Biomedical DNA hydrogels
Abstract
Due to considerable progress in DNA nanotechnology, DNA is gaining significant attention as a programmable building block for the next generation of soft biomaterials. DNA has been used as either a single component to form all-DNA hydrogels or a crosslinker or functional entity to form hybrid DNA hydrogels through physical interactions or chemical reactions. The formed hydrogels exhibit adequate biocompatibility, convenient programmability, tunable multifunctionality and the capability of precise molecular recognition, making them an irreplaceable polymeric platform for interfacing with biology. Responsive DNA hydrogels that are prepared through the hybridization of DNA sticky ends, the formation of i-motifs, enzymatic ligation and enzymatic polymerization are commonly reported nowadays and can undergo disassembly induced by various triggers, including alterations in ionic strength, pH, temperature and biomolecules. These hydrogels are envisioned for applications in drug delivery and biosensing. This perspective assesses the most recent and important developments in this emerging class of biomedically useful DNA hydrogels.
Keywords
INTRODUCTION
DNA is made of nucleotide monomers that are covalently linked to each other by phosphodiester bonds. DNA displays excellent mechanical rigidity and can thus precisely position the tethered nanoparticles[1] and proteins[2] along the rigid rod spacer. Furthermore, its complex assemblies even allow for the spatial arrangement of nanoparticles[3] and proteins[4]. DNA can also exhibit dynamic and adaptable properties after specifically interacting with other molecules and can therefore exhibit a wide scope of external biological signals[5] and manipulate target cells[6] in a dynamic and reversible fashion. Moreover, DNA can be processed precisely by highly specific enzymes, such as ligases, polymerases, endonucleases and exonucleases[7-9]. While the DNA double helix in DNA materials shows a distinct thermally triggered dissociation behavior, its thermal stability can be improved through intercalating agents[10] or covalent crosslinking[11]. Modern DNA synthesis techniques, such as automated solid-phase synthesis, polymerase chain reaction and production in microorganisms, enable rapid preparation of any desired DNA sequence in a large quantity[12,13]. This has further prompted a materials revolution by implementing DNA as a structural or functional entity in biomaterials design. The specific and predictive interactions relying on the Watson-Crick base pairing mechanism provide excellent modeling capabilities and design flexibility, thereby facilitating convenient programming and spontaneous self-assembly of diverse two- and three-dimensional objects with high complexity[14].
Accordingly, DNA has stimulated new directions in soft materials and science[15,16]. In particular, Seeman[17] proposed in 1982 to use DNA as a construction material for assembling geometrically defined objects with nanoscale features, which sets the foundation of an emerging research field, nowadays termed as “structural DNA nanotechnology”[18]. However, since the production of finite DNA nanostructures via structural DNA nanotechnology using DNA tiles remains complicated[19], the development of the “scaffolded DNA origami” technique by Rothemund must be seen as an important milestone. This technique works through predictably folding a circular, single-stranded DNA template into finite, programmable and addressable nanostructures with short, single-stranded DNA staples[20]. Furthermore, DNA is an excellent candidate for the construction of three-dimensional crosslinked networks containing a high amount of water, i.e., supramolecular hydrogels[21]. Unlike many other covalently crosslinked hydrogels derived from synthetic polymers or noncovalently crosslinked hydrogels derived from natural sources, like alginate or gelatin, DNA hydrogels are endowed with desirable features of programmability, stimuli-responsiveness and good biocompatibility by DNA[22]. Therefore, DNA hydrogels are highly promising “smart” platforms in biomedical applications[23].
This perspective focuses on the design principles and different methods used to construct all-DNA and hybrid DNA hydrogels and then highlights their applications in different biomedical applications, in particular, drug delivery and biosensing. This perspective will help to promote the further development of DNA hydrogels and more in-depth studies toward expanding their applications in soft science.
DESIGN AND PREPARATION ASPECTS OF DNA HYDROGELS
Several different design principles have so far been proposed to prepare DNA hydrogels[24-26]. The selected design and preparation methods provide access to customizable DNA hydrogels with unique physiochemical and biological features. In this section, all-DNA hydrogels that consist entirely of DNA sequences and hybrid DNA hydrogels that consist of both DNA sequences and synthetic polymers are introduced.
All-DNA hydrogels
The seminal work on all-DNA hydrogels was carried out by Um et al.[27] using branched DNA building blocks, which were pre-designed to associate with other complementary strands. The gelation of DNA building blocks can be achieved through the hybridization of DNA sticky ends [Figure 1A][28], formation of i-motifs [Figure 1B][29] or enzymatic ligation [Figure 1C][27]. All-DNA hydrogels can also be generated during in situ formation of the building blocks by enzymatic polymerization. For instance, hydrogels can be prepared by a polymerase chain reaction via extending and connecting the forward and reverse, Y-shaped primers that are thermally stabilized by psoralen[30]. Another intriguing example has been demonstrated by using rolling circle amplification to produce extremely long DNA sequences that eventually form gels through physical entanglement [Figure 1D][31].
Figure 1. Self-assembly of all-DNA hydrogels through (A) hybridization of DNA sticky ends[28], (B) formation of i-motifs[29], (C) enzymatic ligation[27] and (D) physical entanglement[31]. (A, B) Reprinted with permission from Refs.[28,29]. Copyright 2009, 2011 Wiley-VCH. (C, D) Reprinted with permission from Refs.[27,31]. Copyright 2006, 2012 Springer Nature.
The building blocks strongly influence the characteristics of resulting all-DNA hydrogels. For instance, physically crosslinked hydrogels made by the hybridization of sequences with complementary sticky ends can undergo revisable sol-gel transitions upon heating[28] or pH alteration[29]. In contrast, covalently crosslinked hydrogels made by enzymatic ligation do not undergo reversible sol-gel transitions[27]. In addition, enzymatic polymerization techniques result in hydrogels with metamaterial properties exhibiting liquid-like properties when taken out of water and solid-like properties when immersed in water[31]. Furthermore, hydrogels with defined geometries in the shape of the letters D, N and A completely deformed after water removal, but returned to their original shapes upon water reintroduction[31].
Hybrid DNA hydrogels
DNA-polymer hybrid hydrogels utilize both the programmable and smart features of DNA components and the chemical stability, flexibility and accessibility of polymers. In 1996, Nagahara reported the first DNA-polymer hybrid hydrogels based on short DNA sequences grafted to a poly(acrylamide) polymer chain[32]. Since then, various DNA-polymer hybrid hydrogels have been prepared with controlled sol-gel transitions. Branched DNA as a structural component enables simultaneous gelation and functionalization of hydrogels. While these DNA linkages typically exhibit multi-responsiveness and thixotropic behavior under heat or in the presence of enzyme, their conjugates, like azobenzene-functionalized linkages, can display light-responsiveness upon alternating irradiation with visible and ultraviolet light, inducing the macroscopic volume changes of the hydrogels [Figure 2A][33]. Logically, semiconducting polymers might be practically explored as a functional unit or scaffold to prepare photosensitizing DNA hydrogels due to the π-electron delocalized backbones they possess[34,35]. Furthermore, multi-responsive hydrogels can be constructed based on DNA linkages by additionally implementing i-motifs [Figure 2B][36], G-quadruplex units[37] or aptamers[38].
Figure 2. (A) Light-responsive hybrid DNA hydrogels based on azobenzene isomerization. Reprinted with permission from Ref.[33]. Copyright 2012, American Chemical Society. (B) pH-responsive hybrid DNA hydrogels based on cyclic switching of i-motifs. Reprinted with permission from Ref.[36]. Copyright 2015 Wiley-VCH. DNA hybrid hydrogels for (C) biomimetic protein release and signal transduction or (D) cell catch and release. (C) Reprinted with permission from Ref.[41]. Copyright 2017, Royal Society of Chemistry. (D) Reprinted with permission from Ref.[42]. Copyright 2012, American Chemical Society.
In contrast, DNA segments have also been explored as functional, bioactive elements to decorate hydrogels for various biomedical applications[39]. The presence of DNA as a bioactive group in these hydrogels typically imparts specific interactions with biomolecules [Figure 2C][40,41] or cells [Figure 2D][42], but does not significantly alter the mechanical properties of the hydrogels. The functionalization of all-DNA hydrogels is straightforward, only requiring the introduction of short DNA tags comprising complementary DNA strands. Alternatively, the functionalization of hydrogels made of synthetic polymers requires the formation of covalent bonds. This is facilitated by phosphoramidite derivatives that provide versatile routes to synthesize chemical group-functionalized oligonucleotides for covalently coupling with polymer chains during or after the gel formation[43-45]. However, a potential drawback of the post-functionalization of hydrogels using DNA is the steric or electrostatic hinderance during infiltration, which is particularly true for larger DNA structures.
BIOMEDICAL APPLICATIONS OF DNA HYDROGELS
The recognition of analytes triggers changes, such as in crosslinking density or mechanical, optical or other properties, in the physicochemical nature of a DNA hydrogel matrix, which can further lead to cargo release for drug delivery or can be transduced into a detectable signal for biosensing[23]. In the following sections, the applications of DNA hydrogels in drug release and biosensing are discussed.
Drug delivery
Conventional chemotherapy based on the systemic injection of small molecule drugs suffers from a lack of specificity toward cancerous cells versus healthy ones, which results in serious side effects. Hydrogel formulations are of particular interest for local and controlled drug delivery to improve drug efficacy and reduce off-target side effects[46]. DNA strands have been extensively explored for enrichment of the anthracycline drug doxorubicin via intercalation into GC/CG-rich sequences. By further incorporating gold nanoparticles, DNA hydrogels allowed for the disassembly of the whole construct and subsequent drug release through a photothermal effect[47]. The anticancer efficacy of this system was found to be improved through the synergy between photothermal therapy and chemotherapy with a reduced dosage of either laser power or drug dosage.
In addition to physical triggers, chemical triggers are attractive for drug release depending on the tumor microenvironment. For instance, adenosine triphosphate (ATP) is overexpressed in cancer cells, making it an interesting trigger for site-selective release systems. An ATP-responsive DNA hydrogel was constructed by incorporating ligand-specific aptamer sequences into the gel scaffold. This hydrogel was able to release the cargo in the presence of ATP due to the dissociation of the gel matrix induced by interactions between the ATP and aptamer [Figure 3A][48]. Furthermore, with delicate design, the pH-sensitive bridges in hydrogels can dissociate via the formation of i-motif structures under tumor acidic conditions, which results in cargo release by increasing the fluidity of gel matrix[48].
Figure 3. Drug delivery applications of (A) DNA hydrogels and (B) patterned hydrogel films. (A) Reprinted with permission from Ref.[48]. Copyright 2017, Royal Society of Chemistry. (B) Reprinted with permission from Ref.[52]. Copyright 2018, American Chemical Society. Biosensing applications of (C) bioimprinted aptamer hydrogels and (D) flexible electrochemical sensors. (C) Reprinted with permission from Ref.[61]. Copyright 2013, American Chemical Society. (D) Reprinted with permission from Ref.[67]. Copyright 2018, Wiley-VCH.
The aptamer-containing hydrogels are particularly useful for the delivery and sustained release of bioactive molecules to specific cells or tissues. The stored cargos in hydrogels can be released either periodically[41] or sustainably[49] through external[50] and mechanical triggers[51] by introducing aptamers that specifically bind to the corresponding ingredients. Based on these, the principle of sequential protein release from DNA hydrogels was further expanded by spatially controlling the immobilization of proteins through a combination of photo-patterning [Figure 3B][52]. It has been demonstrated that VEGF and PDGF-ββ proteins bind to the corresponding aptamers that are incorporated into the patterned hydrogels, giving rise to both spatial and temporal control over the release of two separate proteins using the displacement strand strategy. Most strikingly, the aptamer technology can be employed for simultaneous cancer cell recognition and stimuli-responsive drug release. This has been achieved by the incorporation of aptamers that bind receptors, like nucleolin, overexpressed in tumor cells in biological environments[53].
Furthermore, drug-integrated DNA can be considered as an efficient prodrug to overcome the limitations and severe side effects of systemic drug injection. Cytotoxic nucleoside analogs, such as fluoroxidine, have been covalently integrated into DNA oligonucleotides via solid-phase synthesis and then subsequently assembled into DNA hydrogels. These hydrogels acted as novel Trojan Horses, enabling rapid cellular uptake, effective drug release in the presence of DNases and eventually an enhanced anticancer effect[54].
Biosensing
The detection of various analytes in high sensitivity is vital for many biomedical fields, including point-of-care testing, clinical diagnosis and personalized healthcare[55-57]. The typical design strategies of hydrogel biosensors employ an analyte-binding DNA as the sensor. The hydrogels display changes in volume, mass, color or mechanical properties when the functional DNA unit dissociates only after recognition of the respective targets[58,59]. Different DNA hydrogels have been designed to detect ions[60], small molecules[38], proteins[61] or viruses[62].
DNA hydrogels even enable visualization of the readout via the naked eye in certain cases and therefore do not need a sophisticated equipment[63,64]. While colorimetry is the commonly used detection method of DNA hydrogel biosensors, it often provides semiquantitative results and relatively low sensitivity[65]. A molecular imprinting approach can overcome these mentioned limitations through macroscopic volumetric shrinkage in the presence of targets. For example, DNA hydrogels were formed by the polymerization of bifunctional aptamer-protein complexes, so that proteins, like thrombin or PDGF-ββ, were imprinted into the hydrogels. The resulting hydrogels showed remarkable sensitivity down to femtomolar concentrations of proteins [Figure 3C][61]. Recently, surface-immobilized DNA hydrogels were developed as printable sensors for applications in the fields of clinical diagnosis and personalized medicine[65,66]. Such DNA hydrogels can be generally synthesized on a solid surface via a surficial primer-induced strategy. After loading horseradish peroxidase and bilirubin oxidase enzymes, DNA hydrogels have been explored as stable catalytic systems, allowing for the colorimetric or electrochemical detection of hydrogen peroxide and bilirubin, respectively, with high sensitivity and in a recyclable manner[66].
The immense potential of catalytic DNA hydrogels for next-generation biosensors and bioelectronics has been highlighted by the in situ fabrication of a flexible glucose biosensor [Figure 3D][67]. This biosensor was fabricated by loading the glucose oxidase into a DNA hydrogel, followed by printing into a flexible electrochemical electrode. This detection system revealed excellent electrochemical properties and long-term cycling stability. The integration of DNA hydrogels into surface biosensing devices could thereby broaden hydrogel applications. However, the development of biosensing devices that have the ability to accurately detect and quantify trace amounts of analytes in complex body fluids still represents a significant challenge. Furthermore, DNA hydrogel biosensors are quite costly, thereby hampering their real-world applications. Therefore, either the production costs have to be substantially lowered or the reusability of these sensors needs to be significantly improved. It is anticipated that if the abovementioned challenges can be addressed, DNA hydrogel biosensors will offer great potential as implantable sensors for in vivo diagnostics or even be used as a powerful toolbox for future personalized medicine.
CONCLUSION AND OUTLOOK
DNA is gaining significant attention as a programmable building block, which provides unique characteristics that have propelled the development of various DNA-based hydrogels accessible for exciting biomedical applications, in particular, drug delivery and biosensing. These examples only signify the beginning of a much larger biomedical field in the future. For example, DNA hydrogels have been recently explored for new immunomodulatory treatment and cell-based approaches. For the former case, DNA hydrogels that programmed with rich CpG sequences and incorporated with certain antigens have been explored as an injectable DNA hydrogel vaccine for cancer immunotherapy by causing strong immune responses[68]. For the latter case, DNA hydrogel-based cell envelop and separation strategies have been reported through crosslinking of cell-anchored, ultralong DNA chains produced via RCA, allowing for fishing stem cells or T lymphocytes from tens of thousands of nontarget cells[69,70]. Moreover, hybrid DNA hydrogels can now be also referred to inorganic nanoparticle-incorporated DNA hydrogels that have been synthesized to tune the mechanical, conductive and optical properties of hydrogels, as well as to instruct multiple cell behaviors[71-77].
Even though DNA hydrogels have seen tremendous progress, several challenges need to be addressed before real-world applications. First, the nuclease resistance of DNA hydrogels under physicochemical and biological conditions needs to be improved, since it will be critical to maintain the designed structures and mechanical properties for in vivo applications. Second, the cost for scaling up DNA hydrogels needs to be reduced regarding the clinical translation. We can foresee that DNA hydrogels would improve human health once those remaining challenges are addressed by combining possible scalable and cost-efficient methods of biotechnological “mass production”[13], liquid-phase oligonucleotide synthesis[78] and DNAzyme-catalyzed production[79].
DECLARATIONS
Authors’ contributionsThe author contributed solely to the article.
Availability of data and materialsNot applicable.
Financial support and sponsorshipThe project was supported by the National Natural Science Foundation of China (52103181) and the Fundamental Research Funds for the Central Universities, China (22120210364).
Conflicts of interestThe author declared that there are no conflicts of interest.
Ethical approval and consent to participateNot applicable.
Consent for publicationNot applicable.
Copyright© The Author(s) 2022.
REFERENCES
1. Niemeyer CM, Ceyhan B, Hazarika P. Oligofunctional DNA-gold nanoparticle conjugates. Angew Chem Int Ed Engl 2003;42:5766-70.
2. Erkelenz M, Kuo CH, Niemeyer CM. DNA-mediated assembly of cytochrome P450 BM3 subdomains. J Am Chem Soc 2011;133:16111-8.
3. Zhou C, Duan X, Liu N. DNA-nanotechnology-enabled chiral plasmonics: from static to dynamic. Acc Chem Res 2017;50:2906-14.
4. Stephanopoulos N. Hybrid nanostructures from the self-assembly of proteins and DNA. Chem 2020;6:364-405.
5. Hu Y. Self-assembly of DNA molecules: towards DNA nanorobots for biomedical applications. Cyborg and Bionic Systems 2021;2021:1-3.
6. Freeman R, Stephanopoulos N, Álvarez Z, et al. Instructing cells with programmable peptide DNA hybrids. Nat Commun 2017;8:15982.
8. Roh YH, Ruiz RC, Peng S, Lee JB, Luo D. Engineering DNA-based functional materials. Chem Soc Rev 2011;40:5730-44.
9. Veneziano R, Ratanalert S, Zhang K, et al. Designer nanoscale DNA assemblies programmed from the top down. Science 2016;352:1534.
10. Stafforst T, Hilvert D. Photolyase-like repair of psoralen-crosslinked nucleic acids. Angew Chem Int Ed Engl 2011;50:9483-6.
11. Rajendran A, Endo M, Katsuda Y, Hidaka K, Sugiyama H. Photo-cross-linking-assisted thermal stability of DNA origami structures and its application for higher-temperature self-assembly. J Am Chem Soc 2011;133:14488-91.
12. Kosuri S, Church GM. Large-scale de novo DNA synthesis: technologies and applications. Nat Methods 2014;11:499-507.
13. Praetorius F, Kick B, Behler KL, Honemann MN, Weuster-Botz D, Dietz H. Biotechnological mass production of DNA origami. Nature 2017;552:84-7.
15. Hu Y, Niemeyer CM. From DNA nanotechnology to material systems engineering. Adv Mater 2019;31:e1806294.
16. Han J, Guo Y, Wang H, Zhang K, Yang D. Sustainable bioplastic made from biomass DNA and ionomers. J Am Chem Soc 2021;143:19486-97.
19. Liu Y, Ke Y, Yan H. Self-assembly of symmetric finite-size DNA nanoarrays. J Am Chem Soc 2005;127:17140-1.
21. Li F, Tang J, Geng J, Luo D, Yang D. Polymeric DNA hydrogel: design, synthesis and applications. Prog Polym Sci 2019;98:101163.
22. Shahbazi M, Bauleth-ramos T, Santos HA. DNA hydrogel assemblies: bridging synthesis principles to biomedical applications. Adv Therap 2018;1:1800042.
23. Li J, Mo L, Lu CH, Fu T, Yang HH, Tan W. Functional nucleic acid-based hydrogels for bioanalytical and biomedical applications. Chem Soc Rev 2016;45:1410-31.
24. Wang D, Hu Y, Liu P, Luo D. Bioresponsive DNA hydrogels: beyond the conventional stimuli responsiveness. Acc Chem Res 2017;50:733-9.
25. Kahn JS, Hu Y, Willner I. Stimuli-responsive DNA-based hydrogels: from basic principles to applications. Acc Chem Res 2017;50:680-90.
26. Shao Y, Jia H, Cao T, Liu D. Supramolecular hydrogels based on DNA self-assembly. Acc Chem Res 2017;50:659-68.
27. Um SH, Lee JB, Park N, Kwon SY, Umbach CC, Luo D. Enzyme-catalysed assembly of DNA hydrogel. Nat Mater 2006;5:797-801.
28. Xing Y, Cheng E, Yang Y, et al. Self-assembled DNA hydrogels with designable thermal and enzymatic responsiveness. Adv Mater 2011;23:1117-21.
29. Cheng E, Xing Y, Chen P, et al. A pH-triggered, fast-responding DNA hydrogel. Angew Chem Int Ed Engl 2009;48:7660-3.
30. Hartman MR, Yang D, Tran TN, et al. Thermostable branched DNA nanostructures as modular primers for polymerase chain reaction. Angew Chem Int Ed Engl 2013;52:8699-702.
31. Lee JB, Peng S, Yang D, et al. A mechanical metamaterial made from a DNA hydrogel. Nat Nanotechnol 2012;7:816-20.
32. Nagahara S, Matsuda T. Hydrogel formation via hybridization of oligonucleotides derivatized in water-soluble vinyl polymers. Polymer Gels and Networks 1996;4:111-27.
33. Peng L, You M, Yuan Q, et al. Macroscopic volume change of dynamic hydrogels induced by reversible DNA hybridization. J Am Chem Soc 2012;134:12302-7.
34. Li J, Pu K. Semiconducting polymer nanomaterials as near-infrared photoactivatable protherapeutics for cancer. Acc Chem Res 2020;53:752-62.
35. Li J, Duan H, Pu K. Nanotransducers for near-infrared photoregulation in biomedicine. Adv Mater 2019;31:e1901607.
36. Guo W, Lu CH, Orbach R, et al. pH-stimulated DNA hydrogels exhibiting shape-memory properties. Adv Mater 2015;27:73-8.
37. Lu CH, Qi XJ, Orbach R, et al. Switchable catalytic acrylamide hydrogels cross-linked by hemin/G-quadruplexes. Nano Lett 2013;13:1298-302.
38. Yang H, Liu H, Kang H, Tan W. Engineering target-responsive hydrogels based on aptamer-target interactions. J Am Chem Soc 2008;130:6320-1.
39. Battig MR, Soontornworajit B, Wang Y. Programmable release of multiple protein drugs from aptamer-functionalized hydrogels via nucleic acid hybridization. J Am Chem Soc 2012;134:12410-3.
40. Soontornworajit B, Zhou J, Shaw MT, Fan TH, Wang Y. Hydrogel functionalization with DNA aptamers for sustained PDGF-BB release. Chem Commun (Camb) 2010;46:1857-9.
41. Lai J, Li S, Shi X, et al. Displacement and hybridization reactions in aptamer-functionalized hydrogels for biomimetic protein release and signal transduction. Chem Sci 2017;8:7306-11.
42. Zhang Z, Chen N, Li S, Battig MR, Wang Y. Programmable hydrogels for controlled cell catch and release using hybridized aptamers and complementary sequences. J Am Chem Soc 2012;134:15716-9.
43. Li S, Gaddes ER, Chen N, Wang Y. Molecular encryption and reconfiguration for remodeling of dynamic hydrogels. Angew Chem Int Ed Engl 2015;54:5957-61.
44. Huang Y, Ma Y, Chen Y, et al. Target-responsive DNAzyme cross-linked hydrogel for visual quantitative detection of lead. Anal Chem 2014;86:11434-9.
45. Dave N, Chan MY, Huang PJ, Smith BD, Liu J. Regenerable DNA-functionalized hydrogels for ultrasensitive, instrument-free mercury(II) detection and removal in water. J Am Chem Soc 2010;132:12668-73.
47. Song J, Hwang S, Im K, et al. Light-responsible DNA hydrogel-gold nanoparticle assembly for synergistic cancer therapy. J Mater Chem B 2015;3:1537-43.
48. Liao WC, Lilienthal S, Kahn JS, et al. pH- and ligand-induced release of loads from DNA-acrylamide hydrogel microcapsules. Chem Sci 2017;8:3362-73.
49. Soontornworajit B, Zhou J, Zhang Z, Wang Y. Aptamer-functionalized in situ injectable hydrogel for controlled protein release. Biomacromolecules 2010;11:2724-30.
50. Soontornworajit B, Zhou J, Snipes MP, Battig MR, Wang Y. Affinity hydrogels for controlled protein release using nucleic acid aptamers and complementary oligonucleotides. Biomaterials 2011;32:6839-49.
51. Stejskalová A, Oliva N, England FJ, Almquist BD. Biologically inspired, cell-selective release of aptamer-trapped growth factors by traction forces. Adv Mater 2019;31:e1806380.
52. Zhang Z, Liu C, Yang C, et al. Aptamer-patterned hydrogel films for spatiotemporally programmable capture and release of multiple proteins. ACS Appl Mater Interfaces 2018;10:8546-54.
53. Wang Z, Xia J, Cai F, et al. Aptamer-functionalized hydrogel as effective anti-cancer drugs delivery agents. Colloids Surf B Biointerfaces 2015;134:40-6.
54. Ma Y, Liu H, Mou Q, Yan D, Zhu X, Zhang C. Floxuridine-containing nucleic acid nanogels for anticancer drug delivery. Nanoscale 2018;10:8367-71.
55. Zhu Z, Guan Z, Jia S, et al. Au@Pt nanoparticle encapsulated target-responsive hydrogel with volumetric bar-chart chip readout for quantitative point-of-care testing. Angew Chem Int Ed Engl 2014;53:12503-7.
56. Wei X, Tian T, Jia S, et al. Microfluidic distance readout sweet hydrogel integrated paper-based analytical device (μDiSH-PAD) for visual quantitative point-of-care testing. Anal Chem 2016;88:2345-52.
57. Wang C, He K, Li J, Chen X. Conformal electrodes for on-skin digitalization. SmartMat 2021;2:252-62.
58. Jonášová EP, Stokke BT. Bioresponsive DNA-co-polymer hydrogels for fabrication of sensors. Curr Opin Colloid Interface Sci 2016;26:1-8.
59. Mao Y, Li J, Yan J, et al. A portable visual detection method based on a target-responsive DNA hydrogel and color change of gold nanorods. Chem Commun (Camb) 2017;53:6375-8.
60. Lin H, Zou Y, Huang Y, et al. DNAzyme crosslinked hydrogel: a new platform for visual detection of metal ions. Chem Commun (Camb) 2011;47:9312-4.
61. Bai W, Gariano NA, Spivak DA. Macromolecular amplification of binding response in superaptamer hydrogels. J Am Chem Soc 2013;135:6977-84.
62. Bai W, Spivak DA. A double-imprinted diffraction-grating sensor based on a virus-responsive super-aptamer hydrogel derived from an impure extract. Angew Chem Int Ed Engl 2014;53:2095-8.
63. Ma Y, Mao Y, An Y, et al. Target-responsive DNA hydrogel for non-enzymatic and visual detection of glucose. Analyst 2018;143:1679-84.
64. Zhu Z, Wu C, Liu H, et al. An aptamer cross-linked hydrogel as a colorimetric platform for visual detection. Angew Chem Int Ed Engl 2010;49:1052-6.
65. Liu S, Su W, Li Y, Zhang L, Ding X. Manufacturing of an electrochemical biosensing platform based on hybrid DNA hydrogel: taking lung cancer-specific miR-21 as an example. Biosens Bioelectron 2018;103:1-5.
66. Mao X, Chen G, Wang Z, Zhang Y, Zhu X, Li G. Surface-immobilized and self-shaped DNA hydrogels and their application in biosensing. Chem Sci 2018;9:811-8.
67. Zhong R, Tang Q, Wang S, et al. Self-assembly of enzyme-like nanofibrous G-molecular hydrogel for printed flexible electrochemical sensors. Adv Mater 2018;30:e1706887.
68. Shao Y, Sun ZY, Wang Y, Zhang BD, Liu D, Li YM. Designable immune therapeutical vaccine system based on DNA supramolecular hydrogels. ACS Appl Mater Interfaces 2018;10:9310-4.
69. Yao C, Tang H, Wu W, et al. Double rolling circle amplification generates physically cross-linked DNA network for stem cell fishing. J Am Chem Soc 2020;142:3422-9.
70. Yao C, Zhu C, Tang J, Ou J, Zhang R, Yang D. T lymphocyte-captured DNA network for localized immunotherapy. J Am Chem Soc 2021;143:19330-40.
71. Hu Y, Domínguez CM, Bauer J, et al. Carbon-nanotube reinforcement of DNA-silica nanocomposites yields programmable and cell-instructive biocoatings. Nat Commun 2019;10:5522.
72. Hu Y, Niemeyer CM. Designer DNA-silica/carbon nanotube nanocomposites for traceable and targeted drug delivery. J Mater Chem B 2020;8:2250-5.
73. Hu Y, Rehnlund D, Klein E, Gescher J, Niemeyer CM. Cultivation of exoelectrogenic bacteria in conductive DNA nanocomposite hydrogels yields a programmable biohybrid materials system. ACS Appl Mater Interfaces 2020;12:14806-13.
74. Hu Y, Domínguez CM, Christ S, Niemeyer CM. Postsynthetic functionalization of DNA-nanocomposites with proteins yields bioinstructive matrices for cell culture applications. Angew Chem Int Ed Engl 2020;59:19016-20.
75. Hu Y, Grösche M, Sheshachala S, et al. Bottom-up assembly of DNA-silica nanocomposites into micrometer-sized hollow spheres. Angew Chem Int Ed Engl 2019;58:17269-72.
76. Tang J, Ou J, Zhu C, Yao C, Yang D. Flash synthesis of DNA hydrogel via supramacromolecular assembly of DNA chains and upconversion nanoparticles for cell engineering. Adv Funct Materials ; doi: 10.1002/adfm.202107267.
77. Yao C, Tang J, Zhu C, et al. A signal processor made from DNA assembly and upconversion nanoparticle for pharmacokinetic study. Nano Today 2022;42:101352.
78. Creusen G, Akintayo CO, Schumann K, Walther A. Scalable one-pot-liquid-phase oligonucleotide synthesis for model network hydrogels. J Am Chem Soc 2020;142:16610-21.
How to Cite
Download Citation
Export Citation File:
Type of Import
Tips on Downloading Citation
Citation Manager File Format
Type of Import
Direct Import: When the Direct Import option is selected (the default state), a dialogue box will give you the option to Save or Open the downloaded citation data. Choosing Open will either launch your citation manager or give you a choice of applications with which to use the metadata. The Save option saves the file locally for later use.
Indirect Import: When the Indirect Import option is selected, the metadata is displayed and may be copied and pasted as needed.
About This Article
Copyright
Data & Comments
Data
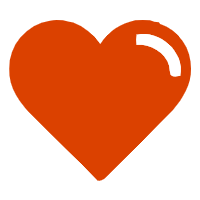
Comments
Comments must be written in English. Spam, offensive content, impersonation, and private information will not be permitted. If any comment is reported and identified as inappropriate content by OAE staff, the comment will be removed without notice. If you have any queries or need any help, please contact us at [email protected].