Atomic modulation and phase engineering of MoS2 for boosting N2 reduction
Abstract
Electrochemical nitrogen reduction reaction (ENRR) has emerged as a potential alternative to the conventional Haber-Bosch process for ammonia production. However, ENRR technology is still restricted by the limited Faradaic efficiency due to the hard-to-break N-N triple bond. Herein, inspired by the biomimetic catalyst, we developed a Fe-modulated MoS2 catalyst (named Fe@MoS2) as an efficient ENRR catalyst. Raman spectra, coupled with the
Keywords
INTRODUCTION
Ammonia (NH3) ranks among the most demanded chemical products in the world, playing an essential role in producing fertilizers, plastics, and medicines[1,2]. Furthermore, it is also an important carbon-free energy carrier, benefiting from its high hydrogen content (17.6 wt%) and high gravimetric energy density
Mo-based nitrogenase enables N2 fixation under ambient conditions[14,15], which inspired researchers to investigate biomimetic catalysts with similar elemental compositions. MoS2, as a layered two-dimensional (2D) semiconductor material, possesses a large surface area and crystal phase[16-19]. Multi-variable interlayer stacking forms and tunable electronic structure make it a promising candidate in the field of electrocatalytic synthesis of NH3[20-22]. Due to the difficulty of activating the non-polar N-N triple bond and the high adsorption barrier of N2, the NH3 yield rate and energy transfer efficiency in ENRR are limited. Moreover, the hydrogen evolution reaction (HER) has a close reaction equilibrium potential to that of ENRR, affecting the selectivity of ENRR in the aqueous phase and leading to unsatisfactory FE[23-25]. Therefore, several strategies have been reported to optimize the MoS2-based catalysts for improving ENRR performance, including vacancy engineering[26,27], heteroatom doping[28,29], phase engineering[30,31], etc.
The catalytic activity and stability of MoS2 with different phases vary greatly in ENRR. Lin et al.[30] compared the ENRR performance of the metastable phase (1T'- and 1T'''-MoS2) and the stable phase
In this work, we developed a synergetic optimization strategy to enhance the ENRR activity of MoS2, coupling vacancy engineering, heteroatom doping, and phase engineering. Biomimetic Fe@MoS2 electrocatalysts were prepared using a one-pot hydrothermal method. The partial MoS2 converts from 2H to 1T phase after Fe atom doping. Furthermore, the unique hydrangea-like morphology and defects on the MoS2 basal plane promote the accessibility of the active site. In-situ and electrochemical characterizations demonstrated that Fe atoms had been inserted into the lattice of MoS2, and its unique coordination structure was analyzed which accelerates the reaction kinetics of N2 to NH3, thus achieving a promising ENRR performance. The maximum FE of 19.7% ± 5.5% and the highest NH3 yield of 20.2 ± 5.3 μg h-1 mg-1 were achieved by Fe@MoS2 at -0.2 V vs. RHE and -0.8 V vs. RHE, respectively.
MATERIALS AND METHODS
Synthesis of materials
One-step hydrothermal synthesis was used to prepare MoS2 [Figure 1A]. Briefly, the mixed solution after sonication (30 mL deionized water (D.I water)) with 0.4 mmol Na2MoO4·6H2O and 0.82 mmol thioacetamide) was transferred into a stainless steel autoclave (50 mL). The heating parameter was set to
Electrochemical measurement
ENRR electrochemical performance was evaluated using a three-electrode system (0.25 M LiClO4) under an ambient environment (25 °C, 1 atm). The working, reference, and counter electrodes are ENRR catalyst,
Determination of NH3
The quantification of NH3 was determined using the salicylic acid-based colorimetric method[34]. To obtain the calibration curve, standard NH4Cl solutions with different concentrations of 0.0, 0.1, 0.2, 0.5, 1.0, and
The NH3 formation rate can be calculated by:
where:
x (ppm): calculated concentration of ammonia.
V (L): volume of reacted electrolyte.
t (h): reaction time in hours.
m (mg): mass of catalyst on the carbon paper.
RNH3 µg h-1 mg-1
The FE is calculated using:
where:
x (ppm): calculated concentration of ammonia.
Mr (NH4+): 18 (g/mol).
V (L): the volume of reacted electrolyte.
I (A): the average current during the reaction.
F: the Faraday constant 96,485 mol-1.
Operando attenuated total reflection surface enhanced infrared absorption spectroscopy
Operando attenuated total reflection surface enhanced infrared absorption spectroscopy (ATR-SEIRAS) utilizes the same three-electrode system as the electrochemical test, and the electrolyte is 0.1 M Li2SO4
RESULTS AND DISCUSSION
Material characterization
The preparation process of Fe@MoS2 and the atomic structure are illustrated in Figure 1A and 1B. X-ray diffraction (XRD) patterns of MoS2 and Fe@MoS2 are shown in Figure 1C. The diffraction peaks at 32.5°, 35.8°, and 57.2° could be indexed to (100), (102), and (110) planes of the standard MoS2 phase (PDF #37-1492)[35-37], respectively. The diffraction peaks of Fe@MoS2 do not change significantly compared to MoS2, and no FeS or FeS2 phases were observed, which indicates that traces of Fe element did not form new sulfide. A scanning electron microscopy (SEM) image [Figure 1D] shows that the synthesized Fe@MoS2 is assembled from multiple 2D nanosheets and exhibits a hydrangea-like morphology. Moreover, Brunauer-Emmett-Teller (BET) test was carried out to characterize the specific surface area of catalysts. Supplementary Figure 2 shows the N2 adsorption-desorption isotherm curve of MoS2 and Fe@MoS2. Fe@MoS2 has a higher BET specific surface area of 54.034 m2 g-1 and a higher pore volume of 0.32 cm3 g-1 than MoS2 (22.63 m2 g-1 and 0.09 cm3 g-1, respectively). The catalytic activity was improved due to more exposed active sites [Supplementary Table 1]. The high specific surface area exposes more active sites, which benefits its catalytic activity. Electrochemically active surface area (ECSA) can be used to evaluate the number of active sites in a catalyst, which is proportional to the double-layer capacitance (Cdl). Supplementary Figure 3 presents the Cdl values obtained after linear fitting. Notably increased Cdl values were observed in Fe@MoS2 (17.85 mF cm-2), compared to MoS2 (0.35 mF cm-2), indicating the beneficial effect of Fe doping on the number of active sites.
No recognizable nanoparticles or clusters are observed in the transmission electron microscopy (TEM) image [Figure 1E]. This suggests that Fe was more likely to be inserted into the MoS2 plane rather than deposited on the surface, which requires further analysis in combination with spectra. Raman spectra
A high-resolution TEM (HRTEM) image [Figure 2A] shows the same results as those obtained from TEM images, with no impurities or other Fe-based structures observed in Fe@MoS2. Further, with the help of dark field scanning TEM (STEM) characterization [Figure 2B and Supplementary Figure 4], it is seen that defects are present on the surface of the 2D structure. Atomic-level Fe (weak Z contrast intensity) replaces some Mo atoms and dopes in the panel of the 2D MoS2 plane. To explore the distribution of different elements in the catalysts, corresponding energy dispersive spectroscopy (EDS) elemental mapping was conducted to characterize Fe@MoS2. Figure 2C demonstrates that Mo, S, and Fe are uniformly distributed in Fe@MoS2.
Figure 2. Material Characterization. (A) HRTEM image of Fe@MoS2. (B) STEM image, and (C) the corresponding EDS elemental mappings of Fe@MoS2, (D)XANES spectra of the Fe K-edge in Fe foil, FeO, Fe2O3, and Fe@MoS2, (E) FT-EXAFS spectra of the Fe K-edge in Fe foil, FeO, and Fe@MoS2. (F) XAFS fitting result of Fe@MoS2 at R space. Inset: Atomic illustration of Fe@MoS2 (Mo atoms: purple; S atoms: yellow; Fe atoms: red).
X-ray absorption near edge structure (XANES) and Fourier transform-extended X-ray absorption fine structure (FT-EXAFS) spectra further revealed the coordination structure and coordination number of Fe atom in Fe@MoS2. As shown in Figure 2D, the absorption edge of Fe@MoS2 is close to the curve of FeO, indicating that Fe of Fe@MoS2 is dominated by a chemical state of +2. In the FT-EXAFS spectra [Figure 2E], a peak can be observed at 1.87 Å for Fe@MoS2, corresponding to the first coordination[43,44]. No Fe-O and Fe-Fe are observed in Fe@MoS2, indicating that the atomically dispersed Fe may dope within Mo-based vacancy and bond with S atoms. Fitting curves [Figure 2F, Supplementary Figure 5,
Electrochemical measurements
The ENRR performance of the Fe@MoS2 is evaluated in an H-type cell equipped with a three-electrode system [Figure 4A]. The linear scan voltammetry (LSV) was first performed to compare the response current density under Ar- or N2-saturated electrolyte of Fe@MoS2. As shown in Figure 4B, higher current density in N2 indicates the possibility of the Fe@MoS2 towards N2 reduction. The subsequent ENRR performance was evaluated using a chronoamperometry method in N2-saturated electrolyte for 3 h, and the response current densities at different potentials were recorded [Figure 4]. The reacted electrolyte was collected and analyzed for the NH3 concentration[34]. The relevant equations have been mentioned above.
Figure 4. Electrochemical performance evaluation. (A) Schematic illustration of the reactor for electrochemical N2 reduction. (B) LSV curves of Fe@MoS2 in Ar and N2-saturated 0.25 M LiClO4 at a scan rate of 10 mV s-1. (C) Chronoamperometric curves of Fe@MoS2 at different applied potentials. NH3 yield rate and FE of (D) MoS2 and (E) Fe@MoS2. The picture of the reacted solution of (F) MoS2 and (G) Fe@MoS2.
The yield rate and FE of NH3 over the electrocatalyst Fe@MoS2 are higher than those of the pristine MoS2 electrocatalyst, which indicates the optimization of the introduction of Fe element in the pristine MoS2 plane. As shown in Figure 4D, the MoS2 can achieve the yield rate of NH3 less than 10 μg h-1 mg-1 between the potential of -0.2 to -0.8 V vs. RHE, while the Fe@MoS2 can achieve the yield rate of NH3 larger than
Operando ATR-SEIRAS was performed to characterize the reaction intermediates and study the reaction mechanism. Figure 5 recorded the ATR-SEIRAS spectra of MoS2 and Fe@MoS2 under different potentials during the ENRR process. As shown in Figure 5A, no obvious peak can be observed for pristine MoS2, indicating no intermediates generated. In contrast, several peaks gradually increase with the increasing potentials for Fe@MoS2 [Figure 5B]. Some peaks attributed to N-H stretching are located at 3,361 and
CONCLUSIONS
In conclusion, an inspired biomimetic electrocatalyst of Fe@MoS2 is designed and successfully prepared using a one-step hydrothermal method. The introduction of atomic-level Fe achieves the geometric and electronic structure modulation of MoS2 and triggers the phase transition of the 2H-phase MoS2 into 1T-phase MoS2. Thanks to the improved atomic structure and increased electrical conductivity of Fe@MoS2, the kinetics are greatly accelerated, thus enhancing ENRR performance. Compared to the pristine 2H MoS2, the prepared Fe@MoS2 boosts the ENRR kinetics and exhibits superior ENRR performance with a yield rate of 20.2 ± 5.3 μg h-1 mg-1 at -0.8 V vs. RHE and a FE of ~19.7% ± 5.5% at -0.2 V vs. RHE. This work provides a new insight for designing efficient ENRR catalysts by synergistic doping and phase engineering.
DECLARATIONS
Authors’ contributions
Synthesis and testing of materials, data collection, and original manuscript writing: Jia Y, Shao G, Li Y, Yang R
Validation and original manuscript revision: Huang M, Huang H, Liu M, Huang G, Lu Q, Gu C
Data collection: Jia Y, Shao G, Li Y, Yang R
Data analysis, writing, review, and editing: Jia Y, Li Y, Shao G
Review and discussion: Jia Y, Li Y, Shao G, Huang H, Huang G, Gu C
Supervision, funding acquisition: Li Y, Huang M
All authors have read and agreed to the published version of the manuscript.
Availability of data and materials
According to reasonable requirements, all of the data examined in this research can be obtained from the correspondents.
Financial support and sponsorship
This work is supported by the National Natural Science Foundation of China (No. 22308322 and No. 52373223), the Science Foundation of Donghai Laboratory (Grant No. DH-2022ZY0010), R&D Project of State Grid Corporation of China (No. 5108-202218280A-2-439-XG), and Sichuan Science and Technology Program (No. 2023NSFSC0434).
Conflicts of interest
Liu M is affiliated with “State Grid Zhejiang Electric Power CO., LTD Research Institute, Hangzhou, China”. While the other authors have declared that they have no conflicts of interest.
Ethical approval and consent to participate
Not applicable.
Consent for publication
Not applicable.
Copyright
© The Author(s) 2024.
Supplementary Materials
REFERENCES
1. Wang J, Yu L, Hu L, Chen G, Xin H, Feng X. Ambient ammonia synthesis via palladium-catalyzed electrohydrogenation of dinitrogen at low overpotential. Nat Commun 2018;9:1795.
2. Suryanto BHR, Matuszek K, Choi J, et al. Nitrogen reduction to ammonia at high efficiency and rates based on a phosphonium proton shuttle. Science 2021;372:1187-91.
4. Chang F, Gao W, Guo J, Chen P. Emerging materials and methods toward ammonia-based energy storage and conversion. Adv Mater 2021;33:e2005721.
5. Cechetto V, Di Felice L, Gallucci F. Advances and perspectives of H2 production from NH3 decomposition in membrane reactors. Energy Fuels 2023;37:10775-98.
6. He W, Zhang J, Dieckhöfer S, et al. Splicing the active phases of copper/cobalt-based catalysts achieves high-rate tandem electroreduction of nitrate to ammonia. Nat Commun 2022;13:1129.
7. Wang L, Xia M, Wang H, et al. Greening ammonia toward the solar ammonia refinery. Joule 2018;2:1055-74.
8. Weng G, Lei S, Wang R, et al. A high-efficiency electrochemical proton-conducting membrane reactor for ammonia production at intermediate temperatures. Joule 2023;7:1333-46.
9. Chen G, Yuan Y, Jiang H, et al. Electrochemical reduction of nitrate to ammonia via direct eight-electron transfer using a copper - molecular solid catalyst. Nat Energy 2020;5:605-13.
10. Sun J, Alam D, Daiyan R, et al. A hybrid plasma electrocatalytic process for sustainable ammonia production. Energy Environ Sci 2021;14:865-72.
11. Arif M, Babar M, Azhar U, et al. Rational design and modulation strategies of Mo-based electrocatalysts and photo/electrocatalysts towards nitrogen reduction to ammonia (NH3). Chem Eng J 2023;451:138320.
12. Mukherjee S, Yang X, Shan W, et al. Atomically dispersed single Ni site catalysts for nitrogen reduction toward electrochemical ammonia synthesis using N2 and H2O. Small Methods 2020;4:1900821.
13. Zheng J, Jiang L, Lyu Y, Jiang SP, Wang S. Green synthesis of nitrogen-to-ammonia fixation: past, present, and future. Energy Environ Mater 2022;5:452-7.
14. Danyal K, Dean DR, Hoffman BM, Seefeldt LC. Electron transfer within nitrogenase: evidence for a deficit-spending mechanism. Biochemistry 2011;50:9255-63.
15. Foster SL, Bakovic SIP, Duda RD, et al. Catalysts for nitrogen reduction to ammonia. Nat Catal 2018;1:490-500.
16. Shi Z, Zhang X, Lin X, et al. Phase-dependent growth of Pt on MoS2 for highly efficient H2 evolution. Nature 2023;621:300-5.
17. Chen S, Liu X, Xiong J, Mi L, Li Y. Engineering strategies for boosting the nitrogen reduction reaction performance of MoS2-based electrocatalysts. Materials Today Nano 2022;18:100202.
18. Li Y, Gu Q, Johannessen B, et al. Synergistic Pt doping and phase conversion engineering in two-dimensional MoS2 for efficient hydrogen evolution. Nano Energy 2021;84:105898.
19. Li Y, Wang H, Xie L, Liang Y, Hong G, Dai H. MoS2 nanoparticles grown on graphene: an advanced catalyst for the hydrogen evolution reaction. J Am Chem Soc 2011;133:7296-9.
20. Zhang L, Ji X, Ren X, et al. Electrochemical ammonia synthesis via nitrogen reduction reaction on a MoS2 catalyst: theoretical and experimental studies. Adv Mater 2018;30:e1800191.
21. Li J, Wei F, Dong C, Wang Z, Xiu Z, Han X. Recent progress of inorganic metal-based catalysts in electrocatalytic synthesis of ammonia. Mater Today Energy 2021;21:100766.
22. Karunadasa HI, Montalvo E, Sun Y, Majda M, Long JR, Chang CJ. A molecular MoS2 edge site mimic for catalytic hydrogen generation. Science 2012;335:698-702.
23. Yan Z, Ji M, Xia J, Zhu H. Recent advanced materials for electrochemical and photoelectrochemical synthesis of ammonia from dinitrogen: one step closer to a sustainable energy future. Adv Energy Mater 2020;10:1902020.
24. Ren Y, Yu C, Tan X, Huang H, Wei Q, Qiu J. Strategies to suppress hydrogen evolution for highly selective electrocatalytic nitrogen reduction: challenges and perspectives. Energy Environ Sci 2021;14:1176-93.
25. Wu W, Wang L, Li Y, et al. Piezoelectricity of single-atomic-layer MoS2 for energy conversion and piezotronics. Nature 2014;514:470-4.
26. Fei H, Guo T, Xin Y, et al. Sulfur vacancy engineering of MoS2 via phosphorus incorporation for improved electrocatalytic N2 reduction to NH3. Appl Catal B Environ 2022;300:120733.
27. Fei H, Liu R, Wang J, et al. Targeted modulation of competitive active sites toward nitrogen fixation via sulfur vacancy engineering over MoS2. Adv Funct Mater 2023;33:2302501.
28. Niu L, Wang D, Xu K, et al. Tuning the performance of nitrogen reduction reaction by balancing the reactivity of N2 and the desorption of NH3. Nano Res 2021;14:4093-9.
29. Guo J, Tadesse Tsega T, Ul Islam I, Iqbal A, Zai J, Qian X. Fe doping promoted electrocatalytic N2 reduction reaction of 2H MoS2. Chin Chem Lett 2020;31:2487-90.
30. Lin G, Ju Q, Guo X, et al. Intrinsic electron localization of metastable MoS2 boosts electrocatalytic nitrogen reduction to ammonia. Adv Mater 2021;33:e2007509.
31. Wu Z, Zhang R, Fei H, Liu R, Wang D, Liu X. Multiphasic 1T@2H MoSe2 as a highly efficient catalyst for the N2 reduction to NH3. Appl Surface Sci 2020;532:147372.
32. You M, Yi S, Hou X, et al. High temperature induced S vacancies in natural molybdenite for robust electrocatalytic nitrogen reduction. J Colloid Interface Sci 2021;599:849-56.
33. Zhao X, Zhang X, Xue Z, Chen W, Zhou Z, Mu T. Fe nanodot-decorated MoS2 nanosheets on carbon cloth: an efficient and flexible electrode for ambient ammonia synthesis. J Mater Chem A 2019;7:27417-22.
34. Ivancic I, Degobbis D. An optimal manual procedure for ammonia analysis in natural waters by the indophenol blue method. Water Res 1984;18:1143-7.
35. Kamila S, Mohanty B, Samantara AK, et al. Highly active 2D layered MoS2-rGO hybrids for energy conversion and storage applications. Sci Rep 2017;7:8378.
36. Sun T, Li Z, Liu X, Ma L, Wang J, Yang S. Facile construction of 3D graphene/MoS2 composites as advanced electrode materials for supercapacitors. J Power Sources 2016;331:180-8.
37. Liu Y, Chen Y, Tian Y, et al. Synergizing hydrogen spillover and deprotonation by the internal polarization field in a MoS2/NiPS3 vertical heterostructure for boosted water electrolysis. Adv Mater 2022;34:e2203615.
38. Wang R, Xu C, Sun J, et al. Heat-induced formation of porous and free-standing MoS2/GS hybrid electrodes for binder-free and ultralong-life lithium ion batteries. Nano Energy 2014;8:183-95.
39. Qian X, Zhu G, Wang K, et al. Bowl-like mesoporous polymer-induced interface growth of molybdenum disulfide for stable lithium storage. Chem Eng J 2020;381:122651.
40. Zhao Y, Chang K, Gu Q, et al. Noble metal-free 2D 1T-MoS2 edge sites boosting selective hydrogenation of maleic anhydride. ACS Catal 2022;12:8986-94.
41. Sheng Z, Qi P, Lu Y, et al. Nitrogen-doped metallic MoS2 derived from a metal-organic framework for aqueous rechargeable zinc-ion batteries. ACS Appl Mater Interfaces 2021;13:34495-506.
42. Hu X, Zeng X, Liu Y, et al. Nano-layer based 1T-rich MoS2/g-C3N4 co-catalyst system for enhanced photocatalytic and photoelectrochemical activity. Appl Catal B Environ 2020;268:118466.
43. Li J, Zhang Y, Liu C, et al. 3.4% Solar-to-ammonia efficiency from nitrate using Fe single atomic catalyst supported on MoS2 nanosheets. Adv Funct Mater 2022;32:2108316.
44. Cao Y, Zhang Y, Yang L, et al. Boosting oxygen reduction reaction kinetics through perturbating electronic structure of single-atom Fe-N3S1 catalyst with sub-nano FeS cluster. J Colloid Interface Sci 2023;650:924-33.
45. Chen K, Wang J, Kang J, Lu X, Zhao X, Chu K. Atomically Fe-doped MoS2-x with Fe-Mo dual sites for efficient electrocatalytic NO reduction to NH3. Appl Catal B Environ 2023;324:122241.
46. Zhao W, Yuan S, Lei S, et al. Tailoring rational crystal orientation and tunable sulfur vacancy on metal-sulfides toward advanced ultrafast ion-storage capability. Adv Funct Mater 2023;33:2211542.
47. Baker M, Gilmore R, Lenardi C, Gissler W. XPS investigation of preferential sputtering of S from MoS2 and determination of MoSx stoichiometry from Mo and S peak positions. Appl Surface Sci 1999;150:255-62.
48. Wang F, Zhang W, Jiang J, et al. Nitrogen-rich carbon-supported ultrafine MoC nanoparticles for the hydrotreatment of oleic acid into diesel-like hydrocarbons. Chem Eng J 2020;382:122464.
49. Xue JY, Li FL, Zhao ZY, et al. In Situ generation of bifunctional Fe-doped MoS2 nanocanopies for efficient electrocatalytic water splitting. Inorg Chem 2019;58:11202-9.
50. Li H, Cheng M, Wang P, et al. Reducing contact resistance and boosting device performance of monolayer MoS2 by in situ Fe doping. Adv Mater 2022;34:e2200885.
51. Liu S, Meng X, Xu J, Zhou H, Liu S, Wang J. Porous Si@C composite anode material prepared using dopamine as a carbon source for high-performance lithium- ion batteries. Int J Electrochem Sci 2020;15:3479-94.
52. Zou W, Yeo SY. Characterization of freeze-thaw treatments upon binders in ancient chinese wall paintings by X-ray diffraction (XRD) and attenuated total reflection - fourier transform infrared (ATR-FTIR) spectroscopy. Anal Lett 2024;57:190-201.
53. Rozenberg M, Jung C, Shoham G. Low temperature FTIR spectra and hydrogen bonds in polycrystalline cytidine. Spectrochim Acta A 2004;60:2369-75.
54. Liu S, Qian T, Wang M, et al. Proton-filtering covalent organic frameworks with superior nitrogen penetration flux promote ambient ammonia synthesis. Nat Catal 2021;4:322-31.
Cite This Article

How to Cite
Download Citation
Export Citation File:
Type of Import
Tips on Downloading Citation
Citation Manager File Format
Type of Import
Direct Import: When the Direct Import option is selected (the default state), a dialogue box will give you the option to Save or Open the downloaded citation data. Choosing Open will either launch your citation manager or give you a choice of applications with which to use the metadata. The Save option saves the file locally for later use.
Indirect Import: When the Indirect Import option is selected, the metadata is displayed and may be copied and pasted as needed.
About This Article
Special Issue
Copyright
Data & Comments
Data
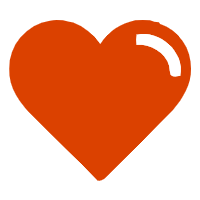
Comments
Comments must be written in English. Spam, offensive content, impersonation, and private information will not be permitted. If any comment is reported and identified as inappropriate content by OAE staff, the comment will be removed without notice. If you have any queries or need any help, please contact us at [email protected].