Novel approaches to therapeutics in pancreatic adenocarcinoma: vitamin C and tumor treatment fields
Abstract
Pancreatic ductal adenocarcinoma (PDAC) is a lethal disease with limited therapeutic options. Despite extensive clinical research over the past several decades, meaningful improvements in care standards have been challenging to achieve. Research efforts are underway on several fronts, including cytotoxic chemotherapy combinations, immunotherapy, and targeted therapy. In this review, we chose to focus less on mainstream avenues of clinical research in PDAC and highlight some novel and innovative research efforts that are typically outside the spotlight of therapies. Examples of these novel approaches include pharmacologic vitamin C and electromagnetic fields. This review’s scope is to present the biological basis of the anti-cancer potential and the early clinical data, as well as the future landscape of these agents, including ongoing clinical trials in these therapeutic avenues.
Keywords
INTRODUCTION
Pancreatic ductal adenocarcinoma (PDAC) is a leading cause of cancer-related mortality with limited therapeutic options and poor outcomes. Data from the global cancer observatory model project approximately 485,000 PDAC cases globally in 2020, with an associated mortality of around 456,000[1]. It is estimated that 57,600 patients will be diagnosed with about 47,050 deaths in the United States, making it the 3rd leading cause of cancer mortality with a relative 5-year survival rate of only 9%[2]. Surgical resection remains the only modality with a curative potential. However, only ~20% of patients present with early-stage disease amenable to resection[3], and the majority of these patients eventually develop metastatic disease even with recent improvements in surgical techniques and adjuvant therapies[4-6]. Despite extensive translational and clinical investigations over the last three decades, meaningful advances in PDAC therapeutics have not been realized. At least 30 out of 32 phase III clinical trials assessing several interventions have failed to add significant value to this disease’s standard of care[7]. Such disappointments highlight the exceptional difficulties in treating PDAC. There is, therefore, an unmet clinical need for innovative therapeutic modalities that target novel characteristic features of PDAC.
Historically, PDAC clinical research focused on the traditional modalities used to treat cancer, such as cytotoxic chemotherapy or radiation, and progress has been slow. More recently, several contemporary therapeutics such as targeted therapy and immunotherapy have materialized but with suboptimal headway. Therefore, the pursuit of more effective anti-cancer therapies continues. The majority of developed and current clinical research targeting metastatic PDAC can be categorized into four groups, as summarized in Table 1: Cytotoxic chemotherapeutics, immunotherapy, targeted therapy and targeting PDAC-specific features. These four highly researched categories are reviewed and critiqued heavily elsewhere. In this review, we attempt to shed light on some non-traditional novel therapeutics that are relatively outside the mainstream of the clinical research efforts in progress. These are innovative in principle and attractive to patients seeking creative solutions. We thus summarize the early limited data about some of these options to guide both clinicians about their existence and researchers about possible avenues for development. These innovative therapeutics encompass a wide range of efforts, such as using vitamin C in pancreatic cancer therapy and harnessing the effect of physical interventions such as electromagnetic fields. We will review some highlights of the basis of these concepts and reflect on the ongoing clinical trials.
Major categories of ongoing clinical research in metastatic pancreas cancer 2015-2020
Cytotoxic chemotherapy | Novel agents (Glufosfamide, nano micelles, EndoTag, etc.) Repurposing of existing agents Sequence of chemotherapy agents |
Immunotherapy | Immune checkpoint inhibitors Vaccines Adoptive cellular therapy Immune modulators |
Targeted therapy | Exploiting DNA damage response defects Intracellular target-linked therapy (mesothelin, Claudin 18.2, etc.) Interference with a signaling pathway |
Targeting PDAC-specific features | Microenvironment Metabolic pathways Cancer stem cells interventions |
VITAMIN C (ASCORBIC ACID, ASCORBATE)
Vitamin C has long been hailed as a potential anti-cancer agent. Vitamin C is a water-soluble vitamin essential for the biosynthesis of collagen that also acts as an important physiological antioxidant[8]. In contrast to most animals and plants, humans cannot produce vitamin C and are dependent on dietary intake[9]. Vitamin C regulates several vital biological processes by acting as an electron donor. At physiological micro molar concentrations, vitamin C functions as an antioxidant reducing harmful reactive oxygen species[9]. However, at pharmacologic millimolar plasma concentrations, it paradoxically functions as a pro-oxidant[10]. In addition to its involvement in redox reactions, vitamin C affects iron metabolism[11] and is a critical cofactor for numerous enzymes[10]. After vitamin C intake, it can be oxidized both intracellularly and extracellularly. Extracellular vitamin C is oxidized through two consecutive steps by free radicals and ascorbate oxidase into dehydroascorbic acid (DHA)[10]. Vitamin C can enter cells either in its reduced form, Ascorbate, utilizing sodium-dependent transporters or in its oxidized form, DHA, through glucose transporters (GLUT)[10]. DHA has a half-life of seconds[11] and represents 1%-5% of vitamin C in the human body[12]. It then performs two crucial steps. In the first step, DHA reacts with labile iron in the extracellular space, which triggers a cascade of events that yields extracellular hydrogen peroxide (H2O2)[13]. The second step involves transportation of the remainder inside the cell through a group of GLUTs[14]. Inside the cell, DHA is swiftly reduced back to ascorbate by reacting with the reduced form of glutathione (GSH). Oxidized glutathione [glutathione disulfide (GSSG)] is then recycled back to GSH by NADPH[15]. This process explains the two most developed proposed anti-cancer effects of vitamin C, generation of H2O2 causing oxidative stress and the consumption of reduced Glutathione/NADPH/GADPH leading to a glycolytic crisis as detailed later.
The concept of using vitamin C to treat cancer has generated much controversy over the last several decades. In the 1970s, researchers hypothesized that vitamin C might limit cancer cell migration by preventing the breakdown of the extracellular matrix[16,17]. Initial early case reports and trials demonstrated a clinical benefit[18,19]. In one of the earlier publications, 100 patients with incurable cancer were given intravenous vitamin C with a notable 300-day survival advantage compared to 1000 matched patients who had received identical treatments except for vitamin C[20]. The 1100 patients on that trial consisted of patients treated by the same clinicians in the same facility with different tumor types including colon, lung, gastric, breast, kidney, bladder, rectum, ovary, and pancreas cancers who were deemed “untreatable” per the definition of the Scottish medical practice of the time. Each patient among the vitamin C group was matched with 10 control patients of similar age, gender, and tumor type. Subsequent trials, however, failed to show benefit. Two randomized, double-blinded clinical trials in the 1980s using oral vitamin C established no survival advantage for the treatment groups over the placebo controls[21,22]. These disappointments led to diminishing interest in the anti-cancer role of vitamin C for decades. It was not until pharmacokinetic studies confirmed that potentially therapeutic doses are only achievable through intravenous administration that interest resurged[9,23]. This understanding of ascorbate’s pharmacokinetics generated renewed interest in its cancer therapeutic potential and led to preclinical and clinical investigations of vitamin C in cancer patients.
The selective anti-cancer effects of vitamin C was shown in several in vitro and in vivo models. The impact of pharmacological concentrations of vitamin C on 43 different cancer cell lines alongside five normal cell lines was delineated in a comprehensive experiment[24]. Non-neoplastic cells were unaffected even in very high ascorbate concentrations (20 mM), while most cancer cell lines showed decreased growth with concentrations < 10 mM ascorbate. Specifically to pancreatic cancer cells, a study demonstrated selective sensitivity of three pancreatic cancer cell lines vs. a non-neoplastic immortalized pancreatic ductal epithelial cell line to vitamin C[25]. This selective cytotoxicity was again confirmed in a panel of eight human and one murine pancreatic cancer cell lines that were all sensitive to concentrations below 5 mM ascorbate. In contrast, even at concentrations as high as 20 mM ascorbate, the viability of the non-tumorigenic pancreatic ductal epithelial cells and fibroblasts was not significantly affected[26]. In vivo evidence of vitamin C’s anti-cancer effect was displayed in several tumor xenograft models, including pancreatic cancer[24]. More studies confirmed that only parenteral vitamin C administration in animals was sufficient to obtain cytotoxic levels[27]. These results were reproduced in PANC-1 orthotopic mice xenograft with evidence of a decreased number of metastases and average tumor weights in the ascorbate-treated animals[26]. As a result of the growing preclinical evidence, several clinical trials exploring the safety and efficacy of intravenous high-dose vitamin C for treating various types of cancers, including pancreas, have emerged either as monotherapy or in combination with chemotherapy[28].
Several hypotheses regarding the anti-cancer mechanism of action of pharmacologic vitamin C have been proposed over the years. The most developed proposed hypothesis with robust laboratory evidence stems from its ability to act as a pro-oxidant at millimolar concentration. Treatment with high-dose ascorbate leads to the extracellular formation of H2O2 in the tumor milieu that will later transfer to the intracellular compartment through diffusion[29]. This process occurs rapidly in the presence of catalytic metal ions concentrated in the tumor microenvironment, especially iron. In the presence of iron, ascorbate is quickly oxidized as it reduces Fe3+ to Fe2+, which then reacts with oxygen, producing superoxide radicals. Two superoxide radicals then undergo dismutation to produce hydrogen peroxide. Hydrogen Peroxide then diffuses inside the cells, where it reacts with ferrous iron through the Fenton reaction producing hydroxyl radical. Hydroxyl radical, in turn, causes DNA damage and leads to cell death[30,31]. This hypothesis is supported with multiple preclinical reports corroborating that the addition of catalase to the medium reversed the decrease in cell viability[29,32]. Subsequent reports confirmed similar results in multiple pancreatic cancer cell lines and demonstrated a time and dose-dependent increase in measured H2O2 production with increased ascorbate concentrations leading to cell death with reversal of the effect with treatment with catalase[12,31]. Other in vitro studies focused on cancer cell lines resistant to vitamin C and confirmed cross-reactivity against H2O2, significantly elevating enzymatic activity of intrinsic catalase, and reversal of resistance upon silencing of catalase by sh-RNA[33]. In vivo, the increase in peri-tumoral H2O2 was shown after a single injection in animal models[30]. The selective cytotoxicity to cancer cells but not to normal cells could be explained by increased iron in the tumor microenvironment, increasing H2O2 production, and higher catalase enzymatic activity in normal tissue[34].
The second popular proposed hypothesis of the anti-cancer effect of vitamin C relates to the action of DHA. Extracellular H2O2 perpetuates the presence of reduced ascorbate in the form of DHA. Accumulated extracellular DHA is then transported intracellularly through GLUT transporters. Subsequently, DHA consumes the intracellular reducing potential of reduced glutathione and NADPH. This reduction depletes intracellular antioxidants and increases endogenous levels of reactive oxygen species (ROS). The increase of ROS, in turn, inactivates a glycolytic enzyme, glyceraldehyde 3-phosphate dehydrogenase (GAPDH). ROS ultimately causes a cascade of events that leads to the depletion of NAD+ and further inhibition of GAPDH[35]. The result impedes glycolysis in cancer cells, causing lower ATP production, energy crisis, and cell death[13,35]. Perhaps the most substantial support for this hypothesis comes from the differential vitamin C sensitivity of KRAS and BRAF mutated colorectal cancer cells in comparison to cells without mutations. Cells with KRAS/BRAF mutations have increased expression of GLUT1 and were shown to have increased uptake of DHA compared to wild-type cells. This increased uptake was nullified by either using GLUT1 inhibitors in the mutated cells or overexpressing GLUT1 in wild-type cells. Metabolomics assays showed that treatment with vitamin C caused accumulation of glycolytic intermediates upstream of GAPDH accompanied by depletion of downstream mediators suggesting that GAPDH was inhibited. Collectively, these findings indicate that KRAS mutated cell cytotoxicity was mediated through inhibition of glycolysis[35]. The Warburg effect could help explain ascorbate effects’ selectivity, a phenomenon where metabolic reprogramming in cancer cells leads to increased dependence on glycolysis for ATP production compared to mitochondrial oxidative phosphorylation. Therefore, glycolytic inhibition causes catastrophic effects on cancer cells with minimal impact on normal cells.
A third interesting hypothesized anti-cancer mechanism of vitamin C is related to hypoxia-induced factor (HIF). Many solid tumors are characterized by hypoxia, which is defined here as the partial pressure of oxygen [pO2] < 10 mmHg or < 1% at the tissue level[36]. Under hypoxic conditions, tumor cells activate the HIF cascade, an αβ heterodimeric transcription factor, leading to the activation of several pro malignant processes such as increased angiogenesis, enhanced glycolysis, and other features that promote tumor growth[37]. HIF-β subunits are constitutive proteins and the active complex regulation is achieved by the abundance and activity of HIF-α subunits. The HIF-α subunits, in turn, are regulated by a group of HIF hydroxylases. Under normoxic conditions, HIF1α is hydroxylated and eventually undergoes proteasomal degradation[37]. Conversely, under hypoxic conditions, the HIF hydroxylases are inactive, leading to the stabilization and activation of HIF. HIF hydroxylases require ascorbate as a cofactor to recycle Fe2+. This dependency implies that ascorbate treatment may enhance the activity of HIF hydroxylases, which leads to HIF α degradation, thus inhibiting the activity of the HIF cascade and suppressing tumor growth[38]. A simplified illustration of these interactive mechanisms is depicted in Figure 1 below.
Figure 1. Hypotheses for Vitamin C anti-cancer potential. H2O2: Hydrogen peroxide; DHA: dehadroascorbic acid; GLUT1: glucose transporters; HIF: hypoxia-induced factor; SVCTs: sodium-vitamin C co transporters; GSSG: glutathione disulfide; GSH: glutathione.
These three possible mechanisms of cytotoxicity have a significant role in pancreatic cancer. Pancreatic adenocarcinoma has a well known dense microenvironment with an abundance of tumor-associated macrophages (TAMs) playing several roles in tumor progression[39]. As one of several effects, TAMs act as iron donating and re-distributing cells to the microenvironment[40], hence assuring the presence of high labile iron content for efficient H2O2 production in the peri-tumoral space. Moreover, 95% of PDAC cases have KRAS mutation, which is associated with high GLUT expression with expected increased DHA uptake and a subsequent glycolytic crisis. KRAS mutated cells are dependent highly on glycolysis, making pancreas cancer cells especially vulnerable to vitamin C treatment. Finally, pancreas cancers are renowned for their extremely hypoxic conditions making HIF signaling a prominent target[41]. For the reasons mentioned above, pancreas adenocarcinoma should be an ideal research target for therapy with vitamin C. Hence, several early clinical trials in this space have been performed.
CLINICAL EXPERIENCE OF VITAMIN C THERAPY IN PANCREATIC ADENOCARCINOMA
Early case reports of using high-dose parenteral ascorbate in cancer treatment came in 2004 when seven patients, including 1 with pancreatic cancer, received 10 to 100 grams/day of IV vitamin C, up to three times per week. Patients had minimal toxicities, improved QOL, and radiographic signs of response size[42]. The lone pancreas cancer case was for a gentleman who had progressed through 5-FU and Gemcitabine and achieved chemical response with CA19-9 and stable radiographic disease while on IV vitamin C. Subsequent phase 1 trial was developed and included 24 patients (1 with pancreatic cancer). This trial revealed that doses up to 1.5 g/kg IV vitamin C given three times a week were well tolerated with minimal adverse events but showed no radiographic response suggestive that vitamin C monotherapy could be sub-optimal. Both poor documented activity as a single agent, and the favorable toxicity profile noted, increased interest in combining vitamin C with chemotherapy rather than vitamin C monotherapy use[43].
There were three early phase clinical trials that targeted metastatic PDAC with combinations of chemotherapy and vitamin C in the last decade. The first trial enrolled 14 patients in 3 cohorts. Patients received standard of care chemotherapy with gemcitabine and the EGFR inhibitor erlotinib plus three different IV vitamin C doses (50, 75, and 100 g) given three times a week (TIW) for eight weeks. The primary endpoint was the regimen’s safety, while secondary endpoints included overall response rate[44]. The trial reported 23 total adverse events, with 8 being serious adverse events. All serious adverse events were attributable to disease progression or concomitant treatment with gemcitabine and erlotinib. None of these adverse events appeared explicitly related to vitamin C except for mild lightheadedness or nausea during infusion as expected from the osmotic load and resolved with eating and drinking. The trial was not powered to determine therapeutic efficacy. Interestingly, a radiographic disease control rate of 77% was noted, with all of these patients experiencing a decrease in tumor size not meeting RECIST criteria for partial response.
A similar trial enrolled 11 patients with metastatic pancreatic adenocarcinoma to receive gemcitabine with IV vitamin C administered twice weekly for the duration of therapy[45]. Again, toxicities were comparable with published trials of gemcitabine regimens. While the study was not powered to determine therapeutic efficacy, the secondary endpoint of PFS was six months, and overall survival (OS) was reported as 12 months (n = 9). These numbers compared favorably to the historical control of gemcitabine monotherapy. This group’s comparable historical control is best matched to the gemcitabine monotherapy arm in the MPACT trial, which revealed a progression-free survival (PFS) of 3.7 months and OS of approximately 6.7 months[46]. A third phase I/II trial enrolling 12 patients who received gemcitabine in addition to vitamin C TIW also showed no increased toxicities. Again, the trial was not powered for efficacy data, and 8 out of the 12 patients received therapy after progression on prior therapies. Despite that, the results were striking, with a reported median OS of 15.1 months[26]. These early clinical research efforts are summarized in Table 2.
Completed early trials with vitamin C in metastatic PDAC
Trial leading group | Regimen | No. of patients | Notable outcomes |
Thomas Jefferson University | Gemcitabine + Erlotinib + vitamin C TIW | 14 | Disease control rate 77% |
University of Iowa | Gem + vitamin c BIW | 11 | PFS 6 months OS 12 months |
University of Kansas | Gemcitabine + vitamin C TIW | 12 | OS 15.1 months |
In summary, the vision of vitamin C as a cancer therapy had swayed between disappointments and renewed interest over the past several decades. Campbell’s initial encouraging results were challenged through 2 negative trials from Mayo Clinic that utilized daily supplementation of 10 g of oral vitamin C as detailed above. As the primary difference between these trials was the route of administration, subsequent pharmacokinetic studies were performed to study any disparity in vitamin C bioavailability. Several reports confirmed that vitamin C bioavailability after oral administration is limited due to tight control of vitamin C through saturable absorption, tissue accumulation, renal reabsorption, and excretion[9,23]. While oral doses of 0.1 g daily produced a plasma concentration of ~60 μM, the supplementation of as high as 2.5 g daily of oral Vitamin C failed to increase it above 80 μM, which was well below the concentrations noted to exert the anti-cancer effect in vitro[47]. This realization was vital in igniting interest in parenteral vitamin C’s anti-cancer effects. However, significant hurdles persisted. Being a water-soluble vitamin meant that any parenteral vitamin C delivery is transient as it won’t be stored in the body. Parenteral infusion rate was optimized to 0.5-1 g/minute, which means a single dose could require several hours. Some pharmacokinetic studies revealed that therapeutic concentrations would only be sustained for ~4 hours following the infusion[24]. This obstacle remains a substantial as lengthy daily infusions are not convenient or sustainable. That difficulty and lack of a patentable form of the drug to date made further advancement in studying vitamin C as cancer therapeutic problematic. Despite these impediments, multiple small trials had been completed as detailed above, but none powered to confirm a statistically significant benefit.
With mounting evidence of good tolerance to vitamin C, and intriguing signs of potential efficacy, it’s necessary to design more extensive clinical trials to show the effect on response rate, progression-free survival, and overall survival. Currently, 2 phase II trials are enrolling patients specifically for PDAC. The first one, PACMAN 2.0 (NCT02905578), is a phase II trial targeting enrollment of 65 patients to receive Gemcitabine, nab-paclitaxel, and intravenous vitamin C at a dose of 75 mg/m2 twice a week. The primary endpoint is overall survival, with secondary endpoints including PFS and response rate. The other trial, AA NABPLAGEM (NCT03797443), is Phase IB/II Trial of High Dose Ascorbic Acid + Nab-Paclitaxel + Cisplatin + Gemcitabine. The phase I portion is a 3+3 design to determine the phase II vitamin C dose out of 4 dose levels (25, 37.5, 56.25, and 75 g/m2) given twice weekly. The trial plans to enroll 36 patients with the primary endpoint for the phase II portion being disease control rate at 18 weeks. The results of both these trials are eagerly awaited and will determine the direction of vitamin C research in metastatic pancreatic cancer.
ELECTROMAGNETIC THERAPY
Over the last century, the vast majority of medical interventions against cancer were pharmacologic. However, some have investigated the physical interventions to understand and interfere with the process of carcinogenesis and metastasis. The majority of the current physical cancer interventions concentrated on the focal non-discretional damage induced by a physical force of different sound or electromagnetic wave spectrum elements. An example of sound wave forces is high-intensity ultrasonic therapy. More commonly, elements across the electromagnetic spectrum have been used in medicine. The prime example of that is the utilization of forces at both ends of the spectrum, such as gamma and X-ray radiation at one end and microwave or radio waves at the other end, as explained in Figure 2. Additionally, alternating electric fields have been used to diagnose, research, and treat numerous medical conditions for many years. Various frequencies have been used for different bio-physiological effects. Electroporation, which is used in both research and treatment of cancer, involves multiple frequencies with repetition of short pulses (~100µs) at either low (1-10 Hz) or high (10 kHz-1 MHz) frequencies. Low-frequency alternating electric fields (< 1 kHz) are used to cause membrane depolarization leading to action potential with applications in defibrillators, ICD, fracture healing, and electroconvulsive therapy[48]. Higher frequency alternating electric fields (> 10 MHz) lead to dielectric polarization heating the tissue with clinical application in radiofrequency or microwave ablation[49]. Intermediate-frequency alternating electric fields, at frequencies between 10 kHz and 1 MHz, neither cause significant dielectric losses nor net depolarization. Therefore, a considerable interest in researching its medical utility evolved as it doesn’t interfere with heart or nerve excitability or cause significant heat tissue damage.
Figure 2. The Electromagnetic spectrum. IRE: Irreversible electroporation; H-FIRE: high-frequency electroporation.
The concept of the utilization of electromagnetic fields in cancer therapy has been researched for the past 25 years[50]. In one of the earlier efforts that highlighted the anti-cancer potential of magnetic fields, a group of researchers in Italy documented the effect of static and extremely low frequency magnetic fields on neoplastic cells and xenografts. In these experiments, two neoplastic cell lines (WiDr human colon adenocarcinoma and MCF-7 human breast adenocarcinoma) and a non-neoplastic cell line (MRC-5 embryonal lung fibroblast) were exposed to variable intensities and frequencies of magnetic fields. A significant increase in cell death was reported exclusively in the two cancer cell lines. There was an apparent increase in apoptosis in morphology in treated cancer cell lines compared to the non-neoplastic line. The treatment of nude mice with WiDr tumors xenografts with daily exposure for 70 min to magnetic fields for 4 weeks caused significant tumor growth inhibition with light microscopic evidence of increased apoptotic bodies and decreased mitotic bodies in treated xenograft. No toxic morphological changes induced by exposure were observed in the normal cells such as bone marrow, hepatocytes, or neurons[50].
Tumor-treating Fields (TTFields) emerged as one of the front runner technologies utilizing electromagnetic fields in cancer therapy. TTFields are low-intensity alternating electric fields with frequencies in the 100-300 kHz delivered through non-contact transducers placed on the skin around the tumor’s anatomic area[48]. Several hypotheses have been proposed over the last two decades, but the anti-cancer effect’s biological basis remains an area of active research. In one of the early reports, researchers documented the anti-proliferative effect of TTFields in vitro and in vivo. The impact of exposing 11 different cancer cell lines, including human breast, lung, prostate, melanoma, and glioma cancers to TTFields was documented. TTFields resulted in significant growth inhibition across all cancer cell lines, some of which lasted well past the therapy duration. This experiment emphasized that optimal electromagnetic intensity and frequency varied across different cancer types and that no significant effects were noted on non-proliferative cells viability. Time-lapse microphotography for the treated cell cultures showed mitosis prolongation, cellular destruction near the completion of the cell separation, and nuclear rotation. Fluorescence labeling of A-tubulin documented TTFields interference with microtubules’ formation and movement, leading to mitosis arrest in metaphase. Animal xenograft showed significant growth inhibition. Histopathological analysis of treated tumors showed extensive necrosis with no significant damage to surrounding tissues[51]. Similar findings were reproduced with a later comprehensive report that expanded to additional cell lines and animal xenografts[52].
Additional reports reiterated the proposal that the anti-cancer effect is partly dependent on the electromagnetic fields’ bi-directional forces on highly polar intracellular elements, such as tubulin and septin molecules. These effects elicit abnormal microtubule polymerization during spindle formation and aberrant cleavage furrow formation[53]. Both of these changes instigate prolonged mitosis, cell death, and chromosome missegregation. This missegregation is hypothesized to result in aneuploidy and hyperploidy in cells that escape apoptosis, explaining the long term influence of TTFields on clonogenic survival beyond the exposure time[53]. Several other reports reaffirmed that the anti-cancer effect of TTFields involves the direct and indirect impact on septins and, to a lesser degree, actins that lead to mitotic exit and membrane blebbing during telophase, which in turn leads to the formation of abnormal daughter cells and induction of cell death in the following interphase[54-56]. However, more recent preclinical reports showed that interruption of mitosis could not be the sole anti-cancer TTFields mechanism. For instance, several reports confirmed the additive or synergistic effect of TTFields combined with anti-mitotic chemotherapies or mitotic checkpoint inhibition[57,58]. Additionally, it was documented that TTFields exposure led to upregulation of autophagy through the AMPK pathway. AMPK depletion resulted in increased sensitization to TTFields, suggesting autophagy was a survival mechanism[59]. These results indicate that there are other mechanisms by which TTFields cause cell killing.
A second proposed anti-cancer mechanism is TTFields-induced DNA replication stress and impaired DNA repair. TTFields had been shown to downregulate Fanconi Anemia pathway genes, including BRCA, leading to replication stress and limited ability to maintain replication fork stability, which in turn decrease the speed of DNA replication, and increase replication errors, R-loops formation, and DNA single and double-stranded breaks culminating in cell death[60]. This finding was of particular importance because it highlights the additive or synergistic effect of TTFields when combined with other therapeutics targeting DNA replication (platinum, PARP inhibitors, radiation, etc.). Furthermore, some in vitro work revealed a limited heating effect that could be observed on cells during cytokinesis[61].
The proposed anti-cancer mechanisms above all have a supporting body of evidence. However, they illuminate the TTFields effect on cancer cells as if they are in an isolated medium. In reality, tumors are in continuous interaction with the patient systems and microenvironments, and a comprehensive understanding of the way TTFields interplay within these systems is needed. This insight led to two exciting hypotheses that attempted to understand the effects of TTFields within cancer-host settings. The first hypothesis suggested that electromagnetic fields induce immunogenic cell death, causing improved antitumor efficacy with anti-PD-1 combinations[62]. As explained above, some of the TTFields treated cancer cells that are not arrested in metaphase will have inappropriate chromosomal segregation. This missegregation, in turn, leads to hyperploid and aneuploid daughter cells, and both of these cell types will contribute to cancer milieu immunomodulation. On the one hand, hyperploidy is a driver for endoplasmic reticulum stress and subsequent calreticulin exposure[63]. On the other hand, aneuploidy stimulates autophagy[64]. Autophagy results in ATP efflux, a signal that attracts phagocytes such as monocytes, macrophages, and dendritic cells toward the apoptotic cells[65]. Researchers were also able to show that TTFields treated cells released high-mobility group box 1, an important piece for the processing and cross-presentation of antigens that serve as a signal for dendritic cell maturation and TH1 polarization[66,67]. In this experiment, the researchers were able to show TTField-induced immune effects in multiple aspects, including effective phagocytosis for TTFields-treated cells by dendritic cells, dendritic cells maturation in vitro, and leukocyte recruitment in vivo. As a final point, C57B1/6 mice model implanted with LLC-1 cells were treated with the combination of TTFields and anti-PD1 revealed significant reduction in tumor volumes.
The other interesting hypothesis is related to the electromagnetic field’s proposed ability to interfere with cancer cells’ metastatic properties. TTFields was shown to significantly inhibit invasion assays in glioma, breast, lung, and colon cancer cell lines[68]. It was notable that the optimal frequency and intensity combination to inhibit invasion was different from frequency and intensity for optimal proliferation inhibition. Additional in vitro experiments suggested application of TTFields in vitro led to a significant reduction in the velocity of cell migration compared with untreated control cells. Cancer cell invasion was significantly reduced compared to untreated cells in all tested cell lines and highlighted the possible anti-invasive and anti-metastatic potential for this technology[68,69]. A deeper analysis illustrated that TTFields changed the microtubule assembly and directionality resulting in interference with cancer cell migration direction[70]. These results were reinforced by the finding that induced electrical fields caused hindrance of EGFR-induced breast cancer cell motility and migration utilizing a much lower intensity induced electrical field[71]. A simplified illustration summarizing the proposed mechanisms is depicted in Figure 3 below.
In pancreas cancer, TTFields applied in vitro revealed an anti-proliferative effect on pancreatic cancer cells[72]. TTFields resulted in increased abnormal mitotic figures and reduced cells in anaphase and telophase, leading to reduced long-term clonogenicity of these cells even weeks after exposure. Furthermore, in combination with chemotherapy, TTFields application demonstrated enhanced treatment efficacy with the addition of gemcitabine, irinotecan, 5FU, and paclitaxel[72]. The same group of researchers applied TTFields 6 days after implanting orthotopic mouse models using PC-1.0 hamster pancreatic cancer cells for seven days. Chemotherapy (5FU or gemcitabine) was injected intraperitoneally on the day of TTFields treatment initiation. Postmortem analysis and tumor imaging using micro-ultrasound demonstrated that the average tumor volume in animals treated with TTFields was significantly smaller than controls. This effect was most prominent in combination with chemotherapy[72]. Subsequent postmortem examination of samples showed a significant increase in abnormal mitotic figures in the treated tumors compared to control. Based on the preclinical hypothesis of activity, a realistic computational simulation of human pancreatic cancer demonstrated that mean therapeutic TTFields intensities could be delivered to the site of the tumor using layouts with one pair of arrays placed on the back and front of the patient and one pair set on the lateral aspects of the patient delivered two almost perpendicular fields. This contraction yielded average intensities in the pancreas above the therapeutic threshold of 1 V/cm[56]. This model set the stage for testing the modality in the clinic.
CLINICAL EXPERIENCE OF TTFIELDS THERAPY IN PANCREATIC ADENOCARCINOMA
Unlike vitamin C, TTFields have an FDA approval for cancer therapy in the form of Optune device to treat Glioblastoma multiforme[73]. The clinical experience of utilizing TTFields to treat GBM has matured through several pilot and pivotal trials over the last two decades with consistent favorable safety profile. In a pilot trial of 10 patients with recurrent GBM who were treated with TTFields monotherapy, the median time to disease progression (TTP) and OS were more than double reported historical controls with no safety concerns[52]. A subsequent large phase 3 trial of TTFields in recurrent GBM, the EF-11 trial enrolled 237 patients with confirmed progression and randomized them to receive either TTFields monotherapy or physicians choice best active chemotherapy[74]. Median survival and PFS were comparable between the TTFields arm and chemotherapy. No concerned safety data were documented with ~15% of patients treated with TTFields, experiencing mild to moderate scalp dermatitis, the expected side effect of TTFields. The results of the EF-11 trial confirmed that TTFields therapy was safe and effective and led to the approval in the US of TTFields in the setting of recurrent GBM. With the favorable safety profile of TTFields proven with EF-11, combination with chemotherapy was the next step. This led to EF-14 trial - a phase III randomized trial evaluating TTFields combined with Temozolomide in patients with newly-diagnosed GBM after completion of chemoradiation[75]. A preplanned interim analysis found a significant PFS and OS benefit in favor of TTFields with TMZ. The benefit was sufficient for the independent data and safety monitoring committee to recommend early termination of the trial and eventually led the FDA to expand the approval of TTFields to include the setting of newly diagnosed GBM.
The safety and efficacy of TTFields in combination with chemotherapy in advanced pancreatic adenocarcinoma was first reported in the PANOVA phase II trial. In this open-label phase II, patients received systemic chemotherapy while wearing a portable medical device pre-programmed to deliver 150 kHz TTFields in two sequential, perpendicular fields’ directions for at least 18 h per day. The primary endpoint was the safety of TTFields in combination with gemcitabine, or gemcitabine plus nab-paclitaxel. The trial had multiple secondary endpoints that included PFS and OS as well as compliance with TTFields therapy. The study enrolled a total of 40 patients. It showed good compliance of 68%-78% of recommended minimal daily exposure. No systemic toxicity was attributed to the electrical field therapy highlighting the remarkable safety profile of this modality. The only additional safety concern was a small amount of grade 3 device-related dermatitis that was easily managed, consistent with clinical experience from GBM. The median PFS for the gemcitabine and nab-paclitaxel TTF cohort was 12.7 months (95%CI: 5.4-NA): 9.3 months in patients with metastatic disease, without being reached in locally advanced patients. PFS at six months was 65%: 50% in metastatic disease and 87.5% in locally advanced patients. Of the evaluable patients, 40% had a partial response, and 47% had stable disease for a disease control rate of 87%. The median OS was not reached, and the 1-year survival rate was 72% (62.5% in metastatic disease and 87.5% in locally advanced disease)[76]. These values compare favorably with the historical control of the gemcitabine nab-paclitaxel regimen (PFS: 5.5, OS: 8.5, 1-Y OS of 35%)[46].
The exciting results of the PANOVA trial led to increased interest in the use of this regimen. Consequently, a phase III PANOVA -3 trial (NCT03377491) is currently enrolling patients. The trial is designed to test the efficacy of adding TTFields to nab-paclitaxel and gemcitabine combination in locally advanced unresectable pancreatic adenocarcinoma (LAPC). The trial is planned to enroll 556 patients with unresectable LAPC (per NCCN guidelines). Patients will be randomized 1:1 to TTFields plus nab-paclitaxel and gemcitabine or nab-paclitaxel and gemcitabine alone. TTFields (150 kHz) will be delivered at least 18 h/day until local disease progression is noted[77].
The results of PANOVA 3 will guide the next step in using TTFields in the unresectable disease and the metastatic settings. Further understanding of the TTFields induced autophagy role as a survival mechanism could potentially lead to combinations with autophagy inhibitors as an anti-resistance combination. Additionally, better delineation of the immunomodulatory effect of TTFields is critical. Immunotherapy had been disappointing in PDAC in multiple trials[78]. Low mutational burden and dense stroma, as well as the immunosuppressive microenvironment are challenges for effective immunotherapy in PDAC[79]. The possibility of TTFields increasing immunogenic cell death, dendritic cell activation, TH1 polarization, and increased leukocyte recruitment opens the door to harness the efficacy of immune checkpoint inhibitors. Concurrently, TTFields investigation in the perioperative setting represents an area of high interest. As detailed above, multiple in vitro models showed a reduction in migration and metastasis. Also, several animal studies demonstrated a lessening in the number of metastatic lesions in treated animals. This theoretical inhibition of metastasis is highly desirable as a concept for PDAC receiving preoperative therapy, mainly because this treatment is continuous rather than the usual pulsed nature of neoadjuvant chemotherapy. TTFields also has a favorable safety profile that allows the incorporation of TTFields with other agents in the neoadjuvant setting, making this strategy highly attractive for a clinical trial. The perioperative utilization of TTFields is expected to come to fruition soon.
CONCLUSIONS
Pancreatic adenocarcinoma is a highly lethal disease. Meaningful improvements in therapy and outcomes have been challenging despite several promising early data on several fronts. Hence, the search continues for innovative, novel, and effective therapeutics.
Intense clinical research efforts are ongoing on several avenues in this disease, with a multitude of promising strategies on the horizon. Advancements in novel cytotoxic chemotherapies, immunotherapy combinations, microenvironment modulation, or targeted therapies, among others, represent the majority of current clinical research. All carry a certain degree of hope and cautious optimism and deserve their time in the spotlight, evident in their dominance in recent clinical research efforts. In this review, we chose to highlight novel research efforts that are not typically in the spotlight - pharmacologic vitamin C and Tumor Treating Fields, both of which have built a growing body of literature supporting their potential to target PDAC. Multiple anti-cancer mechanisms of action have been proposed for each of them, and all align well with pancreas adenocarcinoma serving as a suitable target. They share common flaws, as the reported trials with these agents are all small, underpowered, and preliminary. They also share a similar challenge regarding optimal and convenient administration. Water solubility of vitamin C represents a significant challenge regarding its use as frequent dosing is taxing on patients and staff. Tumor Treating Field battery-powered portable device compliance on a long term basis can likewise be challenging for patients. Despite all of these issues, there is hope and optimism regarding the ongoing trials, as detailed in Table 3. These three innovative therapeutics have shown acceptable safety and tolerability with preliminary signs of encouraging activity. Surely, any successful endeavor is welcome news in the challenging world of pancreatic adenocarcinoma research.
Selected ongoing clinical trials with non-traditional therapeutics in PDAC
Intervention | Trial ID | Investigational arm |
Vitamin C | NCT02905578 | Gemcitabine, nab-paclitaxel and intravenous vitamin C TIW |
NCT03797443 | Gemcitabine + Nab-Paclitaxel + Cisplatin + IV Vitamin C BIW | |
NCT03146962 | Cohort B: IV Vitamin C 4 days/week | |
TTFields | NCT03377491 | Gemcitabine+ nab- paclitaxel + TTFields |
DECLARATIONS
Authors’ contributionsConception of review scope: Abushahin L, Noonan A
Performed data acquisition and critical analysis: Abushahin L, Jones T
Made substantial contributions to the manuscript writing: Abushahin L, Noonan A, Jones T, Song J, Williams T, Shahzad H
Availability of data and materialsNot applicable.
Financial support and sponsorshipNone.
Conflicts of interestAll authors declared that there are no conflicts of interest.
Ethical approval and consent to participateNot applicable.
Consent for publicationNot applicable.
Copyright© The Author(s) 2021.
REFERENCES
1. Ferlay J, Colombet M, Soerjomataram I, et al. Estimating the global cancer incidence and mortality in 2018: GLOBOCAN sources and methods. Int J Cancer 2019;144:1941-53.
3. Wagner M, Redaelli C, Lietz M, Seiler CA, Friess H, Büchler MW. Curative resection is the single most important factor determining outcome in patients with pancreatic adenocarcinoma. Br J Surg 2004;91:586-94.
4. Conroy T, Hammel P, Hebbar M, et al. FOLFIRINOX or Gemcitabine as Adjuvant Therapy for Pancreatic Cancer. N Engl J Med 2018;379:2395-406.
5. Neoptolemos JP, Palmer DH, Ghaneh P, et al. Comparison of adjuvant gemcitabine and capecitabine with gemcitabine monotherapy in patients with resected pancreatic cancer (ESPAC-4): a multicentre, open-label, randomised, phase 3 trial. Lancet 2017;389:1011-24.
6. Oettle H, Neuhaus P, Hochhaus A, et al. Adjuvant chemotherapy with gemcitabine and long-term outcomes among patients with resected pancreatic cancer: the CONKO-001 randomized trial. J AM MED ASSOC 2013;310:1473-81.
7. Rahib L, Fleshman JM, Matrisian LM, Berlin JD. Evaluation of pancreatic cancer clinical trials and benchmarks for clinically meaningful future trials: a systematic review. JAMA Oncol 2016;2:1209-16.
8. Li Y, Schellhorn HE. New developments and novel therapeutic perspectives for vitamin C. J Nutr 2007;137:2171-84.
9. Padayatty SJ, Riordan HD, Hewitt SM, Katz A, Hoffer LJ, Levine M. Intravenously administered vitamin C as cancer therapy: three cases. CAN MED ASSOC J 2006;174:937-42.
10. Corti A, Casini AF, Pompella A. Cellular pathways for transport and efflux of ascorbate and dehydroascorbate. Arch Biochem Biophys 2010;500:107-15.
11. Vera JC, Rivas CI, Zhang RH, Farber CM, Golde DW. Human HL-60 myeloid leukemia cells transport dehydroascorbic acid via the glucose transporters and accumulate reduced ascorbic acid. Blood 1994;84:1628-34.
12. Padayatty SJ, Levine M. Vitamin C: the known and the unknown and Goldilocks. Oral Dis 2016;22:63-93.
13. Ngo B, Van Riper JM, Cantley LC, Yun J. Targeting cancer vulnerabilities with high-dose vitamin C. Nat Rev Cancer 2019;19:271-82.
14. Vera JC, Rivas CI, Fischbarg J, Golde DW. Mammalian facilitative hexose transporters mediate the transport of dehydroascorbic acid. Nature 1993;364:79-82.
16. Cameron E, Pauling L. Ascorbic acid and the glycosaminoglycans. An orthomolecular approach to cancer and other diseases. Oncology 1973;27:181-92.
18. Cameron E, Campbell A. The orthomolecular treatment of cancer. II. Clinical trial of high-dose ascorbic acid supplements in advanced human cancer. Chem Biol Interact 1974;9:285-315.
19. Cameron E, Campbell A, Jack T. The orthomolecular treatment of cancer. III. Reticulum cell sarcoma: double complete regression induced by high-dose ascorbic acid therapy. Chem Biol Interact 1975;11:387-93.
20. Cameron E, Pauling L. Supplemental ascorbate in the supportive treatment of cancer: reevaluation of prolongation of survival times in terminal human cancer. Proc Natl Acad Sci U S A 1978;75:4538-42.
21. Creagan ET, Moertel CG, O'Fallon JR, et al. Failure of high-dose vitamin C (ascorbic acid) therapy to benefit patients with advanced cancer. A controlled trial. N Engl J Med 1979;301:687-90.
22. Moertel CG, Fleming TR, Creagan ET, Rubin J, O'Connell MJ, Ames MM. High-dose vitamin C versus placebo in the treatment of patients with advanced cancer who have had no prior chemotherapy. A randomized double-blind comparison. N Engl J Med 1985;312:137-41.
23. Padayatty SJ, Levine M. New insights into the physiology and pharmacology of vitamin C. CMAJ 2001;164:353-5.
24. Chen Q, Espey MG, Sun AY, et al. Pharmacologic doses of ascorbate act as a prooxidant and decrease growth of aggressive tumor xenografts in mice. Proc Natl Acad Sci U S A 2008;105:11105-9.
25. Waddell N, Pajic M, Patch AM, et al. Whole genomes redefine the mutational landscape of pancreatic cancer. Nature 2015;518:495-501.
26. Polireddy K, Dong R, Reed G, et al. High dose parenteral ascorbate inhibited pancreatic cancer growth and metastasis: mechanisms and a phase I/IIa study. Sci Rep 2017:7,17188.
27. Verrax J, Calderon PB. Pharmacologic concentrations of ascorbate are achieved by parenteral administration and exhibit antitumoral effects. Free Radic Biol Med 2009;47:32-40.
28. Nauman G, Gray JC, Parkinson R, Levine M, Paller CJ. Systematic review of intravenous ascorbate in cancer clinical trials. Antioxidants (Basel) 2018;7:89.
29. Chen Q, Espey MG, Krishna MC, et al. Pharmacologic ascorbic acid concentrations selectively kill cancer cells: action as a pro-drug to deliver hydrogen peroxide to tissues. Proc Natl Acad Sci U S A 2005;102:13604-9.
30. Buettner GR. The pecking order of free radicals and antioxidants: lipid peroxidation, alpha-tocopherol, and ascorbate. Arch Biochem Biophys 1993;300:535-43.
31. Lane DJ, Richardson DR. The active role of vitamin C in mammalian iron metabolism: much more than just enhanced iron absorption! Free Radic Biol Med 2014;75:69-83.
32. Mastrangelo D, Pelosi E, Castelli G, Lo-Coco F, Testa U. Mechanisms of anti-cancer effects of ascorbate: cytotoxic activity and epigenetic modulation. Blood Cells Mol Dis 2018;69:57-64.
33. Klingelhoeffer C, Kämmerer U, Koospal M, et al. Natural resistance to ascorbic acid induced oxidative stress is mainly mediated by catalase activity in human cancer cells and catalase-silencing sensitizes to oxidative stress. BMC Complement Altern Med 2012;12:61.
34. Doskey CM, Buranasudja V, Wagner BA, et al. Tumor cells have decreased ability to metabolize H2O2: Implications for pharmacological ascorbate in cancer therapy. Redox Biol 2016;10:274-84.
35. Yun J, Mullarky E, Lu C, et al. Vitamin C selectively kills KRAS and BRAF mutant colorectal cancer cells by targeting GAPDH. Science 2015;350:1391-6.
36. Semenza GL. Pharmacologic targeting of hypoxia-inducible factors. Annu Rev Pharmacol Toxicol 2019;59:379-403.
38. Vissers MCM, Das AB. Potential mechanisms of action for vitamin C in cancer: reviewing the evidence. Front Physiol 2018;9:809.
39. Ye HL, Zhou QB, Zheng SY, et al. Tumor-associated macrophages promote progression and the Warburg effect via CCL18/NF-kB/VCAM-1 pathway in pancreatic ductal adenocarcinoma. Cell Death Dis 2018;9:453.
40. Pfeifhofer-Obermair C, Tymoszuk P, Petzer V, Weiss G, Nairz M. Iron in the tumor microenvironment-connecting the dots. Front Oncol 2018;8:549.
41. Jin X, Dai L, Ma YL, Wang J, Liu Z. Implications of HIF-1alpha in the tumorigenesis and progression of pancreatic cancer. Cancer Cell Int 2020;20:273.
42. Riordan HD, Riordan NH, Jackson JA, et al. Intravenous vitamin C as a chemotherapy agent: a report on clinical cases. P R Health Sci J 2004;23:115-8.
43. Hoffer LJ, Levine M, Assouline S, et al. Phase I clinical trial of i.v. ascorbic acid in advanced malignancy. Ann Oncol 2008;19:1969-74.
44. Monti DA, Mitchell E, Bazzan AJ, et al. Phase I evaluation of intravenous ascorbic acid in combination with gemcitabine and erlotinib in patients with metastatic pancreatic cancer. PLoS One 2012;7:e29794.
45. Welsh JL, Wagner BA, van't Erve TJ, et al. Pharmacological ascorbate with gemcitabine for the control of metastatic and node-positive pancreatic cancer (PACMAN): results from a phase I clinical trial. Cancer Chemother Pharmacol 2013;71:765-75.
46. Von Hoff DD, Ervin T, Arena FP, et al. Increased survival in pancreatic cancer with nab-paclitaxel plus gemcitabine. N Engl J Med 2013;369:1691-703.
47. Levine M, Wang Y, Padayatty SJ, Morrow J. A new recommended dietary allowance of vitamin C for healthy young women. Proc Natl Acad Sci U S A 2001;98:9842-6.
48. Pless M, Weinberg U. Tumor treating fields: concept, evidence and future. Expert Opin Investig Drugs 2011;20:1099-106.
49. Goldberg SN, Gazelle GS, Mueller PR. Thermal ablation therapy for focal malignancy: a unified approach to underlying principles, techniques, and diagnostic imaging guidance. AJR Am J Roentgenol 2000;174:323-31.
50. Tofani S, Barone D, Cintorino M, et al. Static and ELF magnetic fields induce tumor growth inhibition and apoptosis. Bioelectromagnetics 2001;22:419-28.
51. Kirson ED, Gurvich Z, Schneiderman R, et al. Disruption of cancer cell replication by alternating electric fields. Cancer Res 2004;64:3288-95.
52. Kirson ED, Dbalý V, Tovarys F, et al. Alternating electric fields arrest cell proliferation in animal tumor models and human brain tumors. Proc Natl Acad Sci U S A 2007;104:10152-7.
53. Giladi M, Schneiderman RS, Voloshin T, et al. Mitotic spindle disruption by alternating electric fields leads to improper chromosome segregation and mitotic catastrophe in cancer cells. Sci Rep 2015;5:18046.
54. Gera N, Yang A, Holtzman TS, Lee SX, Wong ET, Swanson KD. Tumor treating fields perturb the localization of septins and cause aberrant mitotic exit. PLoS One 2015;10:e0125269.
55. Tuszynski JA, Wenger C, Friesen DE, Preto J. An overview of sub-cellular mechanisms involved in the action of TTFields. Int J Environ Res Public Health 2016;13:1128.
56. Wenger C, Miranda PC, Salvador R, et al. A review on Tumor-Treating Fields (TTFields): clinical implications inferred from computational modeling. IEEE Rev Biomed Eng 2018;11:195-207.
57. Kessler AF, Frömbling GE, Gross F, et al. Effects of tumor treating fields (TTFields) on glioblastoma cells are augmented by mitotic checkpoint inhibition. Cell Death Discov 2018;4:12.
58. Voloshin T, Munster M, Blatt R, et al. Alternating electric fields (TTFields) in combination with paclitaxel are therapeutically effective against ovarian cancer cells in vitro and in vivo. Int J Cancer 2016;139:2850-8.
59. Shteingauz A, Porat Y, Voloshin T, et al. AMPK-dependent autophagy upregulation serves as a survival mechanism in response to Tumor Treating Fields (TTFields). Cell Death Dis 2018;9:1074.
60. Karanam NK, Ding LH, Aroumougame A, Story MD. Tumor treating fields cause replication stress and interfere with DNA replication fork maintenance: implications for cancer therapy. Transl Res 2020;217:33-46.
61. Berkelmann L, Bader A, Meshksar S, et al. Tumour-treating fields (TTFields): Investigations on the mechanism of action by electromagnetic exposure of cells in telophase/cytokinesis. Sci Rep 2019;9:7362.
62. Voloshin T, Kaynan N, Davidi S, et al. Tumor-treating fields (TTFields) induce immunogenic cell death resulting in enhanced antitumor efficacy when combined with anti-PD-1 therapy. Cancer Immunol Immunother 2020;69:1191-204.
63. Senovilla L, Vitale I, Martins I, et al. An immunosurveillance mechanism controls cancer cell ploidy. Science 2012;337:1678-84.
64. Stingele S, Stoehr G, Storchova Z. Activation of autophagy in cells with abnormal karyotype. Autophagy 2013;9:246-8.
65. Elliott MR, Chekeni FB, Trampont PC, et al. Nucleotides released by apoptotic cells act as a find-me signal to promote phagocytic clearance. Nature 2009;461:282-6.
66. Messmer D, Yang H, Telusma G, et al. High mobility group box protein 1: an endogenous signal for dendritic cell maturation and Th1 polarization. J Immunol 2004;173:307-13.
67. Scaffidi P, Misteli T, Bianchi ME. Release of chromatin protein HMGB1 by necrotic cells triggers inflammation. Nature 2002;418:191-5.
68. Schneiderman R, Giladi M, Zeevi E, et al. Tumor treating fields affect invasion properties and cell morphology of various cancer cells. Neuro-Oncology 2018;20:iii282.
69. Schneiderman RS, Giladi M, Zeevi E, et al. Tumor treating fields (Ttfields) inhibit cancer cell migration and invasion by inducing reorganization of the actin cytoskeleton and formation of cell adhesions. Neuro-Oncology 2018;20:vi30.
70. Voloshin T, Schneiderman RS, Volodin A, et al. Tumor treating fields (TTFields) hinder cancer cell motility through regulation of microtubule and acting dynamics. Cancers (Basel) 2020;12:3016.
71. Garg AA, Jones TH, Moss SM, et al. Electromagnetic fields alter the motility of metastatic breast cancer cells. Commun Biol 2019;2:303.
72. Giladi M, Schneiderman RS, Porat Y, et al. Mitotic disruption and reduced clonogenicity of pancreatic cancer cells in vitro and in vivo by tumor treating fields. Pancreatology 2014;14:54-63.
73. Rominiyi O, Vanderlinden A, Clenton SJ, Bridgewater C, Al-Tamimi Y, Collis SJ. Tumour treating fields therapy for glioblastoma: current advances and future directions. Br J Cancer 2021;124:697-709.
74. Stupp R, Wong ET, Kanner AA, et al. NovoTTF-100A versus physician's choice chemotherapy in recurrent glioblastoma: a randomised phase III trial of a novel treatment modality. Eur J Cancer 2012;48:2192-202.
75. Kesari S, Ram Z. EF-14 Trial Investigators. Tumor-treating fields plus chemotherapy versus chemotherapy alone for glioblastoma at first recurrence: a post hoc analysis of the EF-14 trial. CNS Oncol 2017;6:185-93.
76. Rivera F, Benavides M, Gallego J, Guillen-Ponced C, Lopez-Martine J, Küng M. Tumor treating fields in combination with gemcitabine or gemcitabine plus nab-paclitaxel in pancreatic cancer: results of the PANOVA phase 2 study. Pancreatology 2019;19:64-72.
77. Weinberg U, Giladi M, Bomzon Z, Kirson E. PANOVA-3: a phase III study of tumor treating fields with nabpaclitaxel and gemcitabine for front-line treatment of locally advanced pancreatic adenocarcinoma (LAPC). JCO 2019;37:TPS469.
78. Renouf DJ, Knox JJ, Kavan P, et al. The canadian cancer trials group PA.7 trial: results of a randomized phase II study of gemcitabine (GEM) and nab-paclitaxel (Nab-P) vs GEM, nab-P, durvalumab (D) and tremelimumab (T) as first line therapy in metastatic pancreatic ductal adenocarcinoma (mPDAC). Annals of Oncology 2020;31:S1195.
Cite This Article

How to Cite
Download Citation
Export Citation File:
Type of Import
Tips on Downloading Citation
Citation Manager File Format
Type of Import
Direct Import: When the Direct Import option is selected (the default state), a dialogue box will give you the option to Save or Open the downloaded citation data. Choosing Open will either launch your citation manager or give you a choice of applications with which to use the metadata. The Save option saves the file locally for later use.
Indirect Import: When the Indirect Import option is selected, the metadata is displayed and may be copied and pasted as needed.
About This Article
Copyright
Data & Comments
Data
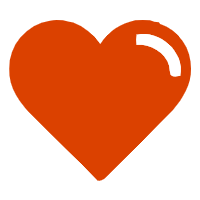
Comments
Comments must be written in English. Spam, offensive content, impersonation, and private information will not be permitted. If any comment is reported and identified as inappropriate content by OAE staff, the comment will be removed without notice. If you have any queries or need any help, please contact us at [email protected].