be fully achieved due to adverse side effects. Only a small-molecule MSAB is known to directly target Armadillo repeat region 2 of CTNNB1 and inhibit its oncogenic signal[117]. Different classes of therapeutic agents such as enzyme inhibitors, β-catenin/TCF antagonists, and transcriptional regulators have been developed to target β-catenin indirectly[114,118]. XAV939, K-756, and WXL-8 are the tankyrase inhibitors that stimulate β-catenin degradation by stabilizing Axin[114]. PFK115-584 and CFP049090 inhibit β-catenin/TCF complex in a dose-dependent manner[119]. Aspirin and indomethacin attenuate β-catenin by modulating TCF activity[120]. Some other molecules like CRT3, iCRT5, iCRT14, BC21, BC23, HI-B1 also inhibit β-catenin-TCF4 interaction and suppress tumor growth[114]. PRI-724 was reported to inhibit the interaction between CTNNB1 and its transcriptional co-activator CBP[121].
MYC gene amplification and protein overexpression in HR-HPV-infected cervical lesions and cervical cancer were reported previously[122,123]. Fluorescence in situ hybridization (FISH) experiments revealed altered MYC expression in HR-HPV-infected cervical cancer cells due to HR-HPV genome integration within the MYC locus (chromosome band 8q24)[64]. Additionally, using FISH, amplification of MYC was investigated in cervical epithelial exfoliated cells for screening of precancerous lesions of cervical carcinoma[124]. Frequent inactivation of FBW7 in cervical cancer (13%) promotes overexpression of MYC and cancer progression[125]. MYC has been described as the "undruggable” target for many decades[99]. But recently, both direct and indirect therapeutic targets of MYC are in clinical trials[99,126,127]. Omomyc, one of the most promising therapeutic drugs directly targeting MYC, is currently under pre-clinical trial[126]. Among the different alternative approaches to inhibit MYC, targeting MYC transcription and translation, as well as its stability, are crucial[127]. The main indirect approach to inhibit MYC is to target MYC/MAX heterodimer. Different small molecule inhibitors such as 10058-F4, 10074-G5, Mycro3, MYCMI-6 and KJ-Pyr-9 were reported to interfere with MYC/MAX interaction[126].
Collectively, we have attempted to understand the critical roles of three important cell fate regulatory pathways, Hedgehog, Wnt and Notch, in the initiation and progression of cervical cancer. We further identified crosstalk molecules among these pathways, which are involved in the regulation of cellular growth, proliferation and survival. Finally, we have discussed the available drug regime against the identified valuable crosstalk molecules. We found that all available therapeutic approaches are primarily targeting a single molecule at a time. This might not be effective in treating cervical cancer or other cancers due to the complexities of disease progression and possible deregulation of crucial cellular processes. We predict that applying combinatorial therapies against the crosstalk molecules may achieve better clinical outcomes in the future.
DECLARATIONS
Authors’ contributions
Drafted and edited the review, created figures and analyzed data: Samadder S
Data mining, analysis and presentation: Paul P
Edited the review and created figures: De A
Availability of data and materials
All data mined and/or generated for creating PPI network will be made freely available upon request.
Financial support and sponsorship
None.
Conflicts of interest
All authors declared that there are no conflicts of interest.
Ethical approval and consent to participate
Not applicable.
Consent for publication
Not applicable.
Copyright
© The Author(s) 2023
REFERENCES
1. Sung H, Ferlay J, Siegel RL, et al. Global cancer statistics 2020: globocan estimates of incidence and mortality worldwide for 36 cancers in 185 countries. CA Cancer J Clin 2021;71:209-49.
2. Arbyn M, Weiderpass E, Bruni L, et al. Estimates of incidence and mortality of cervical cancer in 2018: a worldwide analysis. Lancet Glob Health 2020;8:e191-203.
3. Cogliano VJ, Baan R, Straif K, et al. Preventable exposures associated with human cancers. J Natl Cancer Inst 2011;103:1827-39.
4. Castanheira CP, Sallas ML, Nunes RAL, Lorenzi NPC, Termini L. Microbiome and cervical cancer. Pathobiology 2021;88:187-97.
5. Vogelstein B, Papadopoulos N, Velculescu VE, Zhou S, Diaz Jr LA, Kinzler KW. Cancer genome landscapes. Science 2013;339:1546-58.
6. Perrimon N, Pitsouli C, Shilo BZ. Signaling mechanisms controlling cell fate and embryonic patterning. Cold Spring Harb Perspect Biol 2012;4:a005975.
7. Feinberg AP, Koldobskiy MA, Göndör A. Epigenetic modulators, modifiers and mediators in cancer aetiology and progression. Nat Rev Genet 2016;17:284-99.
8. Doorbar J, Zheng K, Aiyenuro A, et al. Principles of epithelial homeostasis control during persistent human papillomavirus infection and its deregulation at the cervical transformation zone. Curr Opin Virol 2021;51:96-105.
9. Dyke JG, Weaver IS. The emergence of environmental homeostasis in complex ecosystems. PLoS Comput Biol 2013;9:e1003050.
10. Basanta D, Anderson ARA. Homeostasis back and forth: an ecoevolutionary perspective of cancer. Cold Spring Harb Perspect Med 2017;7:a028332.
11. Francis N, Borniger JC. Cancer as a homeostatic challenge: the role of the hypothalamus. Trends Neurosci 2021;44:903-14.
12. Slominski RM, Raman C, Chen JY, Slominski AT. How cancer hijacks the body’s homeostasis through the neuroendocrine system. Trends Neurosci 2023;46:263-75.
14. Di Fiore R, Suleiman S, Drago-Ferrante R, et al. Cancer stem cells and their possible implications in cervical cancer: a short review. Int J Mol Sci 2022;23:5167.
15. Kowatsch C, Woolley RE, Kinnebrew M, Rohatgi R, Siebold C. Structures of vertebrate Patched and Smoothened reveal intimate links between cholesterol and Hedgehog signalling. Curr Opin Struct Biol 2019;57:204-14.
16. Kotulak-Chrząszcz A, Kmieć Z, Wierzbicki PM. Sonic hedgehog signaling pathway in gynecological and genitourinary cancer (review). Int J Mol Med 2021;47:106.
17. Hui CC, Angers S. Gli proteins in development and disease. Annu Rev Cell Dev Biol 2011;27:513-37.
18. Niewiadomski P, Niedziółka SM, Markiewicz Ł, Uśpieński T, Baran B, Chojnowska K. Gli proteins: regulation in development and cancer. Cells 2019;8:147.
19. Garcia N, Ulin M, Al-Hendy A, Yang Q. The role of hedgehog pathway in female cancers. J Cancer Sci Clin Ther 2020;4:487-98.
20. Hanna A, Shevde LA. Hedgehog signaling: modulation of cancer properies and tumor mircroenvironment. Mol Cancer 2016;15:24.
21. Chaudary N, Pintilie M, Hedley D, et al. Hedgehog pathway signaling in cervical carcinoma and outcome after chemoradiation. Cancer 2012;118:3105-15.
22. Samarzija I, Beard P. Hedgehog pathway regulators influence cervical cancer cell proliferation, survival and migration. Biochem Biophys Res Commun 2012;425:64-9.
23. Chakraborty C, Dutta S, Mukherjee N, et al. Inactivation of PTCH1 is associated with the development of cervical carcinoma: clinical and prognostic implication. Tumour Biol 2015;36:1143-54.
24. Ojesina AI, Lichtenstein L, Freeman SS, et al. Landscape of genomic alterations in cervical carcinomas. Nature 2014;506:371-5.
25. Vishnoi K, Mahata S, Tyagi A, et al. Cross-talk between human papillomavirus oncoproteins and hedgehog signaling synergistically promotes stemness in cervical cancer cells. Sci Rep 2016;6:34377.
26. Deng Y, Peng D, Xiao J, et al. Inhibition of the transcription factor ZNF281 by SUFU to suppress tumor cell migration. Cell Death Differ 2023;30:702-15.
27. Rodrigues C, Joy LR, Sachithanandan SP, Krishna S. Notch signalling in cervical cancer. Exp Cell Res 2019;385:111682.
28. Bray SJ. Notch signalling: a simple pathway becomes complex. Nat Rev Mol Cell Biol 2006;7:678-89.
29. Borggrefe T, Oswald F. The Notch signaling pathway: transcriptional regulation at Notch target genes. Cell Mol Life Sci 2009;66:1631-46.
30. Andersen P, Uosaki H, Shenje LT, Kwon C. Non-canonical Notch signaling: emerging role and mechanism. Trends Cell Biol 2012;22:257-65.
31. Ayaz F, Osborne BA. Non-canonical notch signaling in cancer and immunity. Front Oncol 2014;4:345.
33. Lathion S, Schaper J, Beard P, Raj K. Notch1 can contribute to viral-induced transformation of primary human keratinocytes. Cancer Res 2003;63:8687-94.
34. Yousif NG, Sadiq AM, Yousif MG, Al-Mudhafar RH, Al-Baghdadi JJ, Hadi N. Notch1 ligand signaling pathway activated in cervical cancer: poor prognosis with high-level JAG1/Notch1. Arch Gynecol Obstet 2015;292:899-904.
35. Chen Y, Wu Q, Lin J, Wei J. DARS-AS1 accelerates the proliferation of cervical cancer cells via miR-628-5p/JAG1 axis to activate Notch pathway. Cancer Cell Int 2020;20:535.
36. Yu L, Li W. Abnormal activation of notch 1 signaling causes apoptosis resistance in cervical cancer. Int J Clin Exp Pathol 2022;15:11-9.
37. Rangarajan A, Syal R, Selvarajah S, Chakrabarti O, Sarin A, Krishna S. Activated Notch1 signaling cooperates with papillomavirus oncogenes in transformation and generates resistance to apoptosis on matrix withdrawal through PKB/Akt. Virology 2001;286:23-30.
38. Veeraraghavalu K, Subbaiah VK, Srivastava S, Chakrabarti O, Syal R, Krishna S. Complementation of human papillomavirus type 16 E6 and E7 by Jagged1-specific Notch1-phosphatidylinositol 3-kinase signaling involves pleiotropic oncogenic functions independent of CBF1;Su(H);Lag-1 activation. J Virol 2005;79:7889-98.
39. Liu J, Ye F, Chen H, Lü W, Zhou C, Xie X. Expression of differentiation associated protein Hes1 and Hes5 in cervical squamous carcinoma and its precursors. Int J Gynecol Cancer 2007;17:1293-9.
40. Srivastava S, Ramdass B, Nagarajan S, Rehman M, Mukherjee G, Krishna S. Notch1 regulates the functional contribution of RhoC to cervical carcinoma progression. Br J Cancer 2010;102:196-205.
41. Vázquez-Ulloa E, Ramos-Cruz AC, Prada D, et al. Loss of nuclear NOTCH1, but not its negative regulator NUMB, is an independent predictor of cervical malignancy. Oncotarget 2018;9:18916-28.
42. Sun L, Liu M, Sun GC, et al. Notch Signaling activation in cervical cancer cells induces cell growth arrest with the involvement of the nuclear receptor NR4A2. J Cancer 2016;7:1388-95.
43. Kadian LK, Gulshan G, Ahuja P, et al. Aberrant promoter methylation of NOTCH1 and NOTCH3 and its association with cervical cancer risk factors in North Indian population. Am J Transl Res 2020;12:2814-26.
45. Corda G, Sala A. Non-canonical WNT/PCP signalling in cancer: Fzd6 takes centre stage. Oncogenesis 2017;6:e364.
47. McMellen A, Woodruff ER, Corr BR, Bitler BG, Moroney MR. Wnt signaling in gynecologic malignancies. Int J Mol Sci 2020;21:4272.
48. Perez-Plasencia C, Duenas-Gonzalez A, Alatorre-Tavera B. Second hit in cervical carcinogenesis process: involvement of wnt/beta catenin pathway. Int Arch Med 2008;1:10.
49. Zhang Y, Liu B, Zhao Q, Hou T, Huang X. Nuclear localizaiton of β-catenin is associated with poor survival and chemo-/radioresistance in human cervical squamous cell cancer. Int J Clin Exp Pathol 2014;7:3908-17.
50. Chung MT, Sytwu HK, Yan MD, et al. Promoter methylation of SFRPs gene family in cervical cancer. Gynecol Oncol 2009;112:301-6.
51. Lin YW, Chung MT, Lai HC, et al. Methylation analysis of SFRP genes family in cervical adenocarcinoma. J Cancer Res Clin Oncol 2009;135:1665-74.
52. Chung MT, Lai HC, Sytwu HK, et al. SFRP1 and SFRP2 suppress the transformation and invasion abilities of cervical cancer cells through Wnt signal pathway. Gynecol Oncol 2009;112:646-53.
53. Ying Y, Tao Q. Epigenetic disruption of the WNT/beta-catenin signaling pathway in human cancers. Epigenetics 2009;4:307-12.
54. Wang B, Zhang H, Wei L, Li Y. Expression of dickkopf-1 and twist2 in cervical squamous cell carcinoma and their correlation with vasculogenic mimicry. J Healthc Eng 2022;2022:9288476.
55. Koike J, Takagi A, Miwa T, Hirai M, Terada M, Katoh M. Molecular cloning of Frizzled-10, a novel member of the Frizzled gene family. Biochem Biophys Res Commun 1999;262:39-43.
56. Kirikoshi H, Sekihara H, Katoh M. WNT10A and WNT6, clustered in human chromosome 2q35 region with head-to-tail manner, are strongly coexpressed in SW480 cells. Biochem Biophys Res Commun 2001;283:798-805.
57. Kirikoshi H, Sekihara H, Katoh M. Expression of WNT14 and WNT14B mRNAs in human cancer, up-regulation of WNT14 by IFNgamma and up-regulation of WNT14B by beta-estradiol. Int J Oncol 2001;19:1221-5.
58. Kirikoshi H, Katoh M. Expression and regulation of WNT10B in human cancer: up-regulation of WNT10B in MCF-7 cells by beta-estradiol and down-regulation of WNT10B in NT2 cells by retinoic acid. Int J Mol Med 2002;10:507-11.
59. Okino K, Nagai H, Hatta M, et al. Up-regulation and overproduction of DVL-1, the human counterpart of the Drosophila dishevelled gene, in cervical squamous cell carcinoma. Oncol Rep 2003;10:1219-23.
60. Chakraborty C, Samadder S, Roychowdhury A, et al. Activation of Wnt-β-catenin pathway in basal-parabasal layers of normal cervical epithelium comparable during development of uterine cervical carcinoma. Mol Cell Biochem 2018;443:121-30.
61. Liu XF, Li XY, Zheng PS, Yang WT. DAX1 promotes cervical cancer cell growth and tumorigenicity through activation of Wnt/β-catenin pathway via GSK3β. Cell Death Dis 2018;9:339.
62. The Cancer Genome Atlas Research Network. Integrated genomic and molecular characterization of cervical cancer. Nature 2017;543:378-84.
63. Feng Q, Li S, Ma HM, Yang WT, Zheng PS. LGR6 activates the Wnt/β-catenin signaling pathway and forms a β-catenin/TCF7L2/LGR6 feedback loop in LGR6(high) cervical cancer stem cells. Oncogene 2021;40:6103-14.
64. Zhou L, Li H, Zhang D, et al. OTX1 promotes tumorigenesis and progression of cervical cancer by regulating the Wnt signaling pathway. Oncol Rep 2022;48:204.
66. zur Hausen H. Papillomaviruses and cancer: from basic studies to clinical application. Nat Rev Cancer 2002;2:342-50.
67. Pal A, Kundu R. Human papillomavirus E6 and E7: the cervical cancer hallmarks and targets for therapy. Front Microbiol 2019;10:3116.
68. Mitra S, Mazumder Indra D, Bhattacharya N, et al. RBSP3 is frequently altered in premalignant cervical lesions: clinical and prognostic significance. Genes Chromosomes Cancer 2010;49:155-70.
69. Sharp TV, Munoz F, Bourboulia D, et al. LIM domains-containing protein 1 (LIMD1), a tumor suppressor encoded at chromosome 3p21.3, binds pRB and represses E2F-driven transcription. Proc Natl Acad Sci USA 2004;101:16531-6.
70. Chakraborty C, Mitra S, Roychowdhury A, et al. Deregulation of LIMD1-VHL-HIF-1α-VEGF pathway is associated with different stages of cervical cancer. Bio-Chem J 2018;475:1793-806.
71. Kersemaekers AM, Fleuren GJ, Kenter GG, et al. Oncogene alterations in carcinomas of the uterine cervix: overexpression of the epidermal growth factor receptor is associated with poor prognosis. Clin Cancer Res 1999;5:577-86.
72. Shen L, Shui Y, Wang X, et al. EGFR and HER2 expression in primary cervical cancers and corresponding lymph node metastases: implications for targeted radiotherapy. BMC Cancer 2008;8:232.
73. Iida K, Nakayama K, Rahman MT, et al. EGFR gene amplification is related to adverse clinical outcomes in cervical squamous cell carcinoma, making the EGFR pathway a novel therapeutic target. Br J Cancer 2011;105:420-7.
74. Arias-Pulido H, Joste N, Chavez A, et al. Absence of epidermal growth factor receptor mutations in cervical cancer. Int J Gynecol Cancer 2008;18:749-54.
75. Spangle JM, Munger K. The HPV16 E6 oncoprotein causes prolonged receptor protein tyrosine kinase signaling and enhances internalization of phosphorylated receptor species. PLoS Pathog 2013;9:e1003237.
76. Venuti A, Paolini F, Nasir L, et al. Papillomavirus E5: the smallest oncoprotein with many functions. Mol Cancer 2011;10:140.
77. Liao S, Deng D, Zhang W, et al. Human papillomavirus 16/18 E5 promotes cervical cancer cell proliferation, migration and invasion in vitro and accelerates tumor growth in vivo. Oncol Rep 2013;29:95-102.
78. Morgan EL, Scarth JA, Patterson MR, et al. E6-mediated activation of JNK drives EGFR signalling to promote proliferation and viral oncoprotein expression in cervical cancer. Cell Death Differ 2021;28:1669-87.
79. Skoda AM, Simovic D, Karin V, Kardum V, Vranic S, Serman L. The role of the Hedgehog signaling pathway in cancer: a comprehensive review. Bosn J Basic Med Sci 2018;18:8-20.
80. Niyaz M, Khan MS, Mudassar S. Hedgehog signaling: an achilles’ heel in cancer. Transl Oncol 2019;12:1334-44.
81. Zhou B, Lin W, Long Y, et al. Notch signaling pathway: architecture, disease, and therapeutics. Signal Transduct Target Ther 2022;7:95.
82. Castel D, Mourikis P, Bartels SJ, Brinkman AB, Tajbakhsh S, Stunnenberg HG. Dynamic binding of RBPJ is determined by Notch signaling status. Genes Dev 2013;27:1059-71.
83. Katoh M, Katoh M. Notch ligand, JAG1, is evolutionarily conserved target of canonical WNT signaling pathway in progenitor cells. Int J Mol Med 2006;17:681-5.
84. Lecarpentier Y, Schussler O, Hébert JL, Vallée A. Multiple targets of the canonical WNT/β-catenin signaling in cancers. Front Oncol 2019;9:1248.
85. Song L, Li ZY, Liu WP, Zhao MR. Crosstalk between Wnt/β-catenin and Hedgehog/Gli signaling pathways in colon cancer and implications for therapy. Cancer Biol Ther 2015;16:1-7.
86. Heilmann AM, Dyson NJ. Phosphorylation puts the pRb tumor suppressor into shape. Genes Dev 2012;26:1128-30.
88. Szklarczyk D, Gable AL, Lyon D, et al. STRING v11: protein-protein association networks with increased coverage, supporting functional discovery in genome-wide experimental datasets. Nucleic Acids Res 2019;47:D607-13.
89. Shannon P, Markiel A, Ozier O, et al. Cytoscape: a software environment for integrated models of biomolecular interaction networks. Genome Res 2003;13:2498-504.
90. Mishra S. CSNK1A1 and Gli2 as novel targets identified through an integrative analysis of gene expression data, protein-protein interaction and pathways networks in glioblastoma tumors: can these two be antagonistic proteins? Cancer Inform 2014;13:93-108.
91. Zhang X, Wu F, Yang N, et al. In silico methods for identification of potential therapeutic targets. Interdiscip Sci 2022;14:285-310.
93. Valenta T, Hausmann G, Basler K. The many faces and functions of β-catenin. EMBO J 2012;31:2714-36.
95. Paul I, Bhattacharya S, Chatterjee A, Ghosh MK. Current understanding on EGFR and Wnt/β-catenin signaling in glioma and their possible crosstalk. Genes Cancer 2013;4:427-46.
96. Zeisberg M, Neilson EG. Biomarkers for epithelial-mesenchymal transitions. J Clin Invest 2009;119:1429-37.
97. Klinakis A, Szabolcs M, Politi K, Kiaris H, Artavanis-Tsakonas S, Efstratiadis A. Myc is a Notch1 transcriptional target and a requisite for Notch1-induced mammary tumorigenesis in mice. Proc Natl Acad Sci USA 2006;103:9262-7.
98. Dang CV, O’Donnell KA, Zeller KI, Nguyen T, Osthus RC, Li F. The c-Myc target gene network. Semin Cancer Biol 2006;16:253-64.
99. Llombart V, Mansour MR. Therapeutic targeting of "undruggable” MYC. EBioMedicine 2022;75:103756.
100. Adhikary S, Eilers M. Transcriptional regulation and transformation by Myc proteins. Nat Rev Mol Cell Biol 2005;6:635-45.
101. Bretones G, Delgado MD, León J. Myc and cell cycle control. Biochim Biophys Acta 2015;1849:506-16.
102. García-Gutiérrez L, Delgado MD, León J. MYC oncogene contributions to release of cell cycle brakes. Genes 2019;10:244.
103. Malumbres M, Barbacid M. Mammalian cyclin-dependent kinases. Trends Biochem Sci 2005;30:630-41.
105. Sahin I, Eturi A, De Souza A, et al. Glycogen synthase kinase-3 beta inhibitors as novel cancer treatments and modulators of antitumor immune responses. Cancer Biol Ther 2019;20:1047-56.
106. Sears R, Nuckolls F, Haura E, Taya Y, Tamai K, Nevins JR. Multiple Ras-dependent phosphorylation pathways regulate Myc protein stability. Genes Dev 2000;14:2501-14.
107. Robertson H, Hayes JD, Sutherland C. A partnership with the proteasome; the destructive nature of GSK3. Bio-Chem Pharmacol 2018;147:77-92.
109. Takahashi K, Yamanaka S. A decade of transcription factor-mediated reprogramming to pluripotency. Nat Rev Mol Cell Biol 2016;17:183-93.
110. Shamsian A, Sahebnasagh R, Norouzy A, Hussein SH, Ghahremani MH, Azizi Z. Cancer cells as a new source of induced pluripotent stem cells. Stem Cell Res Ther 2022;13:459.
111. Nath N, Rana A, Nagini S, Mishra R. Glycogen synthase kinase-3β inactivation promotes cervical cancer progression, invasion, and drug resistance. Biotechnol Appl Biochem 2022;69:1929-41.
112. Shinohara A, Yokoyama Y, Wan X, et al. Cytoplasmic/nuclear expression without mutation of exon 3 of the beta-catenin gene is frequent in the development of the neoplasm of the uterine cervix. Gynecol Oncol 2001;82:450-5.
113. de Putte G, Kristensen GB, Baekelandt M, Lie AK, Holm R. E-cadherin and catenins in early squamous cervical carcinoma. Gynecol Oncol 2004;94:521-7.
114. Wang B, Li X, Liu L, Wang M. β-Catenin: oncogenic role and therapeutic target in cervical cancer. Biol Res 2020;53:33.
115. Wang Q, Qin Q, Song R, et al. NHERF1 inhibits beta-catenin-mediated proliferation of cervical cancer cells through suppression of alpha-actinin-4 expression. Cell Death Dis 2018;9:668.
116. Chen Q, Cao HZ, Zheng PS. LGR5 promotes the proliferation and tumor formation of cervical cancer cells through the Wnt/β-catenin signaling pathway. Oncotarget 2014;5:9092-105.
117. Hwang SY, Deng X, Byun S, et al. Direct Targeting of β-Catenin by a small molecule stimulates proteasomal degradation and suppresses oncogenic Wnt/β-catenin signaling. Cell Rep 2016;16:28-36.
118. Zhang Y, Wang X. Targeting the Wnt/β-catenin signaling pathway in cancer. J Hematol Oncol 2020;13:165.
119. Lepourcelet M, Chen YN, France DS, et al. Small-molecule antagonists of the oncogenic Tcf/beta-catenin protein complex. Cancer Cell 2004;5:91-102.
120. Dihlmann S, Siermann A, von Knebel Doeberitz M. The nonsteroidal anti-inflammatory drugs aspirin and indomethacin attenuate beta-catenin/TCF-4 signaling. Oncogene 2001;20:645-53.
121. Fischer MM, Cancilla B, Yeung VP, et al. WNT antagonists exhibit unique combinatorial antitumor activity with taxanes by potentiating mitotic cell death. Sci Adv 2017;3:e1700090.
122. Abba MC, Laguens RM, Dulout FN, Golijow CD. The c-myc activation in cervical carcinomas and HPV 16 infections. Mutat Res 2004;557:151-8.
123. Gimenes F, Souza RP, de Abreu AL, Pereira MW, Consolaro ME, da Silva VR. Simultaneous detection of human papillomavirus integration and c-MYC gene amplification in cervical lesions: an emerging marker for the risk to progression. Arch Gynecol Obstet 2016;293:857-63.
124. Li T, Tang L, Bian D, Jia Y, Huang X, Zhang X. Detection of hTERC and c-MYC genes in cervical epithelial exfoliated cells for cervical cancer screening. Int J Mol Med 2014;33:1289-97.
125. Dhanasekaran R, Deutzmann A, Mahauad-Fernandez WD, Hansen AS, Gouw AM, Felsher DW. The MYC oncogene - the grand orchestrator of cancer growth and immune evasion. Nat Rev Clin Oncol 2022;19:23-36.
126. Madden SK, de Araujo AD, Gerhardt M, Fairlie DP, Mason JM. Taking the Myc out of cancer: toward therapeutic strategies to directly inhibit c-Myc. Mol Cancer 2021;20:3.
Cite This Article
How to Cite
Download Citation
Export Citation File:
Type of Import
Tips on Downloading Citation
Citation Manager File Format
Type of Import
Direct Import: When the Direct Import option is selected (the default state), a dialogue box will give you the option to Save or Open the downloaded citation data. Choosing Open will either launch your citation manager or give you a choice of applications with which to use the metadata. The Save option saves the file locally for later use.
Indirect Import: When the Indirect Import option is selected, the metadata is displayed and may be copied and pasted as needed.
About This Article
Copyright
Data & Comments
Data
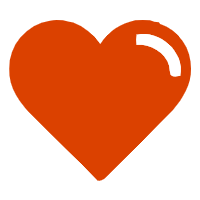
Comments
Comments must be written in English. Spam, offensive content, impersonation, and private information will not be permitted. If any comment is reported and identified as inappropriate content by OAE staff, the comment will be removed without notice. If you have any queries or need any help, please contact us at [email protected].