Assessment of the impact of sampler housing on indoor passive air sampler measurements of SVOCs
Abstract
Passive air sampling (PAS) using a polyurethane foam (PUF) sorbent is a widely used technique to characterize air concentrations of semi-volatile organic compounds (SVOCs) in indoor environments; however, there is little consensus on the type of housing used in sampler housings and how sampler masses are converted to air concentration. We systematically evaluate the three types of PUF-PAS sampler housings most commonly used indoors, and characterize uptake rates for > 50 SVOCs, covering legacy persistent organic pollutants, pesticides, combustion by-products, and flame retardants for all three housing types. There is a clear association between the amount of shielding of the PUF disk and equivalent air volumes for PUF-PAS, with median sampling rates for double-bowl housings of 0.72 m3/day (0.62-0.92 m3/day), 1.3 m3/day (1.0-1.7 m3/day) for single bowl, and
Keywords
INTRODUCTION
PAS for SVOCs is a widely used technique, providing an opportunity to characterize the gaseous and particulate phase air concentrations of a wide range of non-polar and semi-polar organic compounds with limited intrusiveness, low cost, and the ability to capture long-term/time-integrated samples. This technique has been particularly valuable in advancing our ability to characterize indoor air concentrations of SVOCs, given its ease of installation and limited intrusiveness. In recent years, improvements in our understanding of sampling rates have provided better consistency in the characterization of passive air samplers and the ability to convert masses collected by PAS sorbents to concentrations in ambient air[1,2]. The theoretical background underlying passive sampling has been well-elucidated[1,3,4]. In particular, studies have relied on a better understanding of the interdependence of physical-chemical properties of target chemicals[5], atmospheric conditions (e.g., Herkert et al.[6,7]), and sampler geometries[8] to advance our understanding of the key variables in chemical uptake by PAS. However, the focus of our understanding of PAS remains oriented toward the outdoor environment, and indoors, there remains greater heterogeneity in techniques and methods for quantifying air concentrations from PAS.
Despite this, PAS are ideal tools to quantify SVOCs in indoor air: they are unobtrusive, and the more stable indoor environmental conditions mean that variables such as temperature, humidity, wind speed, and oxidant degradation, which can have a substantial impact outdoors[9-12], are much less important indoors. PUF-based PAS have shown their applicability in a wide range of indoor environments, from residences[13,14] and schools[15] to cars[16] and even ships[17].
The greatest source of variability in indoor PAS may be the sampler housings, as this is less consistent than in outdoor environments. Many older studies using PUF-PAS indoors adopted the outdoor air “double-bowl” housing and applied this in indoor environments[18-21]; however, recent work has acknowledged that the greater shielding provided by the double-bowl housing is not necessary indoors due to more limited air movement[22-24], and many studies now rely on a single bowl[25,26] or plate[27], primarily to prevent the gravitational settling of particles onto the PAS sorbent. Beyond this, many more unique configurations have been applied indoors, such as cylindrical mesh tubes encasing the PUF, used as both stationary and personal samplers[17,28,29] and cylindrical chambers incorporating an additional filter[30], or use of PUF without a housing[31].
In this experimental study, we explore the impact of passive sampler housing on the uptake of a wide range of SVOCs to a PUF sorbent, based on simultaneous deployment of PUFs with three different types of sheltering: (1) double-bowl configuration; (2) single-bowl configuration; (3) open deployment without housing, and we provide recommendations for a configuration that balances the need of controlled airflow around the sorbent against sufficient uptake of chemicals of interest.
METHODS
Sampling design
The study was conducted over a period of 56 days in a lecture room at Masaryk University in Brno, Czech Republic (49.1782 N, 16.5711 E) between September and October 2014. The lecture room is located on the 2nd floor of a university building (built in 2012) with a volume of 400 m3, with both mechanical and natural ventilation. Daily average temperatures in the room ranged over 20-23°C. Occupancy of the room was limited to a few hour-long lectures during the study period. PUF-PAS and reference medium volume active air samplers (MV-AAS) were deployed simultaneously [Figure 1 and Supplementary Table 1]. Three sets of PUF-PAS disks (15 cm in diameter x 1.5 cm thick, with a density of 0.030 g cm-3, type T-3037, Molitan a.s., Czech Republic) with different shelter designs were deployed at the start of the study, each containing 24 polyurethane foam passive samplers. The fully sheltered housing consisted of two stainless steel bowls (24 cm diameter lower bowl and 30 cm diameter upper bowl) surrounding a PUF disk, while the partially sheltered design had only the top cover and the non-sheltered design had no bowl [Figure 1]. A set of triplicate PUF-PAS was collected from each set at weekly intervals, generating a total of 24 sets of triplicate PUF-PAS, each corresponding to a specific exposure time and design. An additional set of triplicates for each housing design was deployed from day 28 to day 56. Samplers were hung at 50-100 cm above floor level [Figure 1]. Two MV-AAS (MVS6, Sven Leckel, Ingenieurbüro GmbH, Germany), each equipped with different PM heads (PM1 and PM10), were run continuously as a reference sampler to provide weekly time-integrated concentrations of the targeted chemicals. The sampling train consisted of a Whatman® 47mm ø quartz microfiber filter (QFF, type QMA, GE Healthcare/Cytiva, Chicago, USA) assumed to collect the particle phase and 2 PUFs (55 mm diameter, 50 mm length) assumed as a sorbent for the gas phase. Eight weekly sets of QFF + 2 PUFs were collected for both PM10 and PM1 sampling heads on the same schedule as PAS retrieval to generate uptake curves. Sampler flow rates were 2.3 m3 h-1, resulting in an average of 288 m3 [Supplementary Table 1] per sample. PUF and QFFs were collected and extracted separately to obtain gas and particle-phase concentrations. All sampling media were precleaned by Soxhlet extraction in acetone and dichloromethane, 8 h each. After sampling, exposed media were wrapped in aluminum foil and sealed in plastic bags, and transferred to the laboratory where they were stored at -18 °C until analysis. Detailed methods for PUF/ QFF preparation and deployment have been described elsewhere[24,32].
Analysis
Samples were analyzed at the RECETOX Trace Analytical Laboratories of Masaryk University (accredited CSN EN ISO/IEC 17025). PUFs and QFFs were extracted with DCM using a Büchi B-811 (BÜCHI Labortechnik GmbH, Germany) automated warm Soxhlet extraction system. 13C-labeled or deuterated internal standards (for PBDEs, HBCDDs, PAHs, and OPEs) and non-environmental PCB congeners (for PCBs and OCPs; PCBs 30 and 185) (Wellington Laboratories Inc., Canada) were added before extraction. PUF extracts were split gravimetrically 70:30. The 70% aliquot was purified with an acid-modified silica gel column (50 g activated silica with 22 ml 96% H2SO4) and analyzed for polybrominated diphenyl ethers (PBDEs), hexabromocyclododecane isomers (HBCDDs), polychlorinated biphenyls (PCBs) and organochlorine pesticides (OCPs). The 30% aliquot was cleaned and fractionated using a 5 g activated silica column eluted with 20 mL DCM, followed by 20 mL of 7:3 v/v acetone:DCM. The first fraction was used for the analysis of polycyclic aromatic hydrocarbons (PAHs) and novel halogenated flame retardants (NHFRs), and the second fraction for organophosphate esters (OPEs).
Full compound names and identifiers are given in Supplementary Table 2. Ten PBDEs (congeners 28, 47, 66, 85, 99, 100, 153, 154, 183 and 209) and 23 NHFRs (TBP-AE, TBX, BATE, PBT, PBBZ, PBEB, TBCT, DDC-CO-MA, PBB-Acr, TBP-DBPE, HBB, BTBPE, EH-TBB, TDBP-TAZTO, s-DDC-CO, a-DDC-CO, α-DBE-DBCH, β-DBE-DBCH, α-TBCO, β-TBCO, BEH-TEBP, DBHCTD, DBDPE) were analyzed using gas chromatography coupled to high-resolution mass spectrometry, 15 non-chlorinated OPEs (non-Cl-OPEs: TPrP, TiBP, TnBP, DBPP, TPeP, BDPP, TBOEP, TPHP, EHDPP, TEHP, TOTP, TMTP, TPTP, TIPPP, TDMPP), 3 chlorinated OPEs (Cl-OPEs :TCEP, TCIPP, TDCIPP), 9 PCBs (congeners 9, 11, 28, 52, 101, 118, 153, 138, 180) and 12 OCPs (PeCB, HCB, a-HCH, b-HCH, g-HCH, d-HCH, o,p'-DDE, p,p'-DDE, o,p'-DDD, p,p'-DDD, o,p'-DDT, p,p'-DDT) were analyzed by gas chromatography-tandem mass spectrometry systems, 28 PAHs were analyzed by gas chromatography mass spectrometry and 3 HBCDD isomers were analyzed after exchanging solvent to acetonitrile by liquid chromatography electrospray ionization mass spectrometry. All the analytical procedures used here were published previously[13,14,26,33] and are given in detail in Supplementary Text 1. All analytical standards were purchased from Wellington Laboratories Inc. (Canada). Pesticide residue grade solvents were purchased from Lab-scan (Poland) and Silica Gel 60 (70-230 mesh) from Merck (Germany).
QA/QC
To calculate method detection limits (MDLs) of individual compounds, for each matrix, eight laboratory procedural blanks were processed using precleaned sampling media. An average value plus three times the standard deviation of the blanks was used as MDL, and the average of the procedural blanks was subtracted from the samples above MDL. When a compound was not detected in the blanks, instrumental detection limits were used [Supplementary Table 3].
Compounds with < 50% detection across all samples were excluded from further analysis. For the remaining samples, for statistical data analysis, values below detection were substituted by √2/2*MDL[34]. The Kruskal-Wallis test and multiple comparisons of mean ranks were used to investigate statistical differences. All statistical analyses were performed with STATISTICA (version 13, StatSoft, Inc.) or Microsoft Excel.
Method accuracy was validated through the participation in interlaboratory comparisons for analysis of flame retardants[35], NIST Standard Reference Material 2585[13,14], and through internal QA/QC systems for analysis of POPs in air samples, in accordance with the laboratory accreditation standards.
Literature analysis
To place our calibration study in the wider body of knowledge on the use of PUF-PAS indoors, we performed a comprehensive literature search to determine sampling rates used for indoor PUF-based passive sampling in past studies. This literature search was completed in March 2023, and Web of Science was searched using the keywords [indoor*] AND [air] and [passive*] and a series of chemical-specific terms: [SVOC* OR PCB* OR flame retardant* OR pesticide* OR phthalate* OR OPFR OR PAH. This search identified 248 records, which were manually screened for relevance (i.e., were primary research involving the use of PUF-PAS indoors to capture SVOCs), and to identify any sampling rates/equivalent air volumes used in the study, as well as the source of these rates. Out of the 248 studies, 34 were identified as including information on sampling rates [Supplementary Table 4]. We extracted equivalent sampling volumes or uptake rates from all studies to characterize the range of uptake rates used in practice in the context of sampler housing types.
RESULTS AND DISCUSSION
PM1 vs. PM10 active air samples
Of the 100 target compounds, 64 had detection frequencies > 50% and were thus included in the calculation of sampling rates [Supplementary Table 2].
No significant difference in concentrations measured in QFFs with PM1 and PM10 heads was observed (according to the Kruskal-Wallis test, P > 0.05). Although indoor environments typically have a substantial contribution of 1-10 µm particles (e.g., 75% of total airborne particle mass in French classrooms)[36], these coarse particles typically have less contribution of the chemical burden of particulate matter[37], which may account for the lack of difference between the particle-bound concentrations obtained by the PM1 and PM10 sampling heads. Unfortunately, we are thus not able to use the PM1 vs. PM10 distinction to gain insight into the uptake by samplers according to particle size, which has been suspected to be a contributor to differences observed between different geometries of sampler housings[8,38]. Therefore, the samples from both simultaneously run active samplers were treated as duplicates. i.e., their average of bulk (gas + PM) was used for the ambient air concentration calculation and up to eight equivalent volume points for each time point were used for the uptake curve construction.
Ambient concentrations by active air sampling
The ambient concentrations of the SVOCs determined by AAS were used primarily for calibration of the samplers and are summarized only briefly here. The lowest average bulk (gas + PM) air concentrations were found for PBDEs [Figure 2], which corresponds with the recent construction of the building in 2012, i.e., after European PBDEs bans. Slightly elevated concentrations of NHFRs compared to previous studies[19,24,39,40] were observed, ranging from 0.0452 pg m-3 to 885 pg m-3 (HBB). The highest concentrations of all the compound groups were observed for OPEs, ranging from 0.187 pg m-3 to 127,000 pg m-3 (TCIPP), which agrees with previously published data[14,40]. See Supplementary Table 5 for concentrations and particle-bound fractions determined from the MV- AAS.
Figure 2. Indoor ambient levels of target compound groups, measured by active air sampler during the calibration period. Please note the different units for OPEs and PAHs. Each compound group reflects 8 weekly sampling periods, 2 replicates (n = 16). ∑PCBs is the sum of 6 congeners, ∑OCPs is the sum of 5 compounds, ∑PBDEs is the sum of 4 congeners, ∑HBCDDs is the sum of 3 isomers, ∑NFRs is the sum of 13 compounds, ∑OPEs is the sum of 11 compounds, and ΣPAHs is the sum of 23 compounds.
Concentrations were generally stable over time, in keeping with the limited use of the room during the sampling period. We noted only substantial variations in the levels of NHFRs [Supplementary Figure 1], primarily due to an increase in the levels of BTBPE during sampling week 4, followed by a slow decrease in this compound over subsequent weeks. The source of this BTBPE is unknown, but it does introduce some greater uncertainty into the sampling rates of BTBPE specifically.
Determination of sampling rates
The compound-specific sampling rates were determined as a slope of a linear regression of the equivalent air volume (Veq) dependence on the deployment time. The equivalent volume is calculated as a ratio of a compound mass accumulated in the passive sampler and the corresponding bulk air concentration measured with the active air sampler. Regressions were calculated, taking each triplicate as an individual data point corresponding to the deployment time. The triplicates showed good repeatability for 54 of 65 compounds [Supplementary Table 6], with exceptions being compounds with known analytical challenges, e.g., high molecular weight novel flame retardants such as DBDPE[35]. Additionally, some variations within the room cannot be discounted as contributors to the variability in both the MV-AAS and PAS deployments in the study. While best efforts were made to ensure stable conditions during deployment (selection of time when the room has limited use), in all rooms, we can expect variations in air concentrations and temperature within the room that could contribute differently across the set of samplers in the room.
The following criteria were applied to determine valid sampling rates (SR):
● At least 4 time-points with both AAS and PAS above MDL
● 95th percentile confidence interval of regression line includes origin (reflecting the assumption that concentration is zero at t = 0)
● Regression line fit from the first detected time-point onward (e.g., if t = 7 days and 14 days are < MDL, then regression is fit from t = 21 days onwards)
● Regression line fit is not extended beyond the time-point where all three replicates and all subsequent data points showed a decrease in concentration with increasing deployment. In these cases, the final increasing time-point was identified as the maximum extent of the linear uptake phase.
● Deployment time is identified as a significant (P < 0.05) predictor, although in a few cases, also using less significant relations (0.05 < P < 0.1) was beneficial to show the differences between the shelters and compounds. Furthermore, the scatter around the uptake curves (R2) was also considered to evaluate the relevance of the SR.
Our criteria for valid passive sampling rates rely on the assumption that the passive sampler user wishes to quantify a time-weighted average concentration reflecting the duration of the deployment period, and thus the sampling should be operating in the linear uptake phase, where uptake is air-side controlled and the concentration in the passive sampler is linearly proportional to the sampling time[4]. The results of the regression analysis are given in Supplementary Table 7.
The single and no bowl calibrations yielded valid sampling rates for 38 out of the 62 target compounds for which there was sufficient detection. The double bowl configuration was substantially less successful, with valid rates for only 28 compounds [Figure 3].
Figure 3. Number of valid calibrations for each type of bowl configuration. The green color indicates the percent of each configuration with valid calibration, and the blue shading indicates the number of compounds per compound group that contribute to the total (62).
For the cases where sampling rates were not obtained, key reasons differed. For PCB 153 in the double bowl, there was insufficient detection with the double bowl configuration to calculate a sampling rate, although PCB 153 was consistently detected by the single bowl and no bowl configurations, and by the active sampler, suggesting a reduced equivalent sampling volume with the double-bowl housing. A similar impact was seen for other compounds: many compounds with high octanol-air partitioning coefficients, suggesting higher particle-bound fraction in ambient air, had limited uptake in all bowl configurations and did not have a consistent relationship between deployment time and Veq [Figure 4]. Compounds with logKOA > ~13 generally cannot be considered to have sufficiently consistent uptake over time to be reliably quantified in these sampler housings. Conversely, many gaseous compounds also did not have consistent uptake over time, suggesting rapid initial uptake and exceedance of the linear uptake phase; this effect was especially prominent in the single bowl and no bowl configurations with higher equivalent sampling volumes
Figure 4. Results of calibration represented as sampling rates compared with octanol-air partition coefficients for each individual compound for (A) double bowl; (B) single bowl; (C) no bowl configurations. The points in yellow indicate compounds for which a valid sampling rate could be calculated according to the defined criteria. Compounds for which no valid sampling rate could be calculated are indicated by their logKOA along the lower x-axis, color-coded according to the criteria which prohibited a valid sampling rate from being determined. The dashed green lines show the median sampling rate for each configuration. Three points with outlying sampling rates are omitted from the figures: sampling rates of 13.8 m3/day for BEH-TEBP in the single bowl configuration, 15.7 m3/day for BDE-100 in the single bowl configuration, and 9.38 m3/day for BDE-100 in the no bowl configuration.
The median of the valid sampling rates for the double bowl configuration was 0.72 m3/day
For further analysis and comparison between the bowl configurations, we compared the paired sampling rates for individual compounds across the three housing configurations [Figure 5]. This analysis highlights a few key outcomes of the sampling.
Figure 5. Comparison of sampling rates determined by linear regression for the double bowl, single bowl, and no bowl deployments for (A) PCBs; (B) OCPs; (C) NHFRs; (D) PBDEs and HBCDDs; (E) OPEs; (F) PAHs. Error bars indicate the 95th percentile confidence interval of the linear regression fit of the slopes.
● In addition to the overall difference in the sampling rates identified above, the pattern of higher sampling rates for the more open configurations is clear on an individual compound basis, i.e., double-bowl SR < single-bowl SR < no-bowl SR both for more volatile compounds such as PCBs 9 and 11 [Figure 5A] and largely particle-bound compounds such as BeP and BghiP [Figure 5F]. This is directly related to the greater airflow to the region surrounding the PUF, and thus smaller boundary layer, resulting in a higher equivalent air volume for the different housings. For highly particle-bound substances, this could be further enhanced by gravitational settling of particles on the sorbent when no housing is used; this may account for the higher uptake of selected PAHs to this deployment type.
● For more volatile compounds, valid sampling rates were limited to the two bowl configurations; in this case, the more limited airflow was an advantage as it prevented the sampling from exceeding the linear uptake phase during the deployment period. This was observed for PeCB and HCB, and for PCB-9 only in the two bowl and single bowl configurations.
● Greater uncertainty is introduced for compounds with very low ambient concentrations, e.g., the PBDEs, HBCDDs, and selected NHFRs, resulting in irregular/inconsistent sampling rates. Similarly, despite clear evidence of uptake of particle-bound compounds to PAS, these compounds are associated with low or highly variable sampling rates (e.g., BEH-TEBP, BTBPE, CPD, BeP, BghiP).
● The uncertainty in the sampling rates is lowest for the single-bowl configuration. The relative 95th percent confidence intervals of the regressions were 25% (range 8%-120%) for the single bowl deployment, compared to 33% (range 8%-104%) for the double bowl deployment, and 32% (range 11%-113%) for the no bowl deployment. We hypothesize this is due to the single bowl housing providing a balance of sufficient airflow to the interior of the housing against sufficient sheltering to prevent large variations in air movement without substantially limiting the uptake of particle-bound compounds.
The greater consistency of the single and double bowl configurations is emphasized in the paired comparison of sampling rates per compound across the different housing types. SR for single and double bowl housings are well-correlated across all compounds, while the no bowl configuration is not significantly related to the double-bowl sampling rate [Figure 6]. This suggests that limitations in air flow due to shielding are the main causes of differences in uptake between double- and single-bowl housings, but PUFs deployed without housings are influenced by a combination of additional factors (e.g., irregularities in airflow, particle uptake).
Figure 6. Linear regression of valid SR values from single and no bowl configurations against the double bowl configuration.
Comparison with literature sampling rates
While all three types of housing, as well as variations on these, have been deployed in different studies, no study has comprehensively compared the sampling rates under identical conditions for all three configurations. However, when sampling rates from the literature are analyzed, a similar trend to our experimental study is observed, albeit with greater uncertainty due to the differences in deployment conditions and analytical performance across studies.
Combining the sampling rates given in the literature for a range of SVOCs (including PCBs, OCPs, PBDEs, HBCDDs, OPEs, NHFRs, PAEs, PFAS, and CPs) [Supplementary Table 4], a clear difference is observed between housing types. The median sampling rate in studies deploying a double bowl housing is 1.2 m3/day, compared with 3.8 m3/day, 3.4 m3/day for the upper bowl and upper plate configurations, respectively, and 2.8 m3/day for PUF used without housing [Figure 6]. The sampling rates used for double-bowl configurations are significantly lower than those used for the other deployment types (Mann Whitney
However, the calibrations obtained in our experimental work are well within the range of what is reported in the literature, although the median values from our study (0.72 m3/day (0.62-0.92 m3/day) for double bowl, 1.3 m3/day (1.0-1.7 m3/day) for single bowl, and 1.8 m3/day (1.4-2.2 m3/day) for no bowl are all lower than the median literature values. Such small and systematic variation is likely due to differences in the set of compounds selected for calibrations as well as variations in sampler geometries, such as the size of the upper bowl, PUF dimensions, and overhang, which have been shown to contribute to variations in uptake in outdoor environments[8]. In addition, indoor environment parameters can influence sampler performance, e.g., room ventilation rates and temperature, and in calibration studies, the proximity of the PAS to the AAS inlets and outlets. We note that our study was conducted in a room with lower activity, which may lead to lower uptake compared to calibrations conducted in rooms with more indoor air movement[41].
The range of values obtained by calibrations and applied for the PUF without a housing is substantially larger than for the configurations with housings, replicating what was observed in our experimental study [Figure 7]. The interquartile range is 1.3 for the double-bowl housing, compared to 3.9 for the PUF without a housing. We hypothesize that a combination of free air movement and particle settling can lead to less consistency in this type of deployment than in those with some sheltering. The better consistency of the single-dome housing has been specifically noted by Herkert and Hornbuckle using computational fluid dynamics to model indoor air movement[41].
Figure 7. Synthesis of indoor PAS sampling rates reported in literature (see list of references in Supplementary Table 4). The median is indicated by the horizontal line, the mean is shown by an x, and outliers are indicated by individual points. Double bowl values are significantly lower than upper bowl, upper plate, and no bowl/mesh housings (Mann Whitney U test, P < 0.00001). Results for upper bowl, upper plate, and no bowl/mesh configurations are not significantly different.
Recommendations for application of PUF-PAS indoors
The selection of sampler housing has a clear impact on the uptake of chemicals to the PUF for the full range of SVOCs. No single configuration is ideal for all chemicals and/or deployment conditions, and the needs of the study must be carefully balanced against the advantages and disadvantages of each sampler type. While the use of a PUF disk alone, without housing, appears to allow the highest sampling rate and may be advantageous in situations with short deployment times and/or low ambient concentrations, the lack of a housing was also associated with greater variability in uptake rates (indicated by a wide 95th percent confidence interval in the slopes of the linear regression used to determine sampling rates). A consequence of this is that greater uncertainty would be introduced in the concentrations extrapolated based on the estimated sampling rates. In contrast, a need to sample more volatile compounds in a longer deployment may be better served by a housing that provides greater shielding, e.g., two bowls. For the broadest range of SVOCs under conditions of stable air movement, the single-bowl/plate deployment provides a balance between air flow and shielding, and could be considered the default configuration for indoor studies. However, it must also be acknowledged that the ability of PUF-PAS to provide quantitative concentrations of compounds in indoor air can be considered reliable only for the truly “semi-volatile” non-polar compounds; in our study, this covers those with logKOA from ~7-13, but this range may vary based on ambient conditions such as indoor particle concentrations, temperature, and humidity. Outside this range, many compounds will be detectable, but much greater uncertainty is introduced when detected masses are converted to concentrations. Additionally, the differences between uptake to sampler types due to the amount of shielding may be more substantial in different indoor spaces, such as those with high activity and differing particle size distributions. Room conditions, particularly ventilation rates and variations in movement within a room, can have a significant impact on SR, and calibrations from stable room conditions may not be directly transferable to high activity rooms. This is an inherent limitation in PAS and is best addressed through careful locating of a PAS within a room, e.g., prioritizing locations in well-mixed room air without directed impacts of open windows or ventilation systems.
With the broad proliferation of PUF-PAS use for indoor studies, it has become more frequent that information on the calibration process is excluded, or variations in sampler configuration have
While in this study, we focus on the impact of housing on uptake, it should be noted that other variables, most importantly, the selection of sorbent material, can have a major impact on the range of applicability of PAS indoors. Sorbent-impregnated PUF-PAS (SIP-PAS) have been shown to have improved uptake for a broader range of compounds, attributed to the greater sorptive capacity due to the addition of XAD-4 resin[42,43]. Recent work has identified polydimethylsiloxane (PDMS) foam as a potential sorbent, giving uptake and retention properties similar to PUF, but with lower background and a more appropriate matrix for non-target analysis[44]. These recommendations specific to housing can be extended to the use of other sorbents in PAS bowl housings, and the combined careful consideration of the selection of both sorbent and housing is necessary for reliable use of PAS indoors. Additionally, while variations in sampler housings can impact determinations of air concentrations from PAS, the element of variability introduced by lack of precision and accuracy in laboratory analysis often far exceeds that[8], and when comparing across studies even with different sampler configurations, this may be the greatest cause of discrepancies.
CONCLUSIONS
By calibrating PUF-PAS for a wide range of SVOCs, we were able to identify the ideal range of applicability of PUF-PAS in indoor environments: indoors, PUF-PAS should allow quantification of non-polar SVOCs with logKOA values between 7 and 13. This encompasses a wide range of SVOCs of concern in indoor environments, including many flame retardants, pesticides, combustion by-products, and industrial chemicals, and shows the clear value of PUF-PAS to characterize airborne chemical burdens indoors.
This parallel deployment of indoor passive samplers comparing three deployment/housing configurations showed the impact of housing type on PUF-PAS sampling rates. Sampling rates across the set of targeted SVOCs ranged from 0.02-15 m3/day, with median sampling rates for the double bowl configuration of 0.72 m3/day (0.62-0.92 m3/day), 1.3 m3/day (1.0-1.7 m3/day) for single bowl, and 1.8 m3/day (1.4-2.2 m3/day) for the configuration without a housing. This is matched by the pattern of sampling rates based on a meta-analysis of literature values, which clearly indicate lower uptake for the double-bowl housings, with a median sampling rate in studies deploying a double bowl housing of 1.2 m3/day, compared with 3.8 m3/day for the single bowl configurations, and 2.8 m3/day for PUF used without housings. There is a clear association between the amount of shielding of the PUF disk and equivalent air volumes for PUF-PAS used indoors, and the optimal selection of PUF-PAS housing in indoor studies should consider the physical-chemical properties of the chemicals of interest, as well as the expected ambient levels and deployment durations to enable selection of the best housing configuration. Moreover, it is crucial that the conversion of PUF disk masses to air concentrations is specific to the type of housing used in the PAS deployment.
DECLARATIONS
Authors’ contributions
Conceptualization: Klánová J, Prokeš R, Melymuk L
Methodology: Prokeš R, Vojta Š
Analysis: Vojta Š
Data analysis, manuscript writing: Vojta Š, Melymuk L
Manuscript editing and revision: Vojta Š, Melymuk L, Prokeš R, Klánová J
Supervision and funding: Melymuk L, Klánová J
Availability of data and materials
Data can be obtained from the corresponding author upon reasonable request.
Financial Support and sponsorship
Funded by the European Union, INQUIRE (No. 101057499). The authors acknowledge the RECETOX Research Infrastructure (No LM2023069) financed by the Ministry of Education; Youth and Sports, and Operational Programme Research, Development and Education - project CETOCOEN EXCELLENCE (No CZ.02.1.01/0.0/0.0/17_043/0009632); European Union’s Horizon 2020 research and innovation programme under grant agreement (No 857560) for supportive background. Views and opinions expressed are, however, those of the authors only and do not necessarily reflect those of the European Union or European Health and Digital Executive Agency. Neither the European Union nor the granting authority can be held responsible for them.
Conflict of Interest
Lisa Melymuk is an Editorial Board member of Journal of Environmental Exposure Assessment. Other authors declared that there are no conflicts of interest.
Ethical approval and consent to participate
Not applicable.
Copyright
© The Author(s) 2024.
Supplementary Materials
REFERENCES
1. Wania F, Shunthirasingham C. Passive air sampling for semi-volatile organic chemicals. Environ Sci Process Impacts 2020;22:1925-2002.
2. Li Y, Armitage JM, Wania F. Graphical tools for the planning and interpretation of polyurethane foam based passive air sampling campaigns. Environ Sci Process Impacts 2022;24:414-25.
3. Bartkow ME, Booij K, Kennedy KE, Müller JF, Hawker DW. Passive air sampling theory for semivolatile organic compounds. Chemosphere 2005;60:170-6.
4. Salim F, Górecki T. Theory and modelling approaches to passive sampling. Environ Sci Process Impacts 2019;21:1618-41.
6. Herkert NJ, Martinez A, Hornbuckle KC. A model using local weather data to determine the effective sampling volume for pcb congeners collected on passive air samplers. Environ Sci Technol 2016;50:6690-7.
7. Herkert NJ, Spak SN, Smith A, et al. Calibration and evaluation of PUF-PAS sampling rates across the Global Atmospheric Passive Sampling (GAPS) network. Environ Sci Process Impacts 2018;20:210-9.
8. Melymuk L, Nizzetto PB, Harner T, et al. Global intercomparison of polyurethane foam passive air samplers evaluating sources of variability in SVOC measurements. Environ Sci Policy 2021;125:1-9.
9. Melymuk L, Bohlin P, Sáňka O, Pozo K, Klánová J. Current challenges in air sampling of semivolatile organic contaminants: sampling artifacts and their influence on data comparability. Environ Sci Technol 2014;48:14077-91.
10. Melymuk L, Bohlin-Nizzetto P, Prokeš R, Kukučka P, Klánová J. Sampling artifacts in active air sampling of semivolatile organic contaminants: Comparing theoretical and measured artifacts and evaluating implications for monitoring networks. Environ Pollut 2016;217:97-106.
11. Melymuk L, Bohlin-nizzetto P, Prokeš R, et al. Uncertainties in monitoring of SVOCs in air caused by within-sampler degradation during active and passive air sampling. Atmos Environ 2017;167:553-65.
12. Chaemfa C, Wild E, Davison B, Barber JL, Jones KC. A study of aerosol entrapment and the influence of wind speed, chamber design and foam density on polyurethane foam passive air samplers used for persistent organic pollutants. J Environ Monit 2009;11:1135-9.
13. Venier M, Audy O, Vojta Š, et al. Brominated flame retardants in the indoor environment - Comparative study of indoor contamination from three countries. Environ Int 2016;94:150-60.
14. Vykoukalová M, Venier M, Vojta Š, et al. Organophosphate esters flame retardants in the indoor environment. Environ Int 2017;106:97-104.
15. Marek RF, Thorne PS, Herkert NJ, Awad AM, Hornbuckle KC. Airborne PCBs and OH-PCBs Inside and outside urban and rural U.S. schools. Environ Sci Technol 2017;51:7853-60.
16. Abdallah MA, Harrad S. Modification and calibration of a passive air sampler for monitoring vapor and particulate phase brominated flame retardants in indoor air: application to car interiors. Environ Sci Technol 2010;44:3059-65.
17. Strandberg B, Österman C, Koca Akdeva H, Moldanová J, Langer S. The use of polyurethane foam (PUF) passive air samplers in exposure studies to PAHs in Swedish seafarers. Polycycl Aromat Comp 2022;42:448-59.
18. Persoon C, Hornbuckle KC. Calculation of passive sampling rates from both native PCBs and depuration compounds in indoor and outdoor environments. Chemosphere 2009;74:917-23.
19. Melymuk L, Bohlin-Nizzetto P, Kukučka P, et al. Seasonality and indoor/outdoor relationships of flame retardants and PCBs in residential air. Environ Pollut 2016;218:392-401.
20. Harrad S, Hazrati S, Ibarra C. Concentrations of polychlorinated biphenyls in indoor air and polybrominated diphenyl ethers in indoor air and dust in Birmingham, United Kingdom: implications for human exposure. Environ Sci Technol 2006;40:4633-8.
21. Saini A, Okeme JO, Goosey E, Diamond ML. Calibration of two passive air samplers for monitoring phthalates and brominated flame-retardants in indoor air. Chemosphere 2015;137:166-73.
22. Hazrati S, Harrad S. Calibration of polyurethane foam (PUF) disk passive air samplers for quantitative measurement of polychlorinated biphenyls (PCBs) and polybrominated diphenyl ethers (PBDEs): factors influencing sampling rates. Chemosphere 2007;67:448-55.
23. Harrad S, Abdallah MA. Calibration of two passive air sampler configurations for monitoring concentrations of hexabromocyclododecanes in indoor air. J Environ Monit 2008;10:527-31.
24. Bohlin P, Audy O, Škrdlíková L, et al. Evaluation and guidelines for using polyurethane foam (PUF) passive air samplers in double-dome chambers to assess semi-volatile organic compounds (SVOCs) in non-industrial indoor environments. Environ Sci Process Impacts 2014;16:2617-26.
25. Okeme JO, Yang C, Abdollahi A, et al. Passive air sampling of flame retardants and plasticizers in Canadian homes using PDMS, XAD-coated PDMS and PUF samplers. Environ Pollut 2018;239:109-17.
26. Demirtepe H, Melymuk L, Diamond ML, et al. Linking past uses of legacy SVOCs with today's indoor levels and human exposure. Environ Int 2019;127:653-63.
27. Wilford BH, Harner T, Zhu J, Shoeib M, Jones KC. Passive sampling survey of polybrominated diphenyl ether flame retardants in indoor and outdoor air in Ottawa, Canada: implications for sources and exposure. Environ Sci Technol 2004;38:5312-8.
28. Bohlin P, Jones KC, Strandberg B. Field evaluation of polyurethane foam passive air samplers to assess airborne PAHs in occupational environments. Environ Sci Technol 2010;44:749-54.
29. Strandberg B, Julander A, Sjöström M, Lewné M, Koca Akdeva H, Bigert C. Evaluation of polyurethane foam passive air sampler (PUF) as a tool for occupational PAH measurements. Chemosphere 2018;190:35-42.
30. Tao S, Liu Y, Xu W, et al. Calibration of a passive sampler for both gaseous and particulate phase polycyclic aromatic hydrocarbons. Environ Sci Technol 2007;41:568-73.
31. Meng Z, Wang L, Cao B, Huang Z, Liu F, Zhang J. Indoor airborne phthalates in university campuses and exposure assessment. Build and Enviro 2020;180:107002.
32. Bohlin P, Audy O, Škrdlíková L, et al. Outdoor passive air monitoring of semi volatile organic compounds (SVOCs): a critical evaluation of performance and limitations of polyurethane foam (PUF) disks. Environ Sci Process Impacts 2014;16:433-44.
33. Audy O, Melymuk L, Venier M, et al. PCBs and organochlorine pesticides in indoor environments - a comparison of indoor contamination in Canada and Czech Republic. Chemosphere 2018;206:622-31.
34. Antweiler RC. Evaluation of statistical treatments of left-censored environmental data using coincident uncensored data sets. II. group comparisons. Environ Sci Technol 2015;49:13439-46.
35. Melymuk L, Diamond ML, Riddell N, Wan Y, Vojta Š, Chittim B. Challenges in the analysis of novel flame retardants in indoor dust: results of the INTERFLAB 2 interlaboratory evaluation. Environ Sci Technol 2018;52:9295-303.
36. Tran DT, Alleman LY, Coddeville P, Galloo J. Indoor-utdoor behavior and sources of size-resolved airborne particles in French classrooms. Build and Environ 2014;81:183-91.
37. Xu H, Guinot B, Niu X, et al. Concentrations, particle-size distributions, and indoor/outdoor differences of polycyclic aromatic hydrocarbons (PAHs) in a middle school classroom in Xi'an, China. Environ Geochem Health 2015;37:861-73.
38. Markovic MZ, Prokop S, Staebler RM, Liggio J, Harner T. Evaluation of the particle infiltration efficiency of three passive samplers and the PS-1 active air sampler. Atmos Environ 2015;112:289-93.
39. Melymuk L, Bohlin-Nizzetto P, Vojta Š, et al. Distribution of legacy and emerging semivolatile organic compounds in five indoor matrices in a residential environment. Chemosphere 2016;153:179-86.
40. Cequier E, Ionas AC, Covaci A, Marcé RM, Becher G, Thomsen C. Occurrence of a broad range of legacy and emerging flame retardants in indoor environments in Norway. Environ Sci Technol 2014;48:6827-35.
41. Herkert NJ, Hornbuckle KC. Effects of room airflow on accurate determination of PUF-PAS sampling rates in the indoor environment. Environ Sci Process Impacts 2018;20:757-66.
42. Shoeib M, Harner T, Lee SC, Lane D, Zhu J. Sorbent-impregnated polyurethane foam disk for passive air sampling of volatile fluorinated chemicals. Anal Chem 2008;80:675-82.
43. Ahrens L, Harner T, Shoeib M, Koblizkova M, Reiner EJ. Characterization of two passive air samplers for per- and polyfluoroalkyl substances. Environ Sci Technol 2013;47:14024-33.
Cite This Article

How to Cite
Download Citation
Export Citation File:
Type of Import
Tips on Downloading Citation
Citation Manager File Format
Type of Import
Direct Import: When the Direct Import option is selected (the default state), a dialogue box will give you the option to Save or Open the downloaded citation data. Choosing Open will either launch your citation manager or give you a choice of applications with which to use the metadata. The Save option saves the file locally for later use.
Indirect Import: When the Indirect Import option is selected, the metadata is displayed and may be copied and pasted as needed.
About This Article
Special Issue
Copyright
Data & Comments
Data
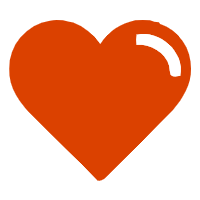
Comments
Comments must be written in English. Spam, offensive content, impersonation, and private information will not be permitted. If any comment is reported and identified as inappropriate content by OAE staff, the comment will be removed without notice. If you have any queries or need any help, please contact us at [email protected].