Si(111) islands on β-phase Si(111)√3 × √3R30°-Bi
Abstract
β-phase √3 × √3R30°-bismuth (Bi) on silicon (Si)(111)7 × 7 surface has been exploited as a template for growing Si films. Two-dimensional Si islands with √3 × √3 reconstruction, parallel to that of Si(111)√3 × √3R30°-Bi, have been resolved by means of scanning tunneling microscopy, grazing-incidence X-ray diffraction (XRD) and low electron energy diffraction. Auger electron spectroscopy and scanning tunneling spectroscopy gave interesting electronic features on two-dimensional Si islands, with the evidence of a reduced band gap to ~0.55 eV, related to the presence of the underneath Bi layer, and atomic structural properties typical of Si(111). These experimental findings fully confirm the recently reported calculation based on the first-principles density functional theory, on the prediction of Si(111) growth on top of β-phase √3 × √3R30°-Bi/Si(111)7 × 7 reconstruction, shedding new light on silicon structures.
Keywords
INTRODUCTION
The study of bismuth (Bi), started in ancient times[1], has stimulated research in surface science physics aimed at understanding the structural and electronic properties of the atomic arrangement of Bi atoms, both on theoretical modelling and experimental fronts, of the Bi interface formation with silicon
Depending on the growth parameters, mainly substrate temperature and Bi coverage, the Bi atoms can arrange on Si(111)7 × 7 in diverse structures, called α-phase, β-phase and honeycomb phase[7-11]. The α-phase is obtained at a temperature of ~613 K, in addition to a Bi coverage of one-third monolayer (ML); β-phase manifests at a temperature of ~523 K and a coverage of one ML of Bi. As a matter of fact, in the interval of temperature 298 K < T < 523 K[7,12-16] and a Bi coverage of two-thirds ML, the honeycomb phase is achieved. These three phases of bismuth on the Si(111)7 × 7 surface exhibit the same reconstruction, presenting the
In the case of α-phase Bi (i.e., a coverage of one-third at high temperature), numerous structural atomic configurations were explored: the so-called T1, T4, H3, and S5 models. The T1 configuration allocates the atoms of Bi above the atoms of Si, which belong to the first layer, leaving active two dangling bonds of silicon; indeed, in the T4 and H3 configurations, there is a threefold coordination with the Si atoms lying on the first layer, being sitting respectively on top of the Si atoms from the second and fourth layers of the substrate. Furthermore, in the S5 model, the Bi atoms substitute the ones of the second Si layer, which are positioned at the T4 sites. The T4 model is considered, from the energy minimization point of view, the most probable for this α-phase Bi[15,16]. Therefore, in this 1/3 ML T4 structure, each Bi atom, sitting above the second layer of the silicon surface atoms, binds with three surface atoms, completely filling the p shell, saturating the surface dangling bonds, and thus passivating the surface[12,15,16].
In the β-phase (1 ML), the structural model is named milkstool due to its peculiar structural shape, where the Bi atoms form trimers, being sideways shifted from the T1 sites, whose centers sit above the T4 site. Thus, the Si atoms from the substrate, rather than carrying one dangling bond, bind to one Bi adatom; Bi then shares electrons with the two nearest-neighbors Bi adatoms, thereby forming the trimer structure[3,10-12].
In the third phase, the 2/3 ML honeycomb structure[11,17], which appears to show a hexagonal structure, the atoms of bismuth are placed on T1 sites, right above the atoms of silicon of the upper layer. One-third of the dangling bonds of the substrate are left unsaturated, making it, more properly, a metastable phase.
Without going into too much detail, we would like to mention the physical importance of the interfaces formed with Bi atoms, in which a two-dimensional (2D) system, such as a surface or interface, can give rise to a spin-polarized 2D electron structure, originating from a structural inversion asymmetry and spin-orbit coupling interaction, formed even on nonmagnetic materials. This effect is called Rashba-Bychkov (RB)[22], and it is the key ingredient in spintronics. This RB effect was observed both on the clean surface of noble metal[23] and on the heavy element of the V group[24], as well as on Bi/Ag(111), where giant spin-splitting appeared through surface alloying of heavy metal atoms adsorbed on a lighter substrate[25]. Even more, a similar behavior could be expected on the interface formation between Bi and much lighter Si atoms.
Particularly intriguing is the case of a one-atom-thick layer of bismuth, organized in a honeycomb grating, called bismuthene, obtained on the insulating silicon carbide substrate, SiC(0001). It has been considered as a structure promoted to exhibit high-temperature quantum spin-Hall effect, as shown by scanning tunneling spectroscopy (STS), which, amazingly enough, depicts a wide gap of ~0.8 eV, with conductive edge states[26].
Equally interesting is the silicene system, a honeycomb layer of one layer of Si atoms, buckled[27] or flat[28], or an ensemble of stacks of silicene layers, called multilayer silicene[29-36].
In particular, recently, silicene and multilayer silicene have been experimentally realized on the α-phase
This work focuses on the formation and investigation of the structural and electronic properties of 2D Si(111) islands on β-phase √3 × √3R30°-Bi on Si(111)7 × 7 surface, through low energy electron diffraction (LEED), reflection high electron energy diffraction (RHEED), Auger electron spectroscopy (AES), grazing incidence X-ray diffraction (GIXRD), scanning tunneling microscopy (STM), and STS. Furthermore, the band gap of the Si islands on β-phase Si(111)√3 × √3R30°-Bi has been explored by STS, which was determined to be ~0.55 eV, exhibiting great potential for various electronic and optoelectronic applications, providing additional insights into the microstructures of silicon.
MATERIALS AND METHODS
The research was executed both at the Institute of Structure of Matter laboratories by Tor-Vergata Campus (Rome, Italy), National Research Council, and at the Institute of Physics by Maria Curie-Sklodowska University (Lublin, Poland). AES spectra were collected using an electron beam energy E = 2.5 keV, setting the electron energy analyzer, a double-pass cylindrical mirror PHY 255G, acquisition mode in the first derivative, at an energy resolution of about 0.5 eV. In order to reconstruct the surface of Si(111), the substrates were annealed by applying several flash cycles at approximately 1,423 K, at the beginning for a few seconds and at the end for 60 s, in an ultra-high vacuum (UHV) apparatus, having a base pressure of about 4 × 10-11 mbar. Bi (99.999%, MATEK Material Technologie Kristalle GmbH) was evaporated on Si(111) (p-doped, 2.5 ohm·cm CZ wafer, MEMC Electronic Material S.p.A.) kept at ~523 K at a rate of
STM imaging in constant current topography and STS measurements were carried at room temperature (RT) in UHV systems, with base pressures better than 5 × 10-11 mbar, equipped with an Omicron LT-STM, RHEED apparatus, and Bi and Si sources. An n-type doped Si(111) sample, with low resistance of
All samples were cleaned in UHV by applying several flashes up to about 1,200 °C from a few seconds up to tens of seconds. Next, ~1.2 ML Bi [counted as ½ Si(111) bilayer atom density] was deposited at RT. The
RESULTS AND DISCUSSION
Figure 1A illustrates the characteristic diffraction pattern related to the 7 × 7 of the Si(111) reconstructed surface. The 1 × 1 unit cell of the unreconstructed Si(111) is characterized by a red rhombus, while the smaller cell, marked by the yellow rhombus, is the one of the Si(111)7 × 7 reconstruction. After the formation of the β-phase of 1 ML of √3 × √3-Bi on Si(111), the LEED pattern reported in Figure 1B shows the complete disappearance of the Si(111)7 × 7 reconstruction. The occurrence of the new √3 × √3 reconstruction related to bismuth, marked by the blue rhombus, rotated by 30° with respect to that of the unreconstructed silicon, is clearly visible. This √3 × √3 reconstruction is maintained for both 1 and 4 MLs of Si deposited in this β-phase of Bi, as evidenced by the LEED patterns of Figure 1C and D. Figure 1E is characteristic of the Si LVV and Bi NOO Auger transitions, located at 91 and 101 eV, respectively, measured from 4 MLs Si grown on top of β-phase of Bi/Si interface, and, for comparison, Si(111)√3 × √3R30°-Bi
Figure 1. LEED Patterns and AES spectrum are reported in (A-E). (A) LEED pattern from: Si(111)7 × 7; (B) LEED pattern from
The Si substrate was kept at a temperature of about ~473 K, and the unit cells of these Si growths, represented in Figure 1C and D also by blue rhombuses, as a guide for the eyes, are overlapped and rotated 30° with respect to that of the Si(111)7 × 7. In these LEED patterns, the background is faintly increased, compared to the interface of bismuth on silicon, as well as the LEED spots, appearing pale and rather broad.
Although unusual, the √3 × √3 reconstruction of bare silicon can take place on the surface of clean Si(111), as already observed in the past[38,39]. In this context, this occurrence is particularly interesting since it constitutes a term of comparison with what was obtained for the synthesized multilayer silicene, instead on the β-phase of Bi interface on Si(111)[37].
Figure 2A shows the XRD measurements acquired on 4 MLs of silicon deposited on the √3 × √3
Figure 2. XRD spectrum and the XRD experimental setup are shown in (A) and (B). (A) XRD from 4 MLs of silicon deposited on the
XRD pattern of Figure 2A was collected slightly out of plane after performing a rocking curve to reduce the contribution of the Si substrate and detect, eventually, a newly appearing peak (α = 1.50°). The tilting of the sample along the α-direction preserves the whole X-ray in-plane scattering angle and, therefore, the position of the XRD reflections, reducing the signal belonging to the monocrystalline silicon substrate, allowing us to probe the contribution from the Si film. Here, we can observe that only a broad XRD peak is present at an angle of 2ϑ = 28.445° (2). This peak comprises both the Si(111) substrate and Si film, where the full width at half maximum is 0.160° ± 0.005°. It is attributed to the (111) reflection of the [111] planes of the silicon substrate, overlapped with the stacked layers of the Si film. By simply applying the law of Bragg (2d = nλsinϑ, where λ is the incident radiation, KαCu = 1.540 Å, and n = 1 is the order of the reflection), it was found, for both Si substrate and film, dSi(111) = 3.140 Å (5), in close agreement with the values achieved in[33-36] for Si substrate alone.
The insert of Figure 2A reports the GIXRD pattern collected from 4 MLs of Si on the β-phase
Figure 3A displays a large-scale STM topographic image, (71.5 × 70.2) nm2, recorded on ~0.27 ML Si deposited at ~220 °C on Si(111)√3 × √3-Bi β-phase interface. The corresponding height profile traced in Figure 3A is reported in Figure 3B, in addition to four colored arrows, set in order to better highlight some main interesting sites on the surface.
Figure 3. The filled-states STM image and height profile are presented in (A) and (B). (A) STM image (71.5 × 70.2) nm2 with a sample bias of -3.0 V and tunneling current I = 100 pA from ~0.27 ML of Si deposited at ~220 °C on Si(111)√3 × √3-Bi β-phase; (B) height profile along the line marked in Figure 1A. In Figure 1B, the positions of blue and red triangles were used to determine the height of the Si island and the step height of the Si(111) substrate with √3 × √3-Bi β-phase, respectively. STM: Scanning tunneling microscopy; ML: monolayer.
At a first visual inspection, it can be observed that the STM image shows several defined silicon islands on top of the substrate. They are iso-distributed on both large terraces, appearing brighter on the left and darker on the right. Most of these Si islands have diameters between 3 and 5 nm. Some of them are especially regular, exhibiting a very flat surface. All of this is shown in the profile of Figure 3B, where a line has been drawn on both the silicon islands of the upper terrace and those of the lower one, intersecting the substrate terrace step. The colored triangles mark the sites where the profile heights were calculated, reporting for the blue one an average island height of 0.32 ± 0.03 nm, a value also found on the height of the island of the lower terrace, whereas the Si(111) step height, marked with two red triangles in Figure 3B, is 0.33 ± 0.03 nm high, in good agreement with the atomic step of Si(111)[41].
To highlight the atomic structure of both Si islands and Bi/Si(111) interface, the high-resolution (HR) empty-states STM images, (14.3 × 14.0) nm2, are shown in Figure 4A and B, where Figure 4A represents the raw data, and Figure 4B those filtered with the fast Fourier transform (FFT), mediated by a broad band pass filter.
Figure 4. The empty-states STM images are reported in (A) and (B). (A) raw data STM image at a sample bias of +1.7 V and tunneling current I = 500 pA (14.3 × 14.0) nm2 from ~0.27 ML of Si deposited at ~220 °C on Si(111)√3 × √3-Bi β-phase; (B) slight FFT filtering of (A) using broad band pass filter. The Si islands and Bi/Si(111) in (B) are labeled, as well as the main crystallographic directions
The Si islands and Bi/Si(111) in Figure 4A are labeled. It should be noted that all Si islands are commensurate with the Si(111) substrate, as shown by the main crystallographic directions
However, the reconstruction pattern in the Si islands is shifted with respect to the Bi/Si(111) direction, and this shift can vary from island to island [Figure 4B], by the two parallel lines along the
Although the surface reconstruction of Bi/Si(111) shown in Figure 4 appears similar to that of the typical Si(111)√3 × √3-Bi α-phase, it is, instead, the Bi β-phase, whose STM images strongly depend on the applied tunneling bias[20].
This behavior is shown in Figure 5A, where the HR filled-states STM image (2.86 × 2.81) nm2 at reversed voltage with respect to that of Figure 4A and B, bias voltage equal to -1.7 V and tunneling current I =
Figure 5. The HR filled-states STM image and height profile are displayed in (A) and (B). (A) STM image (2.86 × 2.81) nm2 collected on Si(111)√3 × √3-Bi β-phase. The image was acquired at -1.7 V bias and tunneling current I = 100 pA; (B) height profile along the line marked in (A). HR: High-resolution; STM: scanning tunneling microscopy.
The I(V) measurements performed on the Si(111)√3 × √3-Bi β-phase and Si island-√3 × √3 surface reconstruction are reported in Figure 6.
Figure 6. I-V characteristics are reported in this figure; the blue curve, A, is collected in the surface reconstruction Si(111)√3 × √3-Bi
Both islands and substrate show semiconducting character with an energy gap of around 0.5 eV. We have validated some scattered data of energy gaps not only for different islands but also within a certain island. Therefore, the value of 0.5 eV bears some uncertainty of about ± 0.2 eV. On the contrary to what is expected for nanostructures, where quantum size effect usually produces a wider band gap[42], in our 2D Si islands, a reduced band gap has been observed. This evidence finds a possible explanation in a Si doping effect due to the underneath Bi layer in the Si/Bi interface formation. On the other hand, two observations have to be taken into consideration: the first is that, nevertheless, the gaps of A (Bi/Si), 0.59 eV, and B (Si/Bi), 0.55 eV, are not so far very different in value, they are different, manifesting without any doubt, the signature of the two regions. Secondly, being the two interfaces one the opposite of the other, and the Bi layer confined between the Si bulk, and the more surface sensitive 2D Si, an imbalance of the electron doping process could favor the B side (Si/Bi), closing the gap and lacking a true crystalline potential here.
On the contrary, the existence of an energy gap larger than 1 eV was recently determined from STS data on the Si(111)√3 × √3-Bi β-phase interface[20].
A possible understandable disagreement between STS data of Ref.[20] and data reported in Figure 6 might be due to a different method of presentation. One has to be aware of very strong dependence of the tunneling current versus tunneling bias, when electron density falls rapidly toward Fermi energy for semiconductors with surface states within the gap. According to density functional theory (DFT) calculations, such states are present in the Ref.[20]. Therefore, a good method to explore states within an energy gap is to determine biases when the tunneling current approaches the unavoidable noise of the tunneling current preamplifier. This determines the limit of the given tunneling microscope. Indeed, the shape of the left side of curve B in Figure 6 clearly deviates from the right side, indicating the presence of additional states at the edge of the valence band. This is in perfect agreement with DFT calculations of Ref.[20] [Figure 2A] showing projected density of states results for Bi surface states in Si(111)√3 × √3-Bi. Moreover, the tunneling curve B for the Si island compared to the A curve for the substrate is shifted by about 0.18 eV towards higher energy, indicating depletion of the surface states on the island in favor of substrate charging.
CONCLUSIONS
Si films were grown in UHV after the √3 × √3R30° interface formation of the β-phase of bismuth on the Si(111)7 × 7 surface reconstruction. LEED, AES, XRD, GIXRD, STM, and STS have been used for the investigation of the Bi/Si(111) interface and Si film growth. Si islands with √3 × √3 reconstruction, commensurate with Bi/Si(111), have been found. All reported results point to the synthesis of (111) stacked Si layers, with a Si-Si along the (111) planes of about dSi(111) = 3.140 Å (5). This constitutes an interesting example of surface science involving 2D materials, where the same reconstruction, √3 × √3, same elements, Bi and Si, same substrate, Si(111), give rise to different Si growth, silicene, in the case of the
DECLARATIONS
Acknowledgments
The authors would like to thank Sandro Priori, Alessandro Ippoliti, and Marco Guaragno for their invaluable technical help.
Authors’ contributions
Conceptualization: De Padova P, Krawiec M
Formal analysis: De Padova P, Ottaviani C, Quaresima C, Generosi A, Paci B, Olivieri B, Jałochowski M, Krawiec M
Funding acquisition: De Padova P, Krawiec M
Investigation: De Padova P, Ottaviani C, Quaresima C, Generosi A, Paci B, Olivieri B, Jałochowski M, Krawiec M
Project administration: De Padova P, Krawiec M
Writing-original draft: De Padova P
Writing-review and editing: De Padova P, Ottaviani C, Quaresima C, Generosi A, Paci B, Olivieri B, Jałochowski M, Krawiec M
All authors have read and agreed to the published version of the manuscript.
Availability of data and materials
Not applicable.
Financial support and sponsorship
This work was supported by the National Science Centre, Poland, under grant no. 2018/29/B/ST5/01572 and Vth Committee of INFN within the NUCLEAAR Project.
Conflicts of interest
All authors declared that there are no conflicts of interest.
Ethical approval and consent to participate
Not applicable.
Consent for publication
Not applicable.
Copyright
© The Author(s) 2024.
REFERENCES
2. Takahashi T, Izumi K, Ishikawa T, Kikuta S. Evidence for a trimer in the √3 × √3 -Bi structure on the Si(111) surface by X-ray diffraction under the nearly normal incidence condition. Surf Sci Lett 1987;183:L302-12.
3. Takahashi T, Nakatani S, Ishikawa T, Kikuta S. Surface structure analysis of Si(111)3×3-Bi by X-ray diffraction - Approach to the solution of the phase problem. Surf Sci Lett 1987;191:L825-34.
4. Park CY, Abukawa T, Higashiyama K, Kono S. Analysis of the atomic structure of the Si(111)√3×√3-Bi surface by X-ray photoelectron diffraction. Jpn J Appl Phys 1987;26:L1335.
5. Shioda R, Kawazu A, Baski AA, Quate CF, Nogami J. Bi on Si(111): two phases of the √3 × √3 surface reconstruction. Phys Rev B 1993;48:4895-8.
6. Park C, Bakhtizin RZ, Tomihiro Hashizume TH, Toshio Sakurai TS. Scanning tunneling microscopy of √3×√3-Bi reconstruction on the Si(111) surface. Jpn J Appl Phys 1993;32:L290.
7. Park C, Bakhtizin RZ, Tomihiro Hashizume TH, Toshio Sakurai TS. Structure of the Bi/Si(111) surface by field-ion scanning tunneling microscopy. Jpn J Appl Phys 1993;32:1416.
8. Bakhtizin RZ, Park C, Hashizume T, Sakurai T. Atomic structure of Bi on the Si(111) surface. J Vac Sci Technol B 1994;12:2052-4.
9. Roesler JM, Sieger MT, Miller T, Chiang TC. New experimental technique of photoelectron holography applied to Bi trimers on Si(111). Surf Sci 1997;380:L485-90.
10. Cheng C, Kunc K. Structure and stability of Bi layers on Si(111) and Ge(111) surfaces. Phys Rev B 1997;56:10283-8.
11. Roesler JM, Miller T, Chiang TC. Photoelectron holography studies of Bi on Si(111). Surf Sci 1998;417:L1143-7.
12. Nakatani S, Takahashi T, Kuwahara Y, Aono M. Use of x-ray reflectivity for determining the Si(111)√3×√3-Bi surface structures. Phys Rev B 1995;52:R8711-4.
13. Miwa RH, Schmidt TM, Srivastava GP. Bi covered Si(111) surface revisited. J Phys Condens Matter 2003;15:2441.
14. Yaginuma S, Nagao T, Sadowski JT, et al. Origin of flat morphology and high crystallinity of ultrathin bismuth films. Surf Sci 2007;601:3593-600.
15. Wan KJ, Guo T, Ford WK, Hermanson JC. Initial growth of Bi films on a Si(111) substrate: two phases of √3 × √3 low-energy-electron-diffraction pattern and their geometric structures. Phys Rev B 1991;44:3471-4.
16. Wan KJ, Guo T, Ford WK, Hermanson JC. Low-energy electron diffraction studies of Si(111)-(
17. Woicik JC, Franklin GE, Liu C, et al. Structural determination of the Si(111) √3×√3-Bi surface by x-ray standing waves and scanning tunneling microscopy. Phys Rev B 1994;50:12246-9.
18. Kuzumaki T, Shirasawa T, Mizuno S, Ueno N, Tochihara H, Sakamoto K. Re-investigation of the Bi-induced Si(111)-(
19. Berntsen MH, Götberg O, Tjernberg O. Reinvestigation of the giant Rashba-split states on Bi-covered Si(111). Phys Rev B 2018;97:125148.
20. Chi L, Nogami J, Singh CV. Bias dependence and defect analysis of Bi on Si(111)
21. Hsieh SC, Hsu CH, Chen HD, Lin DS, Chuang FC, Hsu PJ. Extended α-phase Bi atomic layer on Si(111) fabricated by thermal desorption. Appl Surf Sci 2020;504:144103.
22. Bychkov YA, Rashba ÉI. Properties of 2D electron gas with lifted spectral degeneracy. JETP Lett 1984;39:78-81. Available from: http://jetpletters.ru/ps/1264/article_19121.pdf. [Last accessed on 7 Apr 2024]
23. LaShell S, McDougall BA, Jensen E. Spin splitting of an Au(111) surface state band observed with angle resolved photoelectron spectroscopy. Phys Rev Lett 1996;77:3419-22.
24. Koroteev YM, Bihlmayer G, Gayone JE, et al. Strong spin-orbit splitting on Bi surfaces. Phys Rev Lett 2004;93:046403.
25. Ast CR, Henk J, Ernst A, et al. Giant spin splitting through surface alloying. Phys Rev Lett 2007;98:186807.
26. Reis F, Li G, Dudy L, et al. Bismuthene on a SiC substrate: a candidate for a high-temperature quantum spin Hall material. Science 2017;357:287-90.
27. Vogt P, De Padova P, Quaresima C, et al. Silicene: compelling experimental evidence for graphenelike two-dimensional silicon. Phys Rev Lett 2012;108:155501.
28. Stpniak-dybala A, Dyniec P, Kopciuszyski M, Zdyb R, Jałochowski M, Krawiec M. Planar silicene: a new silicon allotrope epitaxially grown by segregation. Adv Funct Mater 2019;29:1906053.
29. De Padova P, Avila J, Resta A, et al. The quasiparticle band dispersion in epitaxial multilayer silicene. J Phys Condens Matter 2013;25:382202.
30. De Padova P, Vogt P, Resta A, et al. Evidence of Dirac fermions in multilayer silicene. Appl Phys Lett 2013;102:163106.
31. Vogt P, Capiod P, Berthe M, et al. Synthesis and electrical conductivity of multilayer silicene. Appl Phys Lett 2014;104:021602.
32. Grazianetti C, Cinquanta E, Tao L, et al. Silicon nanosheets: crossover between multilayer silicene and diamond-like growth regime. ACS Nano 2017;11:3376-82.
33. De Padova P, Ottaviani C, Quaresima C, et al. 24 h stability of thick multilayer silicene in air. 2D Mater 2014;1:021003.
34. De Padova P, Generosi A, Paci B, et al. Multilayer silicene: clear evidence. 2D Mater 2016;3:031011.
35. De Padova P, Feng H, Zhuang J, et al. Synthesis of multilayer silicene on Si(111)√3 × √3-Ag. J Phys Chem C 2017;121:27182-90.
36. De Padova P, Generosi A, Paci B, et al. New findings on multilayer silicene on Si(111)√3×√3R30°-Ag template. Materials 2019;12:2258.
37. Garagnani D, De Padova P, Ottaviani C, et al. Evidence of sp2-like hybridization of silicon valence orbitals in thin and thick Si grown on α-phase Si(111)√3 × √3R30°-Bi. Materials 2022;15:1730.
38. Berghaus T, Brodde A, Neddermeyer H, Tosch S. STM observation of a new reconstruction on narrow Si(111) terraces. Surf Sci 1987;181:340-5.
39. Fan WC, Ignatiev A, Huang H, Tong SY. Observation and structural determination of (√3×√3)R30° reconstruction of the Si(111) surface. Phys Rev Lett 1989;62:1516-9.
40. Xu YX, Cao XR, Xu LH, Zheng F, Wu SQ, Zhu ZZ. Silicene adsorption on the bismuth-passivated Si(111)
41. Becker RS, Golovchenko JA, McRae EG, Swartzentruber BS. Tunneling images of atomic steps on the Si(111)7 × 7 surface. Phys Rev Lett 1985;55:2028-31.
Cite This Article

How to Cite
Download Citation
Export Citation File:
Type of Import
Tips on Downloading Citation
Citation Manager File Format
Type of Import
Direct Import: When the Direct Import option is selected (the default state), a dialogue box will give you the option to Save or Open the downloaded citation data. Choosing Open will either launch your citation manager or give you a choice of applications with which to use the metadata. The Save option saves the file locally for later use.
Indirect Import: When the Indirect Import option is selected, the metadata is displayed and may be copied and pasted as needed.
About This Article
Special Issue
Copyright
Data & Comments
Data
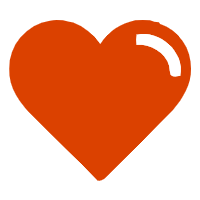
Comments
Comments must be written in English. Spam, offensive content, impersonation, and private information will not be permitted. If any comment is reported and identified as inappropriate content by OAE staff, the comment will be removed without notice. If you have any queries or need any help, please contact us at [email protected].