Extracellular vesicles: emerging therapeutic agents for liver fibrosis
Abstract
Keywords
INTRODUCTION
Liver fibrosis is a complex pathological condition characterized by the excessive accumulation of extracellular matrix (ECM) proteins, including collagen, within the liver[1]. It arises as a wound-healing response to chronic liver injury, where normal tissue architecture is progressively replaced by fibrotic tissue. Liver fibrosis can result from various chronic insults to the liver. The most common causes include chronic viral hepatitis, alcoholic liver disease, metabolic dysfunction-associated steatohepatitis (MASH), cholestatic diseases, autoimmune hepatitis (AIH), and genetic and metabolic disorders. Liver fibrosis disrupts the organ structure and impairs its vital functions, including metabolism, detoxification, bile production, and protein synthesis. Liver fibrosis can lead to significant clinical complications. If left unchecked, fibrosis may progress to cirrhosis, which is characterized by extensive scarring, loss of normal liver architecture, and the development of complications such as portal hypertension, liver failure, and an increased risk of hepatocellular carcinoma (HCC)[2,3].
Liver fibrosis is a dynamic process and, under certain conditions, can be reversed, mainly if the underlying cause of injury is addressed early. This reversibility highlights the importance of timely interventions and the potential therapeutic implications of targeting fibrotic pathways[4]. Recent preclinical studies highlighted the importance of cell-free therapies based on specific cell-derived bio-products, the extracellular vesicles (EVs)[5]. EVs of different sources showed regenerative properties thanks to their biological cargo, which is involved in the reduction of pro-inflammatory molecule secretion and in the targeting of key players of fibrosis, such as activated hepatic stellate cells (HSCs). For these reasons, EVs represent an innovative and promising option to counteract the progression of liver fibrosis by ameliorating organ morphology and function.
EVs
EVs are membrane-bound particles naturally released from cells[6]. EVs consist of aqueous compartments containing diverse biomolecules from the parent cell, including lipids, proteins, nucleic acids, and soluble small molecules[7,8]. EVs are considered key mediators of intercellular communication, influencing a wide range of physiological and pathological processes, and have potential applications in diagnostics and therapeutics. EVs can be classified according to the mode of release. The large membrane vesicles (100-1,000 nm), also called microvesicles (MVs), are secreted by shedding or budding from the plasma membrane. Moreover, exosomes (50-100 nm) originate from internal cell compartments and are released via multivesicular bodies (MVBs), while ectosomes (50-200 nm) are released from the cell surface. Lastly, the apoptotic bodies (50-500 nm) are released from the plasma membrane blebbing of apoptotic cells[5,9,10].
EVs can be found in various biological fluids, including blood, urine, saliva, cerebrospinal fluid, and breast milk. EVs released from cells in different tissues and biofluids provide a rich tapestry of information about the diversity of tissues and cellular sources[11,12]. Key sources include immune cells that release EVs that modulate immune responses, and stem cells that release EVs that contribute to tissue regeneration and repair[13]. EVs produced by adult stem cells, particularly mesenchymal stem cells (MSCs), have critical applications in many sectors, showcasing their versatility. Before, it was thought that MSCs exclusively secreted molecules such as cytokines, chemokines, and growth factors[14]. Nevertheless, research has demonstrated that MSCs can also release EVs reacting to chemical, mechanical, and environmental stimuli[15]. These MSC-derived EVs (MSC-EVs) expressed specific molecules, including CD9, CD90, CD29, CD73, and CD44. MSC-EVs, with their extensive research and significant role as regeneration drivers, have sparked interest in regenerative medicine. The therapeutic effects of MSCs, coupled with the innovative potential of their secretory factors, particularly EVs, could revolutionize therapeutic approaches.
PATHOGENESIS OF LIVER FIBROSIS
The pathogenesis of liver fibrosis involves a complex interplay of cellular and molecular mechanisms [Figure 1]. The initiation phase of liver fibrosis is primarily triggered by hepatocyte injury and the subsequent inflammatory response[16]. Damaged hepatocytes release damage-associated molecular patterns (DAMPs), which are intracellular molecules that act as “danger signals” and initiate a cascade of molecular and cellular events that activate the fibrotic response. Inflammation is a cornerstone of the initiation phase. The inflammatory process begins with the activation of resident immune cells and the recruitment of circulating immune cells. Kupffer cells, the liver resident macrophages, are among the first responders to hepatocyte injury. They recognize DAMPs and pathogen-associated molecular patterns (PAMPs), leading to release of pro-inflammatory cytokines [e.g., tumor necrosis factor-α (TNF-α), interleukin-1β (IL-1β), interleukin-6 (IL-6)], production of chemokines (e.g., CCL2, CCL5), which recruit monocytes, neutrophils, and lymphocytes to the site of injury, and generation of reactive oxygen species (ROS) and nitric oxide, further amplifying oxidative stress[17]. The inflammatory signals attract circulating immune cells, including monocytes, which differentiate into macrophages that perpetuate inflammation and release fibrogenic signals. Neutrophils contribute to tissue damage through degranulation and ROS production; activated T cells release cytokines such as interferon-γ (IFN-γ), driving inflammation and hepatocyte apoptosis. Platelets accumulate at sites of liver injury and release pro-inflammatory mediators, such as serotonin and platelet-derived growth factor (PDGF), which contribute to inflammation[18].
Figure 1. Cells involved in the onset of hepatic fibrosis. Liver fibrosis involves the interplay of several liver-resident and immune cell types. In response to liver injury from various causes, damaged hepatocytes trigger an inflammatory cascade, activating macrophages and promoting the release of ROS and pro-inflammatory cytokines, like TGF-β1. These signals stimulate the activation of quiescent HSCs into myofibroblasts. Activated HSCs proliferate in response to cytokines such as TGF-β and PDGF, producing type I collagen and extracellular matrix components, which drive fibrotic tissue deposition and scar formation. Created using BioRender. Chiabotto, G. (2025) https://BioRender.com/pbjeea3. AIH: Autoimmune hepatitis; ALD: alcohol-associated liver disease; CCLs: CC chemokine ligands; CXCL: CXC chemokine ligand; ECM: extracellular matrix; DAMPs: damage-associated molecular patterns; IFN-γ: interferon-γ; ILs: interleukins; LSEC: liver sinusoid endothelial cell; MAFLD: metabolic-associated fatty liver disease; MASH: metabolic dysfunction-associated steatohepatitis; MMPs: matrix metalloproteinases; NETs: neutrophil extracellular traps; NK: natural killer; NO: nitric oxide; PDGF: platelet-derived growth factor; ROS: reactive oxygen species; TGF-β: transforming growth factor-β; Th: T helper; TNF-α: tumor necrosis factor-α; Treg: T regulatory; VEGF: vascular endothelial growth factor; α-SMA: alpha-smooth muscle actin; TIMPs: tissue inhibitors of metalloproteinases; TLRs: Toll-like receptors; TGF-β1: transforming growth factor-β1; HSCs: hepatic stellate cells.
Central to the process of fibrosis development is the persistent activation of HSCs, the liver’s primary fibrogenic cells[16]. The activation of HSCs is a pivotal event in developing liver fibrosis. HSCs reside in the space of Disse between hepatocytes and sinusoidal endothelial cells and are typically quiescent in a healthy liver. In this state, they serve as storage cells for vitamin A and other retinoids. Upon liver injury, however, HSCs undergo a dramatic transformation into proliferative, migratory, and fibrogenic myofibroblast-like cells, which are the primary producers of ECM components, including collagen[19].
HSC activation results from dynamic crosstalk between various liver cells [Figure 1]. Injured hepatocytes release DAMPs, ROS, and apoptotic bodies, activating signals for HSCs. Kupffer cells secrete pro-inflammatory cytokines (e.g., TNF-α, IL-1β) and transforming growth factor (TGF)-β, directly activating HSCs. Infiltrating immune cells, including monocytes, neutrophils, and T cells, release cytokines and ROS that influence HSC activation[20,21].
ECM remodeling is a critical process in the pathogenesis of liver fibrosis. In healthy livers, ECM components are produced and degraded in a balanced manner. The liver sinusoidal endothelial cells (LSECs), hepatocytes, Kupffer cells, and HSCs all participate in ECM homeostasis through the secretion and degradation of ECM proteins. In liver fibrosis, the normal process of ECM turnover becomes disrupted. The balance between ECM deposition and degradation is skewed toward excessive ECM production and insufficient degradation, leading to fibrosis progression[22,23]. In healthy tissue, matrix metalloproteinases (MMPs), including MMP-2 and MMP-9, degrade collagen and other ECM components, allowing for tissue remodeling and repair. However, in liver fibrosis, the activity of MMPs is inhibited by the overexpression of their inhibitors, leading to a decrease in ECM degradation. The imbalance between MMPs and tissue inhibitors of metalloproteinases (TIMPs) results in accumulating insoluble collagen fibers and other ECM proteins, contributing to fibrosis progression[24].
THERAPEUTIC BENEFITS OF MSC-EVs IN LIVER FIBROSIS
MSC-EVs can mitigate liver fibrosis in different in vitro and in vivo models by influencing crucial mechanisms involved in fibrosis development, such as the activation of HSCs, the regulation of the programmed cell death and the immune cell responses [Figure 2 and Table 1].
Figure 2. Therapeutic effects of MSC-EVs in liver fibrosis. MSC-EVs from various sources exert multiple therapeutic effects in liver fibrosis by delivering bioactive molecules, including proteins, lipids, and nucleic acids, to liver cells. Key mechanisms include the reversion of HSC activation, mainly through the inhibition of fibrosis-related pathways. MSC-EVs also regulate apoptosis by inducing ferroptosis and lipid peroxidation in HSCs while reducing oxidative stress, necroptosis, and pyroptosis in hepatocytes. Additionally, they promote autophagy, suppress angiogenesis, and modulate immune responses, particularly by driving macrophage polarization toward an anti-inflammatory M2 phenotype. Collectively, these effects contribute to fibrosis attenuation and liver tissue regeneration. Created using BioRender. Chiabotto, G. (2025) https://BioRender.com/aitz8fh. Ang-2: Angiopoietin-2; Beclin-1: autophagy-related protein beclin-1; EMT: epithelial-mesenchymal transition; EVs: extracellular vesicles; HSC: hepatic stellate cell; IFN-γ: interferon-gamma; IkBα: inhibitor of nuclear factor kappa b alpha; IL-4: interleukin-4; IL-6: interleukin-6; IL-10: interleukin-10; IL-13: interleukin-13; Keap1: Kelch-like ECH-associated protein 1; LC3: microtubule-associated protein 1 light chain 3; LPS: lipopolysaccharide; MSC-EVs: mesenchymal stromal cell-derived extracellular vesicles; NF-κB: nuclear factor kappa B; Nrf2: nuclear factor erythroid 2-related factor 2; PI3K/AKT/mTOR: phosphoinositide 3-kinase/protein kinase b/mammalian target of rapamycin; TGF-β: transforming growth factor-beta; TNF-α: tumor necrosis factor-alpha.
Effects of MSC-EVs isolated from different tissues in liver fibrosis
MSC-EVs source | Therapeutic effects and mechanisms of action | Liver fibrosis models | References |
Wharton’s Jelly | Inhibition of TGF-β signaling and collagen deposition | TGF-β/cholesterol-treated LX-2 | [25-27] |
Monocyte reprogramming into M2-like anti-inflammatory macrophages and T cell inhibition | Macrophages, LX-2, T cells | [28] | |
Umbilical cord | Inhibition of TGF-β and Hedgehog signaling, reduction of collagen deposition and pathological angiogenesis, reversion of EMT | TGF-β-treated LX-2 and liver spheroids, CCl4, TAA, MASH | [29-38] |
Ferroptosis induction | CCl4 | [39] | |
Macrophage polarization toward M2 phenotype | Macrophages, CCl4, MCD diet | [40,41] | |
Reduction of liver granuloma, inflammation and fibrosis | S. japonicum | [34] | |
Placenta | Reduction of collagen deposition | TGF-β-treated LX-2 and liver organoids | [42] |
Amnion | Inhibition of TGF-β signaling and collagen deposition | LX-2, CCl4 | [43-45] |
Macrophage polarization toward M2 phenotype | LPS-treated Kupffer cells, macrophages, CCl4, MCD diet | [43,44] | |
Embryo | Inhibition of TGF-β signaling | TGF-β-treated LX-2, CCl4 | [46] |
Bone marrow | Inhibition of TGF-β, Hedgehog and Wnt/β-catenin signaling, reduction of collagen deposition | TGF-β-treated LX-2 and liver spheroids, CCl4, DEN | [38,47-52] |
Autophagy restoration | D-GalN/LPS-treated hepatocytes | [53] | |
Inhibition of pyroptosis | CCl4-induced hepatocytes, CCl4 | [54] | |
Suppression of intrahepatic B cell activity | B cells, CCl4, MCD diet | [55] | |
Reduction of liver granuloma, inflammation and fibrosis | S. japonicum | [56] | |
Adipose tissue | Inhibition of TGF-β signaling, reduction of collagen deposition and pathological angiogenesis, reversion of EMT | TGF-β-treated LX-2, CCl4, TAA, DEN | [57-62] |
Autophagy restoration via Nrf2/Keap1/p62 pathway | CCl4 | [59] | |
Macrophage polarization toward M2 phenotype | Macrophages, CCl4, WD/LPS | [63,64] | |
Tonsil | Inhibition of Hedgehog signaling and collagen deposition | TGF-β-treated LX-2, CCl4 | [65] |
Liver | Inhibition of TGF-β signaling and collagen deposition | TGF-β-treated LX-2, CCl4, MCD diet | [66-68] |
Macrophage polarization toward M2 phenotype | Macrophages, CCl4 | [69] |
MSC-EVs regulate HSC activation and modulate pro-fibrotic pathways
Recent findings highlight the potential of MSC-EVs to effectively inhibit TGF-β expression and suppress HSC activation. TGF-β is crucial for wound healing, tissue homeostasis, and embryonic development, with functions extending to cell proliferation, senescence, apoptosis, inflammatory responses, tissue fibrosis, and aging. Among the numerous isoforms of TGF-β, TGF-β1 is the most relevant to hepatic fibrosis, as it promotes the activation of HSCs via the TGF-β1/Smads signaling pathway[70,71].
Fetal tissue-derived MSC-EVs, particularly those from the umbilical cord and Wharton’s jelly, known for their abundance of MSCs, have shown significant promise in the treatment of hepatic fibrosis[72]. EVs isolated from Wharton’s jelly MSCs (WJ-MSC-EVs) can inhibit HSC activation by partially restoring the expression of miR-133a, whose downregulation is associated with fibrosis progression[25]. In vitro, WJ-MSC-EVs substantially reduced the expression of NOX1, NOX2, and NOX4 genes, which are closely associated with TGF-β signaling, as well as the TGF-β/Smad3 pathway, resulting in a decrease of ECM accumulation[25,26]. Moreover, exosomes isolated from WJ-MSCs have been shown to reduce cholesterol-induced liver fibrosis by inhibiting the phosphorylation of the Smad3 protein in LX-2, a HSC human cell line[27].
EVs derived from umbilical cord MSCs (UC-MSC-EVs) demonstrated significant anti-fibrotic potential[29-38]. In a thioacetamide (TAA)-induced liver fibrosis model, UC-MSCs-EVs exerted therapeutic effects through the inhibition of the Hedgehog/Smoothened signaling pathway, which plays a key role in HSC activation[29]. Notably, UC-MSC-EVs reversed the expression of epithelial-to-mesenchymal transition (EMT) markers induced by TGF-β in HSCs, both in vitro and in vivo, as shown in a carbon tetrachloride (CCl4)-induced liver fibrosis model[30]. On a molecular level, these EVs inhibited HSC activation by modulating the miR-148a-5p/SLIT3 axis, providing deeper insights into their anti-fibrotic mechanism of action[31]. By interfering with enzymes like lysyl oxidase (LOX) that mediate collagen crosslinking, UC-MSC-EVs contribute to maintaining ECM flexibility and reduce fibrosis progression[32]. In a MASH model induced by a methionine-choline-deficient (MCD) diet, UC-MSC-EVs attenuated liver fibrosis by inhibiting LSEC angiogenesis via USP9X-mediated modulation of the IκBα/NF-κB/Ang-2 pathway[33]. Comparably to UC-MSC-EVs, EVs from placenta-derived MSCs suppressed collagen I expression via the miR-378c/SKP2 axis, effectively downregulating ECM components in liver fibrosis 2D and 3D in vitro models[42]. Amnion-derived MSC-EVs (AMSC-EVs) downregulated TGF-β1 signaling, reducing HSC activation and collagen production[43,44]. Additionally, they delivered miR-200a to hepatocytes, inhibiting PIK3R3 transcription via suppression of ZEB, thereby mitigating hepatic fibrosis[45]. Likewise, miR-6766-3p-enriched exosomes from human embryonic stem cells (ESCs) have been shown to significantly reduce the expression of pro-fibrotic markers in LX-2 cells. This effect is achieved through the inhibition of TGFβRII and Smad signaling, leading to decreased HSC activation and the prevention of hepatic fibrosis[46].
EVs derived from human bone marrow MSCs (BMSC-EVs) exhibit potent anti-fibrotic properties comparable to those observed with fetal MSC-EVs. In vitro, both BMSC-EVs and UC-MSC-EVs effectively suppressed the expression of pro-fibrotic markers in TGF-β1-activated liver spheroids and HSCs[38]. In a CCl4-induced liver fibrosis model, the administration of BMSC-EVs significantly alleviated liver fibrosis by reducing collagen deposition, improving liver functionality, suppressing inflammation, and promoting hepatocyte regeneration[47,48]. Mechanistically, BMSC-EVs inhibited the expression of key components of the Wnt/β-catenin signaling pathway, such as PPARγ, Wnt3a, Wnt10b, β-catenin, WISP1, and Cyclin D1, alongside fibrogenic markers like alpha-smooth muscle actin (α-SMA) and collagen I, in both activated HSCs and fibrotic liver tissues[47]. Additional anti-fibrotic mechanisms include BMSC-EV-derived miR-618 targeting Smad4, a key mediator in the TGF-β/Smad pathway[49], and circCDK13 regulating MFGE8 through the miR-17-5p/KAT2B axis[50]. Specifically, MFGE8 strongly inhibited TGF-β1-induced HSC activation and mitigated liver fibrosis[51]. In a diethylnitrosamine-induced liver fibrosis model, BMSC-EVs combined with the antihistamine drug rupatadine demonstrated significant anti-fibrotic efficacy by attenuating oxidative stress, inflammation, and necroptosis. This combination therapy upregulated miR-200a levels, leading to reduced expression of key fibrotic markers, including TGF-β1 and α-SMA, while inhibiting the Hedgehog signaling pathway, a critical driver of HSC activation[52].
In a TAA-induced liver fibrosis model, EVs isolated from adipose tissue-derived MSCs (ADSCs-EVs) significantly suppressed the expression of fibrogenic markers, including MMP-2, collagen-1, and α-SMA, while accumulating in fibrotic liver tissue to restore liver functionality[57]. In a CCl4-induced liver fibrosis model, ADSC-EVs alleviated hepatic fibrosis by inhibiting HSC activation, and reducing collagen deposition, EMT, and liver inflammation[58,59]. Mechanistically, ADSC-EVs exploited their anti-fibrotic activity by targeting the PI3K/AKT/mTOR signaling pathway and regulating lipid and choline metabolism, with CHPT1 playing a role in vesicular membrane maintenance[58]. Additionally, ADSC-EVs have been shown to remodel glutamine and ammonia metabolism mediated by hepatocellular glutamine synthetase[60]. Specific microRNAs (miRNAs) in ADSC-EVs, including miR-150-5p, attenuated fibrosis by inhibiting CXCL1 expression[61], while miR-20a-5p targeted the p38 MAPK/NF-κB pathway via TGFBR2 to mitigate fibrogenesis[62].
Tonsil-derived MSCs (T-MSCs) showed comparable characteristics to BMSCs and ADSCs, including similar morphology, surface marker expression, and robust potential for proliferation and differentiation. EVs derived from T-MSCs (T-MSC-EVs) effectively inhibited HSC activation and mitigated fibrosis in a CCl4-induced chronic liver injury model. Notably, T-MSC-EVs are enriched with miR-486-5p, which targets Smoothened to suppress Hedgehog signaling, a key pathway in HSC activation and fibrosis progression[65].
EVs derived from human liver stem cells (HLSC-EVs), an MSC-like population derived from healthy human livers, exhibit potent anti-fibrotic and anti-inflammatory effects. In a murine model of liver fibrosis induced by MCD diet, HLSC-EVs promoted tissue regeneration by delivering bioactive molecules that suppressed fibrogenic pathways and enhanced hepatocyte repair[66]. They also influenced the expression of long non-coding RNAs in the liver, pivotal regulators of hepatic fibrosis and inflammation[67]. Additionally, in vitro studies demonstrated that HLSC-EVs reduce fibrotic markers by modulating TGF-β signaling and inhibiting HSC activation, with their anti-fibrotic effects partially mediated by the delivery of miRNAs, such as miR-146a-5p[68].
MSC-EVs regulate programmed cell death
In addition to the inhibition of HSC activation, MSC-EVs can influence hepatocyte programmed cell death and proliferation - other important aspects to consider in liver fibrosis[73]. MSCs-EVs suppress acetaminophen (APAP)- and H2O2-induced apoptosis of hepatocytes in vitro by positively regulating Bcl-xl expression, and stimulate hepatocyte proliferation in vivo in CCL4-induced liver damage[74]. Another study shows that MSC-EVs reduce apoptosis by increasing autophagy in hepatocytes. Autophagy is a crucial cellular mechanism responsible for maintaining internal homeostasis by degrading and recycling organelles, proteins, and other macromolecules[75]. This process enables cells to adapt to metabolic stress by reusing intracellular components[76]. In the liver, autophagy plays dual roles: it can suppress hepatocyte death and regulate macrophage activity, mitigating fibrosis, while also supporting HSC activation, which promotes fibrosis[77]. Autophagy is essential for sustaining intrahepatic equilibrium in both parenchymal and non-parenchymal cells, emphasizing its significance in energy balance and the removal of damaged cellular components[78]. BMSC-EVs have been shown to enhance autophagy markers such as LC3 and Beclin-1, increasing autophagosome formation in hepatocytes and reducing apoptosis induced by D-galactosamine and lipopolysaccharides (LPS)[53]. ADSC-EVs restored autophagy and Nrf2 expression in a CCl4-induced liver fibrosis model via Nrf2/Keap1/p62 pathway[59]. Thus, leveraging MSC-EVs to regulate autophagy represents a promising therapeutic approach for hepatic fibrosis.
Pyroptosis is another form of programmed cell death associated with inflammation and characterized by gasdermin-mediated cell membrane pore formation, cytokine release, and cellular lysis. It plays a significant role in the progression of liver fibrosis by amplifying inflammatory responses and promoting hepatocyte and HSC damage. In a cirrhosis model, Zhang et al. demonstrated that BMSC-EVs inhibited pyroptosis by downregulating the expression of key pyroptotic markers such as gasdermin D (GSDMD), caspase-1, and IL-1β. By mitigating pyroptosis in hepatocytes and HSCs, BMSC-EVs not only reduced inflammation but also ameliorated fibrosis progression, further supporting their role in promoting hepatic regeneration and maintaining tissue integrity[54].
Ferroptosis is a regulated cell death caused by iron-dependent lipid peroxidation, different from apoptosis, cell necrosis, and autophagy. Recent evidence showed that MSC-EVs can induce ferroptosis in HSCs, thereby alleviating liver fibrosis[39]. Within UC-MSC-EVs, Tan et al. identified BECN1 (Beclin 1), a key regulator of various cellular processes, including autophagy and apoptosis[39]. In a CCl4-induced liver fibrosis model, BECN1-containing exosomes promoted ferroptosis in HSCs by modulating the cystine/glutamate exchange transporter (xCT) and the glutathione peroxidase 4 (GPX4) enzyme. The xCT antiporter system is critical in maintaining redox balance, while GPX4 protects against oxidative stress and lipid peroxidation. By regulating this axis, UC-MSC-EVs facilitate lipid peroxidation and ferroptosis in HSCs, offering a novel therapeutic strategy for liver fibrosis.
MSC-EVs regulate macrophage polarization and influence immune cells
Recent studies highlight the contribution of hepatic macrophages in liver fibrosis, where they initiate, perpetuate, and amplify the inflammatory cascade while critically modulating HSC activation[79]. Macrophages, which exhibit notable plasticity, are categorized into two primary subpopulations: classically activated (M1) macrophages and alternatively activated (M2) macrophages[80] [Figure 1]. M1 macrophages, typically induced by LPS and IFN-γ, are pro-inflammatory cells able to secret cytokines such as IL-1β,
In a CCl4-induced liver fibrosis model, ADSC-EVs localized primarily in liver lesions, with macrophages showing the highest EV uptake[63]. In a melanocortin type-4 receptor knockout mouse model fed with a western diet (WD) and injected with LPS, ADSC-EVs administration increased the frequency of anti-inflammatory macrophages in the liver, thus contributing to improved liver function and attenuated inflammation and fibrosis[64].
MSC-EVs have emerged as pivotal regulators of macrophage polarization, effectively shifting M1 macrophages to the anti-inflammatory M2 phenotype[82]. This polarization plays a critical role in alleviating hepatic fibrosis. For instance, miR-148a, enriched in UC-MSC-EVs, targets the KLF6 pathway, which is involved in the modulation of macrophage behavior through the JAK/STAT signaling pathway[40]. The intravenous administration of UC-MSC-EVs in an MCD-induced liver fibrosis model alleviated hepatic fibrosis by promoting macrophage polarization toward an anti-inflammatory phenotype, leading to increased production of anti-inflammatory cytokines and suppression of pro-inflammatory mediators[41]. Similarly, AMSC-EVs reduced hepatic macrophage infiltration, and favored their polarization to a pro-reparative M2-like phenotype both in vitro and in vivo[43,44]. Furthermore, a specific subset of WJ-MSC-EVs has shown potential in reprogramming monocytes into M2-like functional macrophages with enhanced phagocytic activity, which effectively inhibited the activation of LX-2 cells[28]. By delivering miR-142-5p, liver stem cell-derived EVs regulated macrophage polarization by targeting cathepsin B expression, thereby reducing the pro-inflammatory M1 phenotype and promoting an anti-inflammatory M2 phenotype[69]. This modulation mitigated the inflammatory cascade and fibrosis progression. Based on this evidence, targeting macrophage polarization and leveraging their anti-inflammatory capabilities through MSC-EVs holds great potential for preventing hepatic fibrosis progression.
While the roles of macrophages and Kupffer cells in liver fibrosis are well-established, recent findings by Feng et al. revealed that B cells are also critical mediators of liver fibrosis progression[55]. In both CCl4-induced and MCD diet-induced liver fibrosis models, BMSC-EVs suppressed intrahepatic B cell activity by modulating the MAPK and NF-κB signaling pathways, reducing inflammation and fibrogenesis. These insights provide a novel perspective on the interaction between MSC-EVs and intrahepatic immune cells, expanding their potential applications in the treatment of liver fibrosis.
In addition to toxin-induced liver fibrosis, parasitic infections such as schistosomiasis are major contributors to hepatic fibrogenesis, especially in endemic regions. Schistosoma infection drives liver fibrosis through chronic inflammation and granuloma formation around deposited parasite eggs, perpetuated by macrophage activation and T helper type 2-skewed immune responses. With their proven anti-inflammatory and anti-fibrotic properties, both BMSC-EVs[56] and UC-MSCs[34] have shown effectiveness in treating schistosomiasis-induced liver fibrosis, as demonstrated by the improved liver granuloma and the decreased collagen deposition in the livers of schistosome-infected mice.
THERAPEUTIC EFFECTS OF EVs FROM OTHER SOURCES IN LIVER FIBROSIS
EVs derived from non-MSC animal sources
Differentiated resident liver cell-derived EVs exhibit anti-fibrotic effects comparable to those of MSC-EVs [Table 2]. EVs from LSECs effectively inhibited HSC activation in vitro[83]. Primary human and mouse hepatocyte-derived EVs not only suppressed HSC activation in vitro but also bound to HSCs in vivo, mitigating fibrogenesis, reducing hepatocyte injury, and attenuating inflammation[84,85]. Interestingly, chemical-induced hepatocytes (iHep), hepatocyte-like cells derived from ADSCs[96] and from mouse embryonic fibroblasts[97], offer a scalable and sustainable source of EVs due to their capacity for expansion
Effects of EVs from different sources on liver fibrosis
EV source | Therapeutic effects and mechanisms of action | Liver fibrosis models | References |
LSEC | Increase of inflammatory gene expression in activated HSCs | Primary rat liver cells | [83] |
Decrease of the inflammatory gene expression in Kupffer cells | |||
Primary human and mouse hepatocyte | Suppression of fibrogenic gene expression, reduction of inflammation, and protection of hepatocytes from damage | CCl4 | [84,85] |
iHep | Suppression of the expression of inflammatory genes and cytokines | CCl4 | [86,87] |
Inhibition of HSC activation by targeting the TGF-β1/Smad signaling pathway | |||
Activation of the Nrf2/HO-1 signaling pathway and reduction of oxidative stress, inflammation and fibrosis | |||
iPSC | Reduction of HSC activation and pro-fibrotic markers | Activated human HSC, CCl4, BDL | [88] |
Inhibition of HSC proliferation and migration | |||
Downregulation of fibrosis-related genes | |||
Reduction of collagen deposition and HSC activation in vivo | |||
NK cells | Suppression of TGF-β1-induced HSC proliferation | CCl4 | [89] |
Reduction of fibrosis | |||
Inhibition of HSC activation | |||
Serum | Reduction of hepatocyte death, inflammation, and fibrosis-associated gene expression, increase of hepatocyte proliferation | CCl4, TAA | [90] |
Attenuation of activation of HSCs and reduction of the expression of fibrosis markers | Activated human HSCs | ||
PRP | Reduction of liver fibrosis | CCl4 | [91] |
Decrease of serum ALT level | |||
Promotion of hepatocyte proliferation | |||
Induction of macrophage polarization to an anti-inflammatory phenotype | |||
Milk | Inhibition of HSC proliferation and collagen expression | Activated HSCs | [92] |
Increase PPAR-γ expression | |||
Tea leaf | Inhibition of HSC activation | LX-2, CCl4 | [93] |
Reduction of collagen deposition | |||
Decrease of serum AST & ALT levels | |||
Cannabis bud (Hemp sprout) | Inhibition of HSC activation | LX-2, NAFLD | [94] |
Reduction of collagen deposition | |||
Attenuation of liver fibrosis | |||
Akkermansia muciniphila | Inhibition of HSC activation | LX-2, HFD/CCl4 | [95] |
Reduction of fibrosis markers | |||
Modulation of inflammatory response | |||
Improvement of liver and colon histopathology | |||
Amelioration of intestinal permeability |
Human-induced pluripotent stem cells (iPSCs) naturally produce EVs capable of modulating the pro-fibrogenic phenotype of HSCs. Once internalized by HSCs, iPSC-EVs reduced the expression of fibrogenic markers. Genomic analyses and preclinical efficacy studies revealed that iPSC-EVs are rich in miRNAs, preferentially accumulated in the liver following administration, and effectively alleviated liver fibrosis and HSC activation in murine models of liver injury[88]. Additionally, exosomes derived from immune cells, such as natural killer (NK) cells, exhibited comparable anti-fibrotic properties. Both in vitro and in vivo studies demonstrated that NK cell-derived exosomes suppressed TGF-β1-induced HSC proliferation, reduced fibrosis in CCl4-treated mice, and inhibited HSC activation[89].
EVs from the body fluids of healthy individuals have demonstrated therapeutic potential in liver fibrosis. Serum-derived EVs, for example, attenuated HSC activation, reduced hepatocyte injury, and mitigated inflammation. These effects are largely attributed to specific miRNAs present in serum EVs, such as
EVs derived from non-animal sources
Recent studies have explored the anti-fibrotic potential of EVs derived from non-animal sources, such as plants and bacteria, in treating liver fibrosis [Table 2]. Plant-derived EVs (PDEVs) contain bioactive molecules like miRNAs, proteins, and lipids that reduce inflammation and modulate oxidative stress pathways, central to liver fibrosis progression[98,99]. For instance, EVs derived from tea leaves effectively inhibited HSC activation and attenuated liver fibrosis in a CCl4-induced fibrosis model. Moreover, tea leaves-derived EVs delivered miR-44, a molecule shown to suppress fibrogenic pathways[93]. Furthermore, cannabis bud-derived EVs have proved hepatoprotective in a non-alcoholic fatty liver disease (NAFLD) model by inhibiting oxidative stress and reducing fibrosis-associated markers[94]. Notably, PDEVs exhibit high biocompatibility and low immunogenicity, making them attractive candidates for clinical applications[99]. EVs from probiotics, including Lactobacillus and Bifidobacterium species, further demonstrate therapeutic potential by modulating gut-liver axis communication[100]. For instance, EVs derived from Akkermansia muciniphila, a gut microbiota member, have been shown to regulate inflammation and promote the regression of activated HSCs. In a high-fat diet and CCl4-induced liver injury model, these EVs reduced fibrosis and inflammation biomarkers, ameliorated liver and colon histopathological damage, improved liver function, and normalized inflammatory cytokine levels[95]. These findings suggest bacterial EVs as a promising strategy for addressing liver fibrosis through gut-liver axis modulation.
ENHANCING EV THERAPEUTICS THROUGH CELLULAR PRECONDITIONING AND GENETIC MODIFICATION
Preconditioning of MSCs has emerged as a promising approach to boosting their therapeutic potential in addressing fibrosis and inflammation. Various approaches have been explored to optimize this effect. Hypoxic preconditioning upregulates pro-survival and anti-inflammatory factors[101,102], while exposure to pro-inflammatory cytokines, like TNF-α and IFN-γ, enhances the production of immunomodulatory molecules[103-106]. Pharmacological preconditioning using small molecules[107-109] and genetic modification techniques, such as overexpression of specific proteins or miRNAs, have been employed to augment the therapeutic efficacy of MSCs[110]. These methods collectively aim to modulate the cargo and functional properties of MSC-EVs, ultimately improving their therapeutic efficacy in treating fibrotic and inflammatory conditions[111] [Table 3 and Figure 3].
Figure 3. Enhanced effects of EVs derived from preconditioned cells in liver fibrosis. EVs derived from MSCs and hepatocytes can be preconditioned using various strategies, including exposure to pro-inflammatory cytokines (e.g., IFN-γ and TGFβ1), pharmacological compounds, or genetic engineering with lentiviral and adenoviral vectors, to enhance their therapeutic potential. Compared to native EVs, these modified EVs exhibit improved anti-fibrotic and anti-inflammatory effects. The therapeutic efficacy of these preconditioned EVs has been evaluated in in vivo models of liver fibrosis, including CCl4, TAA, MCD diet, and AIH. Created using BioRender. Created in BioRender. Chiabotto, G. (2025) https://BioRender.com/7120wd6. AIH: Autoimmune hepatitis; CCl4: carbon tetrachloride; EVs: extracellular vesicles; HSC: hepatic stellate cell; MCD: methionine- and choline-deficient; MSCs: mesenchymal stromal cells; TAA: thioacetamide.
Effect of EVs derived from preconditioned and engineered cells in liver fibrosis
Model | EV name | EV sources | Preconditioning method | Effects of EV-administration | Targeted cells | References |
CCl4 | γ-sEVs | ADSC | Preconditioning with IFN-γ | · Induction of anti-inflammatory macrophages · Improvement of inflammation · Reduction of fibrosis |
Macrophages Regulatory T cells |
[112] |
CCl4/TAA | WJ-MSCs exosomes | WJ-MSC | Preconditioning with TGFβ1 | · Decrease of phospho-Smad2/3 levels · Downregulation of α-SMA, and collagen1 · Upregulation of E-cadherin gene expression |
HSCs | [113] |
MCD | MSCs/Exo-Cur | CB-MSC | Preconditioning with curcumin | · Amelioration of fibrosis, steatosis and inflammation · Decrease of the serum level of liver enzymes, triglycerides, and cholesterol · Increase of lipid peroxidation · Downregulation of the ASK-JNK-BAX genes |
HepG2 cells and liver cells in vivo | [114] |
CCl4 | Que-EVs | BMSCs | Preconditioning with quercetin | · Inhibition of M1 macrophage polarization · Reduction of liver fibrosis and inflammation by suppressing the GNAS/PI3K/ERK/STAT3 signaling pathway |
Macrophages | [115] |
CCl4 | Exo- miR146 -5p | Hepatocytes | Preconditioning with salidroside | · Inhibition of HSCs activation · Suppression of liver fibrosis · Reduction of EMT process · Decrease of fibrosis-related genes |
HSCs | [116] |
S100 LPS/ATP |
BMSCs-exomiR-223 | BMSCs | Transfection with lentivirus to overexpress miR-223 or with a miR-223 inhibitor | · Downregulation of inflammatory cytokines · Reduction of liver injury markers · Downregulation of the expression of NLRP3 and caspase-1 |
Hepatocytes (AML12) | [117] |
MCD | HExos | BMSCs | Adenoviral transfection to overexpress HO-1 | · Attenuation of IRI · Reduction of ferroptosis · Improvement of liver function · Reduction of activation of Kupffer and T cells · Reduction of long-term biliary fibrosis · Negative regulation of ferroptosis |
Hepatocytes Bile duct epithelial cells ACSL4 (Enzyme) |
[118] |
TAA | miR-148a-5p-Exo | BMSCs | Lentiviral transfection to overexpress miR-148a-5p | · Downregulation of mRNA and protein levels of TGF-β1, TIMP-1, collagen I, and α-SMA | HSCs | [119] |
Human HSCs (LX-2) | MSC-circDIDO1 Exo | BMSCs | Transfection with lipofectamine with plasmid encoding circDIDO1 | · Suppression of HSC activation through the miR-141-3p/PTEN/AKT pathway | HSCs | [120] |
CCl4 | miR-181-5p-ADSCs Exo | ADSCs | Transfection with lipofectamine to overexpress miR-181-5p | · Downregulation of Stat3 and Bcl-2 · Activation of autophagy · Downregulation of collagen I, vimentin, a-SMA, and fibronectin |
HSCs | [121] |
CCl4 | ADSC-122 Exo | ADSCs | Lentiviral transfection of pre-miR-122 | · Reduction of the gene expression levels of IGF-1R, CCNG1, and P4HA1 · Reduction of collagen deposition |
HSCs | [122] |
CCl4 | ADSCHGF-Exo | ADSCs | Lentiviral transfection to overexpress HGF | · Improvement of liver function · Amelioration of histopathological changes in the liver · Upregulation of the expressions of ALB, CK-18, and HNF4α · Downregulation of the expression of α-SMA, collagen I and Rho GTPase |
Hepatocytes | [123] |
CCl4 TGF-β1-activated mouse HSCs (C2211) |
ADSCHGF-Exo | ADSCs | Lentiviral transfection to overexpress HGF | · Alleviate the activation of HSC · Reduction of the expression of RhoA, Cdc42, and Rac1 · Reduction of the production of collagen |
HSCs | [124] |
TAA | IGF-1-HUCPVC-EVs | ADSC iMSC HUCPVC |
Adenoviral transfection to express IGF-1 | · Reduction of collagen I and α-SMA | HSCs | [125] |
TAA | IGF-1-HUCPVCs EVs | HUCPVC | Adenoviral transfection to express IGF-1 | · Reduction of collagen deposition and expression of pro-fibrogenic genes · Macrophage polarization toward anti-inflammatory phagocytes |
HSCs Hepatic macrophages |
[126] |
CCl4 | BMP7-EVs | UC-MSCs | Lentiviral transfection to express BMP7 | · Modulation of fibrosis cascade response · Reversion of activated HSC phenotype · Suppression of HSC proliferation |
HSCs | [127] |
CCl4 | MSC-EVmiR-4465 | UC-MSCs | Transfection with Lipofectamine to overexpress miR-4465 | · Reduction of LOXL2 expression · Decrease of HSC activation and collagen production |
HSCs | [128] |
Cell preconditioning with pro-inflammatory cytokines
Takeuchi et al. demonstrated that EVs from IFN-γ-preconditioned human ADSCs exhibited enhanced therapeutic effects[112]. Administering these (γ-EVs) in a murine CCl4-induced liver fibrosis model resulted in improved liver function and a marked reduction in fibrosis, with decreased collagen deposition and downregulation of fibrosis-related genes. Additionally, γ-EVs promoted a shift in the liver macrophage population toward an anti-inflammatory phenotype and the expansion of regulatory T cells (Tregs), thus contributing to immune tolerance and fibrosis suppression. At the molecular level, γ-EVs were found enriched with anti-fibrotic miRNAs and proteins. Furthermore, the γ-EVs enhanced efferocytosis, the process by which macrophages clear apoptotic cells, aiding in the resolution of inflammation and preventing chronic fibrosis.
Similarly, Salehipour Bavarsad et al. investigated exosomes derived from WJ-MSCs preconditioned with TGFβ1[113]. The preconditioning of WJ-MSCs with TGFβ1 altered the exosomal cargo by enriching it with proteins and miRNAs associated with the regulation of fibrogenic pathways in the liver, thus enhancing their therapeutic efficacy. In CCl4- and TAA-induced liver fibrosis models, the administration of TGFβ1-preconditioned exosomes significantly reduced liver fibrosis. This anti-fibrotic effect was particularly evident in HSCs, where exosomes downregulated the TGFβ/Smad signaling pathway and promoted the breakdown of ECM components, thereby mitigating fibrosis.
Cell preconditioning using pharmacological compounds
Tawfeek et al. explored exosomes derived from human cord blood mesenchymal stromal cells (CB-MSCs) preconditioned with curcumin (MSCs/Exo-Cur) for treating MCD-induced liver fibrosis[114]. Administration of MSCs/Exo-Cur significantly reduced hepatic lipid accumulation, improved liver function, and exhibited prolonged anti-fibrotic effects. Unlike unconditioned MSC-derived exosomes, which provided only temporary benefits, MSCs/Exo-Cur offered sustained protection against fibrosis both in vitro and in vivo, suggesting that curcumin preconditioning significantly enhances their anti-inflammatory, anti-oxidant, and anti-fibrotic properties. Besides curcumin, another well-known compound with broad pharmacological activities is quercetin (Que). Jiang et al. demonstrated that priming BMSCs with this flavonoid enhances the anti-fibrotic effects of their EVs[115]. Notably, Que preconditioning increased levels of the anti-inflammatory miR-136-5p in BMSC-EVs. In a CCl4-induced liver fibrosis model, Que-BMSC-EVs inhibited M1-type macrophage polarization and alleviated liver inflammation by suppressing the GNAS/PI3K/ERK/STAT3 signaling pathway. Another study by Lang et al. examined the efficacy of exosomes derived from hepatocytes preconditioned with Salidroside, a natural anti-inflammatory and anti-oxidant compound, in liver fibrosis[116]. Preconditioning of hepatocytes with Salidroside enhanced the bioactive properties of their exosomes, making them more effective in mediating the crosstalk between hepatocytes and HSCs. Upon administration in a CCl4-induced liver fibrosis model, exosomes derived from Salidroside-preconditioned hepatocytes effectively inhibited the activation of HSCs and suppressed the EMT, a crucial step in fibrosis progression, and downregulated the expression of fibrosis-related genes in HSCs. By delivering anti-fibrotic miRNAs such as miR-146a-5p, these exosomes could modulate HSC behavior, thus reducing fibrogenic activity and mitigating the fibrotic response in the liver.
Cell preconditioning through genetic engineering
Another promising preconditioning strategy involves the use of genetically engineered MSCs as an EV source. Chen et al. generated a murine model featuring major pathologic characteristics of AIH through the injection of hepatic S100[117]. Then, they tested the in vivo efficacy of exosomes derived from BMSC transfected with a lentiviral vector to overexpress miR-223. These miR-223-enriched exosomes ameliorated liver function and reduced inflammation, as evidenced by decreased levels of pro-inflammatory cytokines and essential inflammatory mediators, including NLRP3 and caspase-1, involved in the inflammasome pathway. This dual action of reducing inflammation and modulating immune responses highlights the role of miR-223-enriched exosomes in mitigating AIH progression. The downregulation of NLRP3 and caspase-1 induced by miR-223-enriched exosomes was also validated in vitro using the murine hepatocyte cell line AML12.
Fatty liver disease presents significant challenges in transplantation, mainly due to the increased susceptibility to ischemia-reperfusion injury (IRI). This heightened susceptibility complicates the post-transplant recovery process, contributing to poorer outcomes. To address this issue, Tian et al. explored the therapeutic potential of exosomes isolated from BMSCs genetically modified through adenoviral transfection to overexpress heme oxygenase 1 (HO-1), an enzyme known for its cytoprotective and anti-inflammatory properties[118]. In a rat model of hepatic steatosis induced by a MCD diet, which closely mimics the clinical scenario of fatty liver transplantation, HO-1-overexpressing exosomes prevented the development of biliary fibrosis, improved liver function, and attenuated IRI by reducing oxidative stress and ferroptosis, as evidenced by decreased levels of markers of oxidative damage, such as malondialdehyde (MDA) and iron. Furthermore, EV treatment also led to decreased activation of Kupffer cells and reduced infiltration of T cells, suggesting a dampened inflammatory response.
In another study, Xuan et al. used lentiviral transfection to generate miR-148a-5p-overexpressing BMSCs, demonstrating significant anti-fibrotic effects in a TAA-induced liver fibrosis model[119]. Exosomes from engineered BMSCs improved overall liver function and attenuated fibrosis by delivering miR-148a-5p directly into HSCs and downregulating Smad4, a key signaling molecule in the TGF-β1 pathway. This led to a decrease in pro-fibrotic markers expression, thereby reducing ECM deposition and ameliorating fibrosis.
Beyond miRNAs, circular RNAs (circRNAs) also play a crucial role in EV-mediated anti-fibrotic effects.
Qu et al. investigated the therapeutic potential of exosomes derived from miR-181-5p-modified ADSCs in liver fibrosis[121]. In a CCl4-induced liver fibrosis model, these exosomes attenuated liver injury and significantly downregulated fibrotic markers. In vitro, miR-181-5p-enriched exosomes suppressed HSC activation and significantly enhanced autophagy by inhibiting the STAT3/Bcl-2/Beclin 1-dependent pathway. Similarly, Lou et al. demonstrated that lentiviral transfection of ADSCs with miR-122 improved the therapeutic potential of their exosomes[122]. In a CCl4-induced liver fibrosis model, miR-122-enriched exosomes suppressed HSC activation and reduced collagen deposition. In vitro, these exosomes enhanced the G0/G1 arrest of HSCs by regulating key miR-122 target genes, such as insulin-like growth factor receptor 1 (IGF-1R), Cyclin G1 (CCNG1), and prolyl-4-hydroxylase α1 (P4HA1), which are involved in HSC proliferation and collagen maturation. Furthermore, exosomes derived from ADSCs transfected with a lentiviral vector to overexpress hepatocyte growth factor (HGF) exhibited enhanced anti-fibrotic properties[123,124]. Compared to exosomes from unmodified ADSCs, those from HGF-overexpressing ADSCs (ADSCHGF-Exo) demonstrated superior efficacy in reducing the accumulation of collagen and restoring liver function. Besides the reduction of hepatic fibrosis-related proteins, ADSCHGF-Exo treatment also downregulated Rho GTPase proteins, specifically CDC42 and Rac1, both involved in the progression of liver injury[123]. In vitro, ADSCHGF-Exo effectively attenuated cell viability, induced cell cycle arrest at the S phase, and promoted apoptosis of TGF-β1-activated HSCs[124]. Compared to those from unmodified ADSCs, ADSCHGF-Exo showed a stronger suppressive effect on the expression of crucial fibrogenic markers in TGF-β1-treated HSCs.
The engineering of human umbilical cord perivascular cells (HUCPVCs) with adenoviruses coding for insulin-like growth factor 1 (IGF-1) produced IGF-1-enriched EVs with enhanced anti-fibrotic effects. In a TAA model, treatment with IGF-1-enriched EVs reduced fibrosis and promoted liver regeneration.
EVs AS DRUG-DELIVERY SYSTEMS IN LIVER FIBROSIS
EVs represent a new generation of targeted drug delivery carriers that can contribute to the improvement of therapeutic strategies for human diseases, such as liver fibrosis[129]. Considering their biocompatibility, capacity to cross the blood-brain barrier, intrinsic targeting ability, and involvement in cell-to-cell communications, EVs show different advantages over artificial systems[130]. The application of EVs loaded with drugs, RNA interference molecules, miRNAs, and proteins represents a promising frontier in treating acute and chronic liver diseases[131] [Figure 4 and Table 4]. This innovative potential of modified EVs in drug delivery and regenerative medicine opens a world of possibilities, inspiring further research and development.
Figure 4. EV modifications to enhance drug delivery in liver fibrosis. Different engineering strategies used to optimize EVs for drug delivery in liver fibrosis. EVs can be modified to improve their targeting ability and enhance cargo loading. Various molecular cargoes, including small molecules, RNAs, and proteins, can be loaded into EVs to counteract liver fibrosis. The combination of these modifications enables the development of more efficient and precise EV-based drug delivery systems for liver fibrosis treatment. Created using BioRender. Created in BioRender. Chiabotto, G. (2025) https://BioRender.com/nblg6av. ASO: Antisense oligonucleotide; Cas9: CRISPR-associated protein 9; CRISPR: clustered regularly interspaced short palindromic repeats; DSPE-PEG: 1,2-distearoyl-sn-glycero-3-phosphoethanolamine-polyethylene glycol; EV: extracellular vesicle; mRNA: messenger RNA; siRNA: small interfering RNA; STAT3: signal transducer and activator of transcription 3; VP64: viral protein 64.
EVs as drug delivery systems in liver fibrosis
Model | Loading protocol | EV sources/Isolate | Loaded components | Effects of EV-administration | Targeted cells | References |
MSC-EVs | ||||||
Murine CCl4 | Sonication | Human WJ-MSCs |
OCA | · Activation of the FXR-Cyp7a1 cascade to reduce liver fibrosis · Prevention of HSC activation · ECM remodelling |
HSCs | [132] |
Murine CCl4 | CaCl2 | Human WJ-MSCs | miR-124-3p mimic | · Downregulation of STAT3 signaling · Downregulation of pro-inflammatory and pro-fibrotic markers |
HSCs Ly6Chi monocytes |
[133] |
Murine CCl4 | Electroporation | Human BMSCs |
siRNA or ASO targeting STAT3 | · Downregulation of STAT3 · Amelioration of liver function and morphology · Inhibition of HSC activation |
HSCs | [134] |
Rat CCl4 |
Sonication and physical incubation | Rat BMSCs |
LUT | · Amelioration of liver function · Reduction of collagen deposition · Reduction of immune cell infiltration · Downregulation of TNF-α, MMP-2 and TGF-β |
HepG2 | [135] |
Murine CCl4 |
Electroporation | Human ADSCs |
siRNA targeting OPN | · Inhibition of HSC proliferation · Reduction of collagen deposition and α-SMA expression · Inhibition of TGF-β1 signaling |
HSCs | [136] |
Murine TAA | Phospholipid insertion method | Human ADSCs |
DSPE-PEG-Vitamin A on the surface | · Inhibition of HSC activation · Downregulation of α-SMA, p-SMAD2, TIMP-1 and LYC6 protein expression · Upregulation of CK18 protein expression |
HSCs | [137] |
EVs from other cell sources | ||||||
Rat TAA |
Electroporation | Rat primary hepatocytes | miR-4500 | · Targeting of TGF-βR1 · Inhibition of HSC activation · Attenuation of liver fibrosis, oxidative stress and angiogenesis |
HSCs | [138] |
Murine CCl4 |
CRISPR/dCas9 technology | Murine hepatocytes (AML12) |
CRISPR/dCas9-VP64 | · Activation of HNF4α · Downregulation of α-SMA and collagen I protein expression |
HSCs | [139] |
Murine APAP-ALI, CCl4, orthotopic model | Electroporation | Human HSCs (LX-2) | Cas9 RNP complexes | · Downregulation of PUMA, Cyclin E1 and KAT5 · Inhibition of HSC activation · Amelioration of liver fibrosis |
Hepatocytes and HSCs | [140] |
Rat CCl4 |
Electroporation | Rat HSCs (HSC-T6) | lefty1 mRNA | · Inhibition of HSC activation · Downregulation of α-SMA, collagen I, and TIMP-1 and MMP-1 · Inhibition of TGF-β/SMAD signaling |
HSCs | [141] |
Rat CCl4 |
Co-incubation and ultrasound cycles | Rat HSCs (HSC-T6) | PFD; DSPE-PEG2000-HA on the surface | · Inhibition of HSC activation · Amelioration of hepatic cell morphology · Amelioration of liver fibrosis |
HSCs | [142] |
Zebrafish TAA |
EDC/NHS chemistry; co-incubation in ultrasonic conditions | Milk | FA; DSPE-PEG2000-HA on the surface | · Inhibition of TGF-β1-induced HSC proliferation · Downregulation of α-SMA and collagen protein · Amelioration of liver function and morphology |
HSCs | [143] |
Zebrafish TAA |
EDC/NHS chemistry; co-incubation | Milk | PHI; DSPE-PEG2000-HA on the surface | · Inhibition of HSC activation · Downregulation of autophagy · Amelioration of liver function |
HSCs | [144] |
Murine CCl4 |
Co-incubation and saponin-mediated loading | Milk | Curcumin | · Reduction of serum markers of liver function · Reduction of ECM components |
Hepatocytes | [145] |
Murine ALF and orthotopic model | Electroporation | RBC | miR-155-ASO, doxorubicin, sorafenib | · Amelioration of liver function and histology · Inhibition of tumor growth and angiogenesis · Induction of polarization of M2 macrophages |
M1 macrophages, tumoral cells | [146] |
Modified MSC-EV applications in liver fibrosis
MSC-EVs can be modified to load small-molecule payloads, enhancing their anti-fibrotic properties[147]. Their small size, straightforward structure, and low immunogenicity further underscore their strong abilities as regulatory factors and transporters of signaling molecules[148]. As reported in Table 4, different studies delve into groundbreaking therapeutic approaches for liver fibrosis using EVs derived from MSCs as delivery systems for different therapeutic molecules. EVs derived from h-WJ-MSCs loaded with obeticholic acid (OCA), a farnesoid X receptor (FXR) agonist with hepatoprotective properties, were administered in a CCl4-induced liver fibrosis murine model[132]. Exo-loaded OCA accumulated in the liver and effectively improved liver function and mitigated tissue fibrosis compared to free OCA. Through the activation of FXR-Cyp7a1 cascade, Exo-loaded OCA was able to prevent HSC activation and modulate the expression of genes and proteins involved in ECM remodeling, facilitating hepatic recovery.
Considering that the aberrant expression of miR-124-3p is associated with the progression of liver diseases, Niknam et al. produced miR-124-3p-enriched exosomes (ExomiR-124), which were administered to CCl4-mice for three weeks[133]. ExomiR-124 improved hepatic fibrosis by reducing the production of pro-inflammatory cytokines and pro-fibrotic markers, particularly collagen. Furthermore, ExomiR-124 induced the splenic monocytes to acquire a restorative Ly6Clo phenotype and downregulated Signal transducer and activator of transcription 3 (STAT3) levels, which contributed to the inhibition of α-SMA gene expression, resulting in HSC deactivation. As mentioned above, STAT3 is a key regulator of liver fibrosis; in fact, it is involved in the activation of fibroblasts and HSCs, which acquire a myofibroblast-like phenotype[149,150]. In the study conducted by Tang et al., EVs were purified from human-BM-MSCs, and they were electroporated with small interfering RNAs (siRNA) (iExosiRNA-STAT3) or antisense oligonucleotides specifically targeting STAT3 (iExomASO-STAT3)[134]. In vitro experiments demonstrated that iExosiRNA-STAT3 and iExomASO-STAT3 effectively targeted activated HSCs and reduced the mRNA levels of STAT3. In vivo, an amelioration of liver function and morphology was observed in the CCl-4 murine model, mainly due to the reduction in ECM deposition. Transcriptomic analyses on liver tissue revealed that modified EVs improved liver function by downregulating genes involved in fibrosis-associated pathways, including ECM accumulation, HSC activation, and inflammation. These results suggested that the EV-mediated delivery of STAT3-targeting therapeutics could represent a highly innovative and promising strategy for treating chronic fibrotic liver diseases.
In another research study, EVs were isolated from rat-BMSCs and loaded with luteolin (LUT), a flavonoid found in many edible plants, which possesses anti-inflammatory and anti-oxidant properties, and is known to alleviate liver fibrosis by inhibiting the proliferation of activated HSCs[151,152]. In a rat CCl4-induced model of liver fibrosis, Ashour et al. demonstrated that luteolin-loaded exosomes (LUT-Ex) reduced the expression of pro-inflammatory marker TNF-α and pro-fibrotic markers hydroxyproline, MMP-2, and TGF-β[135]. Compared to LUT-suspension and to blank EVs, LUT-Ex induced a stronger improvement of hepatic function, and a greater anti-fibrotic activity, as shown by the decreased immune cell infiltration and collagen deposition in fibrotic livers.
Other research studies investigate EVs isolated from ADSCs as a delivery platform for siRNA-based therapy targeting key regulatory genes in liver fibrosis. For example, high plasma levels of osteopontin (OPN) correlate with advanced fibrosis progression[153], and a similar trend was also observed in patients with NAFLD[154] and chronic HBV-induced fibrosis[136]. Tang et al. demonstrated that exosome-mediated delivery of a siRNA targeting OPN (iExosiRNA-OPN) inhibited ECM deposition in a CCl4-induced liver fibrosis model[136]. In vitro results demonstrated how iExosiRNA-OPN were taken up by HSCs and reduced their proliferative capacity. Interestingly, by speculating the mechanism of action of iExosiRNA-OPN, Tang et al. highlighted the inhibition of TGF-β1 expression due to the blocking of HMGB1 translocation to the cytoplasm, resulting in HSC inactivation and reduced liver fibrosis. Interestingly, EVs isolated from ADSCs and coupled with vitamin A (V-EVs) showed potent anti-fibrotic effects in liver fibrosis[136]. You et al. demonstrated that V-EVs were selectively taken up by activated HSCs, thus reducing the deposition of ECM and inhibiting the activation and proliferation of HSCs in a TAA-induced liver fibrosis model[137]. Furthermore, the administration of V-EVs resulted in a decrease in the number of LY6Chi macrophages, responsible for the phagocytosis of damaged hepatocytes, and consequently in the production of pro-inflammatory cytokines, thus contributing to hepatocyte regeneration.
Modified EVs from other sources in liver fibrosis
In addition to MSC-EVs, EVs from other cell sources have also been modified to enhance drug delivery for liver fibrosis treatment. Yang et al. developed a ROS-responsive injectable hydrogel encapsulating hepatocyte-derived exosomes loaded with miR-4500 (QCG@ExosmiR-4500)[138]. This hydrogel, formed through dynamic crosslinking of quaternized chitosan and carboxyphenylboronic acid-modified gelatin, specifically targets activated HSCs, delivering miR-4500 to inhibit their activation and promote apoptosis. In a TAA-induced liver fibrosis model, this treatment effectively reduced collagen deposition and improved liver function.
Luo et al. isolated EVs from murine hepatocytes (AML12), and they were loaded with CRISPR/dCas9-VP64 (clustered regularly interspaced short palindromic repeats/associated protein) system to test a new therapeutic strategy for liver fibrosis[139]. Interestingly, this system was efficiently transferred into HSCs both in vitro and in vivo, inducing an amelioration of liver fibrosis. The researchers observed that the EV-mediated delivery of the CRISPR/dCas9-VP64 system to activated HSCs in vivo induced the reduction of the mRNA and protein levels of α-SMA and collagen I, providing a promising gene therapy strategy for treating hepatic fibrosis through HSC reprogramming.
The EV-mediated delivery system for CRISPR-Cas9 gene editing was also employed on EVs isolated from LX-2. Wan et al. successfully loaded CRISPR-Cas9 ribonucleoprotein (RNP) into purified HSC-derived EVs (exosomeRNP)[140]. In vitro, exosomeRNP efficiently delivered RNP in the cytosol of HSCs and, in vivo, they accumulated in the hepatic tissue. This EV-mediated delivery of Cas9 RNP showed significant therapeutic potential in a mouse model of a single overdose of APAP-induced acute liver injury via targeting of p53-upregulated modulators of apoptosis (PUMA). In addition, exosomeRNP targeted cyclin E1 (CcnE1) and K (lysine) acetyltransferase 5 (KAT5) in a murine model of CCl4-induced chronic liver injury and an orthotopic murine model of HCC, respectively. Cyclin E1 is a protein kinase that regulates the proliferation of HSCs[155] and its genome editing mediated by exosomeRNP resulted in a significant reduction of HSC activation, with consequent attenuation of liver fibrosis[140].
Other studies further explore the therapeutical application of EVs isolated from HSCs. For instance,
Milk-derived EVs were also explored as drug delivery systems to treat liver diseases. Gong et al. described a targeted delivery method based on milk-derived EVs to encapsulate and administer Forsythiaside A (FA), an active herbal ingredient of traditional Chinese medicine Forsythiae Fructus with hepatoprotective properties[143]. In this study, the researchers developed CD44-specific ligand HA-modified milk-derived exosomes (mExo) loaded with FA (HA-mExo-FA). In vitro, HA-mExo-FA inhibited the proliferation of LX-2 and reduced the gene and protein levels of both α-SMA and collagen. In vivo, the researchers developed a zebrafish TAA-induced liver fibrosis model and observed the ability of HA-mExo-FA to improve hepatic function and morphology compared to free FA. Interestingly, the same research group conjugated milk-derived EVs with Hyaluronic acid (DSPE-PEG2000-HA) on their surface and loaded them with Phillygenin (PHI), a hepatoprotective agent with low water solubility[144]. PHI-HA-mEXO could specifically bind to the surface of activated HSCs and enter through CD44-mediated endocytosis. PHI-HA-mEXO exhibited a beneficial anti-fibrotic effect by partially inhibiting HSC activation through the downregulation of autophagy. Another research study unveils a promising method for delivering natural compounds. To improve curcumin bioavailability and therapeutic efficacy for the treatment of chronic liver disease, Albaladejo-García et al. encapsulated curcumin within small milk EVs (sEVCur)[145]. In a CCl4-induced liver fibrosis model, sEVs loaded with curcumin via active cargo loading with saponin (sEVCurAc) significantly decreased both the serum markers of liver damage and the deposition of ECM components compared to free curcumin.
In the last years, different studies have highlighted the ability of red blood cell-derived EVs (RBC-EVs) as drug delivery carriers due to their ability to accumulate in the liver, as well as their biocompatibility and safety in transporting bioactive molecules, including RNAs[156]. Zhang et al. observed that RBC-EVs accumulate in the liver through macrophage-dependent mechanisms[146]. Considering this aspect, they investigated whether RBC-EVs could serve as natural drug delivery systems for treating liver diseases. To test this hypothesis, RBC-EVs were electroporated with FAM-labeled miR-155-ASOs and their protective effects were evaluated in a murine model of acute liver failure. By regulating gene expression, RBC-EVs/miR-155-ASOs promoted macrophage polarization toward the anti-inflammatory M2 phenotype, ameliorating hepatic function and histology. Moreover, the researchers loaded RBC-EVs with doxorubicin (RBC-EVs/Dox) and sorafenib (RBC-EVs/SRF) to assess their therapeutic efficacy compared to free drugs in an orthotopic liver cancer mouse model. RBC-EVs/Dox inhibited tumoral growth in vivo and suppressed HCC-LM3 cell proliferation, while RBC-EVs/SRF reduced angiogenesis both in vitro and in vivo.
EV-LIPOSOME HYBRID DELIVERY SYSTEMS IN LIVER FIBROSIS
The development of EV-liposome hybrid delivery systems has unveiled promising therapeutic opportunities for liver fibrosis, combining the unique attributes of EVs and liposomes. These hybrid systems integrate the targeting precision and biocompatibility of EVs with the high drug-loading capacity and stability of liposomes, creating an advanced platform for the precise delivery of anti-fibrotic agents[157]. Moreover, their surface can be functionalized with ligands or antibodies to enhance specificity toward fibrotic liver tissue, ensuring precise drug delivery while minimizing systemic toxicity[158]. Additionally, EVs naturally exhibit a longer half-life in vivo compared to synthetic nanoparticles, preserving the therapeutic efficacy of encapsulated drugs. Their fusion with liposomes further strengthens structural integrity and improves drug retention, ensuring targeted delivery to liver-resident cells[159].
One prominent example is the HCQ@VA-Lip-Exo system developed by Zhang et al.[160]. This innovative system employs hydroxychloroquine (HCQ) as an autophagy inhibitor to target activated HSCs. By modifying liposomes with vitamin A and fusing them with BMSC-EVs, this dual-membrane platform efficiently delivers HCQ to activated HSCs while minimizing damage to surrounding hepatic cells. Beyond HCQ’s anti-fibrotic effects, the EV component provides synergistic therapeutic benefits, such as reversing HSC activation and suppressing their proliferation and differentiation. Another cutting-edge application involves the delivery of a combined regimen of clodronate and nintedanib via EV-liposome hybrids[161]. This approach specifically targets Kupffer cells, mitigating their pro-inflammatory activity and reducing liver fibrosis progression.
EV-liposome hybrids overcome several limitations of standalone EVs or liposomes, including suboptimal bioavailability, rapid clearance, and off-target effects. A key advantage of these hybrid systems is their ability to overcome biological barriers, such as the sinusoidal endothelial capillary barrier in the liver, which often hinders the penetration of therapeutic agents. It has been shown that hybrid vesicles can effectively navigate this barrier, delivering therapeutic molecules directly to activated HSCs and reducing collagen deposition and fibrotic progression[162]. Furthermore, functionalization with biomimetic coatings, such as neutrophil membranes, increases their targeting efficiency toward fibrotic regions while aiding immune evasion. However, potential challenges and limitations of these hybrid systems, such as immune responses or unexpected interactions between the EVs and the liposomes, should be carefully evaluated in future research to ensure their safety and efficacy in liver fibrosis therapy.
CONCLUSION
Numerous studies highlight the potential of EVs from different sources as promising therapeutic tools to treat liver diseases, in particular hepatic fibrosis. Compared to the cells of origin, EVs are stable, biocompatible and act as carriers of biologically active molecules (mRNAs, proteins, and non-coding RNAs), which are transferred to the target cell, influencing its pathophysiological state. In the context of liver fibrosis, the EV mechanisms of action include suppression of the expression of TGF-β and of HSC activation, regulation of programmed cell death, and reduction of inflammation, contributing to hepatic regeneration and preservation of tissue integrity. Among the different sources, MSC-EVs demonstrate significant therapeutic potential, which can be improved using different approaches, including the preconditioning of the cell of origin, to enhance their efficacy. Various preconditioning strategies, such as exposure to pro-inflammatory cytokines, pharmacological treatments, and genetic modifications, have been explored to optimize the therapeutic efficacy of cell-derived EVs.
EVs also represent a promising drug delivery system that is able to improve the pharmacokinetic and pharmacodynamic properties of medications. Loading EVs with different types of molecules, including drugs, RNA interference molecules, and therapeutic miRNAs, offers several advantages, such as deep tissue penetration, sustained cargo release, prolonged circulation time, safety, and biocompatibility. Additionally, EV surface engineering improves their targeting ability, ensuring the precise and efficient delivery of therapeutic cargo to liver cells, in particular HSCs and macrophages, thereby alleviating liver fibrosis. Furthermore, combining natural or engineered EV formulations with existing anti-fibrotic, immunomodulatory, or anti-inflammatory treatments may further enhance their efficacy in ameliorating liver morphology and function. All these approaches could pave the way for highly specific and effective therapies, not only for liver fibrosis but also for other fibrotic diseases characterized by excessive ECM deposition.
Although EVs have demonstrated promising biological activity in several preclinical models of liver disease, further research is needed to address several challenges related to their clinical application. First, there is a need for the standardization and large-scale production of EVs in accordance with current good manufacturing practices (CGMP). Achieving CGMP compliance to produce clinical-grade EVs requires adherence to strict regulatory standards, including the establishment of a qualified cell bank. Among the available isolation methods, differential ultracentrifugation represents the most widely used; however, it is time-consuming and cost-inefficient for large-scale processing and does not align with CGMP regulations. Size-exclusion chromatography, while CGMP-compatible, lacks scalability. In the last years, tangential flow filtration has emerged as an attractive alternative due to its ability to process larger sample volumes for clinical-grade EV production, although an additional ultracentrifugation step is often required to concentrate the final product.
Another critical aspect is optimizing EV delivery to ensure effective targeting of the desired cell populations. Natural EV tropism toward certain organs, like the liver, as well as the administration regimen, can influence therapeutic efficacy. However, the sequestration of EVs within the hepatic parenchyma may reduce bioavailability and limit their interaction with key cellular components required to induce a regenerative response. Engineering techniques, such as functionalizing the EV surface with targeting moieties, could refine cell specificity and increase their therapeutic ability.
Finally, standardized quality control measures are essential to assess product stability. Defining optimal storage conditions, including temperature ranges and the use of cryopreservatives, is crucial for preserving EV bioactivity, and ensuring safety for their translation to the clinic. Furthermore, for future clinical applications, efforts should focus on clarifying EV mechanisms of action, standardizing isolation protocols, and establishing comprehensive CGMP guidelines to facilitate regulatory approval and widespread therapeutic use.
DECLARATIONS
Authors’ contributions
Conceived the idea for the manuscript: Chiabotto G, Bruno S
Drafted the manuscript: Chiabotto G, Semnani A, Ceccotti E
Edited the manuscript: Chiabotto G, Ceccotti E, Bruno S
Supervision: Bruno S
Availability of data and materials
Not applicable.
Financial support and sponsorship
None.
Conflicts of interest
All authors declared that there are no conflicts of interest.
Ethical approval and consent to participate
Not applicable.
Consent for publication
Not applicable.
Copyright
© The Author(s) 2025.
REFERENCES
1. Sharma S, Le Guillou D, Chen JY. Cellular stress in the pathogenesis of nonalcoholic steatohepatitis and liver fibrosis. Nat Rev Gastroenterol Hepatol. 2023;20:662-78.
2. Airola C, Pallozzi M, Cerrito L, et al. Microvascular thrombosis and liver fibrosis progression: mechanisms and clinical applications. Cells. 2023;12:1712.
3. Rinella ME, Neuschwander-Tetri BA, Siddiqui MS, et al. AASLD practice guidance on the clinical assessment and management of nonalcoholic fatty liver disease. Hepatology. 2023;77:1797-835.
4. Ebrahimi H, Naderian M, Sohrabpour AA. New concepts on reversibility and targeting of liver fibrosis; a review article. Middle East J Dig Dis. 2018;10:133-48.
5. Bruno S, Chiabotto G, Camussi G. Extracellular vesicles: a therapeutic option for liver fibrosis. Int J Mol Sci. 2020;21:4255.
6. Wiklander OPB, Brennan MÁ, Lötvall J, Breakefield XO, El Andaloussi S. Advances in therapeutic applications of extracellular vesicles. Sci Transl Med. 2019;11:eaav8521.
7. Chong SY, Lee CK, Huang C, et al. Extracellular vesicles in cardiovascular diseases: alternative biomarker sources, therapeutic agents, and drug delivery carriers. Int J Mol Sci. 2019;20:3272.
8. de Jong OG, Kooijmans SAA, Murphy DE, et al. Drug delivery with extracellular vesicles: from imagination to innovation. Acc Chem Res. 2019;52:1761-70.
9. Théry C, Ostrowski M, Segura E. Membrane vesicles as conveyors of immune responses. Nat Rev Immunol. 2009;9:581-93.
10. Welsh JA, Goberdhan DCI, O’Driscoll L, et al; MISEV Consortium. Minimal information for studies of extracellular vesicles (MISEV2023): from basic to advanced approaches. J Extracell Vesicles. 2024;13:e12404.
11. Kalluri R, LeBleu VS. The biology, function, and biomedical applications of exosomes. Science. 2020;367:eaau6977.
12. Mori MA, Ludwig RG, Garcia-Martin R, Brandão BB, Kahn CR. Extracellular miRNAs: from biomarkers to mediators of physiology and disease. Cell Metab. 2019;30:656-73.
13. Ogawa R, Tanaka C, Sato M, et al. Adipocyte-derived microvesicles contain RNA that is transported into macrophages and might be secreted into blood circulation. Biochem Biophys Res Commun. 2010;398:723-9.
14. Simons M, Raposo G. Exosomes--vesicular carriers for intercellular communication. Curr Opin Cell Biol. 2009;21:575-81.
15. Sarvar D, Shamsasenjan K, Akbarzadehlaleh P. Mesenchymal stem cell-derived exosomes: new opportunity in cell-free therapy. Adv Pharm Bull. 2016;6:293-9.
16. Berumen J, Baglieri J, Kisseleva T, Mekeel K. Liver fibrosis: pathophysiology and clinical implications. WIREs Mech Dis. 2021;13:e1499.
17. Stalnikowitz D, Weissbrod AB. Liver fibrosis and inflammation. A review. Ann Hepatol. 2003;2:159-63.
18. Tanaka M, Miyajima A. Liver regeneration and fibrosis after inflammation. Inflamm Regen. 2016;36:19.
19. Garbuzenko DV. Pathophysiological mechanisms of hepatic stellate cells activation in liver fibrosis. World J Clin Cases. 2022;10:3662-76.
20. Cai X, Wang J, Wang J, et al. Intercellular crosstalk of hepatic stellate cells in liver fibrosis: new insights into therapy. Pharmacol Res. 2020;155:104720.
21. Matsuda M, Seki E. Hepatic stellate cell-macrophage crosstalk in liver fibrosis and carcinogenesis. Semin Liver Dis. 2020;40:307-20.
22. Roehlen N, Crouchet E, Baumert TF. Liver fibrosis: mechanistic concepts and therapeutic perspectives. Cells. 2020;9:875.
23. Iredale JP, Thompson A, Henderson NC. Extracellular matrix degradation in liver fibrosis: biochemistry and regulation. Biochim Biophys Acta. 2013;1832:876-83.
24. Bonnans C, Chou J, Werb Z. Remodelling the extracellular matrix in development and disease. Nat Rev Mol Cell Biol. 2014;15:786-801.
25. Saki S, Monjezi S, Ghaffari F, et al. Unraveling the exosome-miR-133a axis: targeting TGF-β signaling via WJ-MSC-derived exosomes for anti-fibrotic therapy in liver fibrosis. Iran Biomed J. 2024;28:235-44.
26. Afarin R, Behdarvand T, Shakerian E, Salehipour Bavarsad S, Rashidi M. Exosomes of Whartons’ jelly mesenchymal stem cell reduce the NOX genes in TGF-β-induced hepatic fibrosis. Iran J Basic Med Sci. 2022;25:1498-503.
27. Rashidi M, Matour E, Monjezi S, et al. Effects of exosomes of mesenchymal stem cells on cholesterol-induced hepatic fibrogenesis. Iran J Basic Med Sci. 2023;26:695-700.
28. Torabi S, Zarrabi M, Shekari F, et al. Wharton’s Jelly mesenchymal stem cell-derived extracellular vesicles induce liver fibrosis-resolving phenotype in alternatively activated macrophages. J Cell Mol Med. 2024;28:e18507.
29. Zong R, Zheng Y, Yan Y, et al. Mesenchymal stem cells-derived exosomes alleviate liver fibrosis by targeting Hedgehog/SMO signaling. Hepatol Int. 2024;18:1781-91.
30. Li T, Yan Y, Wang B, et al. Exosomes derived from human umbilical cord mesenchymal stem cells alleviate liver fibrosis. Stem Cells Dev. 2013;22:845-54.
31. Yuan M, Yao L, Chen P, et al. Human umbilical cord mesenchymal stem cells inhibit liver fibrosis via the microRNA-148a-5p/SLIT3 axis. Int Immunopharmacol. 2023;125:111134.
32. Cheng F, Yang F, Wang Y, Zhou J, Qian H, Yan Y. Mesenchymal stem cell-derived exosomal miR-27b-3p alleviates liver fibrosis via downregulating YAP/LOXL2 pathway. J Nanobiotechnology. 2023;21:195.
33. Wang Y, Wang C, Yang F, et al. USP9X-enriched MSC-sEV inhibits LSEC angiogenesis in MASH mice by downregulating the IκBα/NF-κB/Ang-2 pathway. Pharmacol Res. 2024;209:107471.
34. Dong L, Pu Y, Chen X, et al. hUCMSC-extracellular vesicles downregulated hepatic stellate cell activation and reduced liver injury in S. japonicum-infected mice. Stem Cell Res Ther. 2020;11:21.
35. Huang YJ, Cao J, Lee CY, Wu YM. Umbilical cord blood plasma-derived exosomes as a novel therapy to reverse liver fibrosis. Stem Cell Res Ther. 2021;12:568.
36. Jiang W, Tan Y, Cai M, et al. Human umbilical cord MSC-derived exosomes suppress the development of CCl4-induced liver injury through antioxidant effect. Stem Cells Int. 2018;2018:6079642.
37. Sani F, Soufi Zomorrod M, Azarpira N, Soleimani M. The effect of mesenchymal stem cell-derived exosomes and miR17-5p inhibitor on multicellular liver fibrosis microtissues. Stem Cells Int. 2023;2023:8836452.
38. Chiabotto G, Semnani A, Ceccotti E, Guenza M, Camussi G, Bruno S. Mesenchymal stromal cell-derived extracellular vesicles for reversing hepatic fibrosis in 3D liver spheroids. Biomedicines. 2024;12:1849.
39. Tan Y, Huang Y, Mei R, et al. HucMSC-derived exosomes delivered BECN1 induces ferroptosis of hepatic stellate cells via regulating the xCT/GPX4 axis. Cell Death Dis. 2022;13:319.
40. Tian S, Zhou X, Zhang M, et al. Mesenchymal stem cell-derived exosomes protect against liver fibrosis via delivering miR-148a to target KLF6/STAT3 pathway in macrophages. Stem Cell Res Ther. 2022;13:330.
41. Shi Y, Yang X, Wang S, et al. Human umbilical cord mesenchymal stromal cell-derived exosomes protect against MCD-induced NASH in a mouse model. Stem Cell Res Ther. 2022;13:517.
42. Zheng W, Bian S, Qiu S, et al. Placenta mesenchymal stem cell-derived extracellular vesicles alleviate liver fibrosis by inactivating hepatic stellate cells through a miR-378c/SKP2 axis. Inflamm Regen. 2023;43:47.
43. Alhomrani M, Correia J, Zavou M, et al. The human amnion epithelial cell secretome decreases hepatic fibrosis in mice with chronic liver fibrosis. Front Pharmacol. 2017;8:748.
44. Ohara M, Ohnishi S, Hosono H, et al. Extracellular vesicles from amnion-derived mesenchymal stem cells ameliorate hepatic inflammation and fibrosis in rats. Stem Cells Int. 2018;2018:3212643.
45. Xu AL, Han L, Yan J, Liu D, Wang W. Effects of mesenchymal stem cells-derived extracellular vesicles on inhibition of hepatic fibrosis by delivering miR-200a. Tissue Eng Regen Med. 2024;21:609-24.
46. Wang N, Li X, Zhong Z, et al. 3D hESC exosomes enriched with miR-6766-3p ameliorates liver fibrosis by attenuating activated stellate cells through targeting the TGFβRII-SMADS pathway. J Nanobiotechnology. 2021;19:437.
47. Rong X, Liu J, Yao X, Jiang T, Wang Y, Xie F. Human bone marrow mesenchymal stem cells-derived exosomes alleviate liver fibrosis through the Wnt/β-catenin pathway. Stem Cell Res Ther. 2019;10:98.
48. Rostom DM, Attia N, Khalifa HM, Abou Nazel MW, El Sabaawy EA. The therapeutic potential of extracellular vesicles versus mesenchymal stem cells in liver damage. Tissue Eng Regen Med. 2020;17:537-52.
49. Sun C, Shi C, Duan X, Zhang Y, Wang B. Exosomal microRNA-618 derived from mesenchymal stem cells attenuate the progression of hepatic fibrosis by targeting Smad4. Bioengineered. 2022;13:5915-27.
50. Ma J, Li Y, Chen M, et al. hMSCs-derived exosome circCDK13 inhibits liver fibrosis by regulating the expression of MFGE8 through miR-17-5p/KAT2B. Cell Biol Toxicol. 2023;39:1-22.
51. An SY, Jang YJ, Lim HJ, et al. Milk fat globule-EGF factor 8, secreted by mesenchymal stem cells, protects against liver fibrosis in mice. Gastroenterology. 2017;152:1174-86.
52. Didamoony MA, Atwa AM, Ahmed LA. Modulatory effect of rupatadine on mesenchymal stem cell-derived exosomes in hepatic fibrosis in rats: a potential role for miR-200a. Life Sci. 2023;324:121710.
53. Zhao S, Liu Y, Pu Z. Bone marrow mesenchymal stem cell-derived exosomes attenuate D-GaIN/LPS-induced hepatocyte apoptosis by activating autophagy in vitro. Drug Des Devel Ther. 2019;13:2887-97.
54. Zhang Y, Zhangdi H, Nie X, et al. Exosomes derived from BMMSCs mitigate the hepatic fibrosis via anti-pyroptosis pathway in a cirrhosis model. Cells. 2022;11:4004.
55. Feng X, Feng B, Zhou J, et al. Mesenchymal stem cells alleviate mouse liver fibrosis by inhibiting pathogenic function of intrahepatic B cells. Hepatology. 2025;81:1211-27.
56. Ellakany AR, El Baz H, Shoheib ZS, Elzallat M, Ashour DS, Yassen NA. Stem cell-derived exosomes as a potential therapy for schistosomal hepatic fibrosis in experimental animals. Pathog Glob Health. 2024;118:429-49.
57. Han HS, Lee H, You D, et al. Human adipose stem cell-derived extracellular nanovesicles for treatment of chronic liver fibrosis. J Control Release. 2020;320:328-36.
58. Zhang Z, Shang J, Yang Q, et al. Exosomes derived from human adipose mesenchymal stem cells ameliorate hepatic fibrosis by inhibiting PI3K/Akt/mTOR pathway and remodeling choline metabolism. J Nanobiotechnology. 2023;21:29.
59. Al Saihati HA, Badr OA, Dessouky AA, et al. Exploring the cytoprotective role of mesenchymal stem cell-derived exosomes in chronic liver fibrosis: insights into the Nrf2/Keap1/p62 signaling pathway. Int Immunopharmacol. 2024;141:112934.
60. Wu B, Feng J, Guo J, et al. ADSCs-derived exosomes ameliorate hepatic fibrosis by suppressing stellate cell activation and remodeling hepatocellular glutamine synthetase-mediated glutamine and ammonia homeostasis. Stem Cell Res Ther. 2022;13:494.
61. Du Z, Wu T, Liu L, Luo B, Wei C. Extracellular vesicles-derived miR-150-5p secreted by adipose-derived mesenchymal stem cells inhibits CXCL1 expression to attenuate hepatic fibrosis. J Cell Mol Med. 2021;25:701-15.
62. Gan L, Zheng L, Yao L, et al. Exosomes from adipose-derived mesenchymal stem cells improve liver fibrosis by regulating the miR-20a-5p/TGFBR2 axis to affect the p38 MAPK/NF-κB pathway. Cytokine. 2023;172:156386.
63. Takeda N, Tsuchiya A, Mito M, et al. Analysis of distribution, collection, and confirmation of capacity dependency of small extracellular vesicles toward a therapy for liver cirrhosis. Inflamm Regen. 2023;43:48.
64. Watanabe T, Tsuchiya A, Takeuchi S, et al. Development of a non-alcoholic steatohepatitis model with rapid accumulation of fibrosis, and its treatment using mesenchymal stem cells and their small extracellular vesicles. Regen Ther. 2020;14:252-61.
65. Kim J, Lee C, Shin Y, et al. sEVs from tonsil-derived mesenchymal stromal cells alleviate activation of hepatic stellate cells and liver fibrosis through miR-486-5p. Mol Ther. 2021;29:1471-86.
66. Bruno S, Pasquino C, Herrera Sanchez MB, et al. HLSC-derived extracellular vesicles attenuate liver fibrosis and inflammation in a murine model of non-alcoholic steatohepatitis. Mol Ther. 2020;28:479-89.
67. Chiabotto G, Ceccotti E, Pasquino C, et al. Human liver stem cell-derived extracellular vesicles modulate long non-coding RNA expression profile in an in vivo model of non-alcoholic steatohepatitis. Explor Dig Dis. 2023;2:172-87.
68. Chiabotto G, Ceccotti E, Tapparo M, Camussi G, Bruno S. Human liver stem cell-derived extracellular vesicles target hepatic stellate cells and attenuate their pro-fibrotic phenotype. Front Cell Dev Biol. 2021;9:777462.
69. Hu Z, Zhao Y, Jiang J, et al. Exosome-derived miR-142-5p from liver stem cells improves the progression of liver fibrosis by regulating macrophage polarization through CTSB. Environ Toxicol. 2023;38:1860-73.
70. Frangogiannis N. Transforming growth factor-β in tissue fibrosis. J Exp Med. 2020;217:e20190103.
71. Carthy JM. TGFβ signaling and the control of myofibroblast differentiation: implications for chronic inflammatory disorders. J Cell Physiol. 2018;233:98-106.
72. Russo E, Alberti G, Corrao S, et al. The truth is out there: biological features and clinical indications of extracellular vesicles from human perinatal stem cells. Cells. 2023;12:2347.
73. Hazrati A, Malekpour K, Soudi S, Hashemi SM. Mesenchymal stromal/stem cells and their extracellular vesicles application in acute and chronic inflammatory liver diseases: emphasizing on the anti-fibrotic and immunomodulatory mechanisms. Front Immunol. 2022;13:865888.
74. Tan CY, Lai RC, Wong W, Dan YY, Lim SK, Ho HK. Mesenchymal stem cell-derived exosomes promote hepatic regeneration in drug-induced liver injury models. Stem Cell Res Ther. 2014;5:76.
75. Gómez-Virgilio L, Silva-Lucero MD, Flores-Morelos DS, et al. Autophagy: a key regulator of homeostasis and disease: an overview of molecular mechanisms and modulators. Cells. 2022;11:2262.
76. Talukdar S, Das SK, Emdad L, Fisher PB. Autophagy and senescence: insights from normal and cancer stem cells. Adv Cancer Res. 2021;150:147-208.
77. Mao YQ, Fan XM. Autophagy: a new therapeutic target for liver fibrosis. World J Hepatol. 2015;7:1982-6.
78. Hazari Y, Bravo-San Pedro JM, Hetz C, Galluzzi L, Kroemer G. Autophagy in hepatic adaptation to stress. J Hepatol. 2020;72:183-96.
79. Wang C, Ma C, Gong L, et al. Macrophage polarization and its role in liver disease. Front Immunol. 2021;12:803037.
80. Arora S, Dev K, Agarwal B, Das P, Syed MA. Macrophages: their role, activation and polarization in pulmonary diseases. Immunobiology. 2018;223:383-96.
81. Xue JD, Gao J, Tang AF, Feng C. Shaping the immune landscape: multidimensional environmental stimuli refine macrophage polarization and foster revolutionary approaches in tissue regeneration. Heliyon. 2024;10:e37192.
82. Hu Q, Lyon CJ, Fletcher JK, Tang W, Wan M, Hu TY. Extracellular vesicle activities regulating macrophage- and tissue-mediated injury and repair responses. Acta Pharm Sin B. 2021;11:1493-512.
83. Wang J, Wu Z, Xia M, et al. Extracellular vesicles derived from liver sinusoidal endothelial cells inhibit the activation of hepatic stellate cells and Kupffer cells in vitro. Biochim Biophys Acta Mol Basis Dis. 2024;1870:167020.
84. Chen L, Chen R, Kemper S, Brigstock DR. Pathways of production and delivery of hepatocyte exosomes. J Cell Commun Signal. 2018;12:343-57.
85. Li X, Chen R, Kemper S, Brigstock DR. Extracellular vesicles from hepatocytes are therapeutic for toxin-mediated fibrosis and gene expression in the liver. Front Cell Dev Biol. 2019;7:368.
86. Liu W, Wu J, Cao H, et al. Human-induced hepatocytes-derived extracellular vesicles ameliorated liver fibrosis in mice via suppression of TGF-β1/smad signaling and activation of Nrf2/HO-1 signaling. Stem Cells Dev. 2023;32:638-51.
87. Zhong Z, Cui XL, Tan KJ, et al. Apoptotic vesicles (apoVs) derived from fibroblast-converted hepatocyte-like cells effectively ameliorate liver fibrosis. J Nanobiotechnology. 2024;22:541.
88. Povero D, Pinatel EM, Leszczynska A, et al. Human induced pluripotent stem cell-derived extracellular vesicles reduce hepatic stellate cell activation and liver fibrosis. JCI Insight. 2019;5:125652.
89. Wang L, Wang Y, Quan J. Exosomes derived from natural killer cells inhibit hepatic stellate cell activation and liver fibrosis. Hum Cell. 2020;33:582-9.
90. Chen L, Chen R, Kemper S, Cong M, You H, Brigstock DR. Therapeutic effects of serum extracellular vesicles in liver fibrosis. J Extracell Vesicles. 2018;7:1461505.
91. Maeda Y, Watanabe Y, Ishikawa N, et al. Platelet-rich plasma-derived extracellular vesicles improve liver cirrhosis in mice. Regen Ther. 2024;26:1048-57.
92. Reif S, Atias A, Musseri M, Koroukhov N, Gerstl RG. Beneficial effects of milk-derived extracellular vesicles on liver fibrosis progression by inhibiting hepatic stellate cell activation. Nutrients. 2022;14:4049.
93. Gong Q, Zeng Z, Jiang T, et al. Anti-fibrotic effect of extracellular vesicles derived from tea leaves in hepatic stellate cells and liver fibrosis mice. Front Nutr. 2022;9:1009139.
94. Kim JS, Eom JY, Kim HW, et al. Hemp sprout-derived exosome-like nanovesicles as hepatoprotective agents attenuate liver fibrosis. Biomater Sci. 2024;12:5361-71.
95. Keshavarz Azizi Raftar S, Ashrafian F, Yadegar A, et al. The protective effects of live and pasteurized akkermansia muciniphila and its extracellular vesicles against HFD/CCl4-induced liver injury. Microbiol Spectr. 2021;9:e0048421.
96. Xu F, Liu J, Deng J, et al. Rapid and high-efficiency generation of mature functional hepatocyte-like cells from adipose-derived stem cells by a three-step protocol. Stem Cell Res Ther. 2015;6:193.
97. Zhong Z, Du J, Zhu X, et al. Highly efficient conversion of mouse fibroblasts into functional hepatic cells under chemical induction. J Mol Cell Biol. 2024;15:mjad071.
98. Calzoni E, Bertoldi A, Cusumano G, Buratta S, Urbanelli L, et al. Plant-derived extracellular vesicles: natural nanocarriers for biotechnological drugs. Processes. 2024;12:2938.
99. Shao M, Jin X, Chen S, Yang N, Feng G. Plant-derived extracellular vesicles -a novel clinical anti-inflammatory drug carrier worthy of investigation. Biomed Pharmacother. 2023;169:115904.
100. Zhang S, Wang Q, Tan DEL, et al. Gut-liver axis: potential mechanisms of action of food-derived extracellular vesicles. J Extracell Vesicles. 2024;13:e12466.
101. Yang Y, Wu Y, Yang D, et al. Secretive derived from hypoxia preconditioned mesenchymal stem cells promote cartilage regeneration and mitigate joint inflammation via extracellular vesicles. Bioact Mater. 2023;27:98-112.
102. Bader AM, Klose K, Bieback K, et al. Hypoxic preconditioning increases survival and pro-angiogenic capacity of human cord blood mesenchymal stromal cells in vitro. PLoS One. 2015;10:e0138477.
103. Duijvestein M, Wildenberg ME, Welling MM, et al. Pretreatment with interferon-γ enhances the therapeutic activity of mesenchymal stromal cells in animal models of colitis. Stem Cells. 2011;29:1549-58.
104. François M, Romieu-Mourez R, Li M, Galipeau J. Human MSC suppression correlates with cytokine induction of indoleamine 2,3-dioxygenase and bystander M2 macrophage differentiation. Mol Ther. 2012;20:187-95.
105. Noone C, Kihm A, English K, O’Dea S, Mahon BP. IFN-γ stimulated human umbilical-tissue-derived cells potently suppress NK activation and resist NK-mediated cytotoxicity in vitro. Stem Cells Dev. 2013;22:3003-14.
106. Ragni E, Perucca Orfei C, De Luca P, et al. Inflammatory priming enhances mesenchymal stromal cell secretome potential as a clinical product for regenerative medicine approaches through secreted factors and EV-miRNAs: the example of joint disease. Stem Cell Res Ther. 2020;11:165.
107. Lim J, Lee S, Ju H, et al. Valproic acid enforces the priming effect of sphingosine-1 phosphate on human mesenchymal stem cells. Int J Mol Med. 2017;40:739-47.
108. Lee S, Lim J, Lee JH, et al. Ascorbic acid 2-glucoside stably promotes the primitiveness of embryonic and mesenchymal stem cells through ten-eleven translocation- and cAMP-responsive element-binding protein-1-dependent mechanisms. Antioxid Redox Signal. 2020;32:35-59.
109. Kang H, Kim KH, Lim J, et al. The therapeutic effects of human mesenchymal stem cells primed with sphingosine-1 phosphate on pulmonary artery hypertension. Stem Cells Dev. 2015;24:1658-71.
110. Damasceno PKF, de Santana TA, Santos GC, et al. Genetic engineering as a strategy to improve the therapeutic efficacy of mesenchymal stem/stromal cells in regenerative medicine. Front Cell Dev Biol. 2020;8:737.
111. Chen S, Sun F, Qian H, Xu W, Jiang J. Preconditioning and engineering strategies for improving the efficacy of mesenchymal stem cell-derived exosomes in cell-free therapy. Stem Cells Int. 2022;2022:1779346.
112. Takeuchi S, Tsuchiya A, Iwasawa T, et al. Small extracellular vesicles derived from interferon-γ pre-conditioned mesenchymal stromal cells effectively treat liver fibrosis. NPJ Regen Med. 2021;6:19.
113. Bavarsad S, Jalali MT, Bijan Nejad D, Alypoor B, Babaahmadi Rezaei H, Mohammadtaghvaei N. TGFβ1-pretreated exosomes of wharton jelly mesenchymal stem cell as a therapeutic strategy for improving liver fibrosis. Hepat Mon. 2022;22:e123416.
114. Tawfeek GA, Kasem HA. Curcumin preconditioned mesenchymal stem cells derived exosomes transplantation ameliorate and protect against non- alcoholic steatohepatitis by regulation the expression of key genes of inflammation and oxidative stress. Transpl Immunol. 2023;78:101837.
115. Jiang X, Liu Z, You H, et al. Quercetin-primed BMSC-derived extracellular vesicles ameliorate chronic liver damage through miR-136-5p and GNAS/STAT3 signaling pathways. Int Immunopharmacol. 2024;142:113162.
116. Lang Z, Li Y, Lin L, et al. Hepatocyte-derived exosomal miR-146a-5p inhibits hepatic stellate cell EMT process: a crosstalk between hepatocytes and hepatic stellate cells. Cell Death Discov. 2023;9:304.
117. Chen L, Lu FB, Chen DZ, et al. BMSCs-derived miR-223-containing exosomes contribute to liver protection in experimental autoimmune hepatitis. Mol Immunol. 2018;93:38-46.
118. Tian X, Wu L, Li X, Zheng W, Zuo H, Song H. Exosomes derived from bone marrow mesenchymal stem cells alleviate biliary ischemia reperfusion injury in fatty liver transplantation by inhibiting ferroptosis. Mol Cell Biochem. 2024;479:881-94.
119. Xuan J, Xu H, Li H, et al. Extracellular vesicles from miR-148a-5p-enriched bone marrow mesenchymal stem cells relieve hepatic fibrosis by targeting Smad4. Mol Biotechnol. 2022;64:535-45.
120. Ma L, Wei J, Zeng Y, et al. Mesenchymal stem cell-originated exosomal circDIDO1 suppresses hepatic stellate cell activation by miR-141-3p/PTEN/AKT pathway in human liver fibrosis. Drug Deliv. 2022;29:440-53.
121. Qu Y, Zhang Q, Cai X, et al. Exosomes derived from miR-181-5p-modified adipose-derived mesenchymal stem cells prevent liver fibrosis via autophagy activation. J Cell Mol Med. 2017;21:2491-502.
122. Lou G, Yang Y, Liu F, et al. MiR-122 modification enhances the therapeutic efficacy of adipose tissue-derived mesenchymal stem cells against liver fibrosis. J Cell Mol Med. 2017;21:2963-73.
123. Yu L, Xue J, Wu Y, Zhou H. Therapeutic effect of exosomes derived from hepatocyte-growth-factor-overexpressing adipose mesenchymal stem cells on liver injury. Folia Histochem Cytobiol. 2023;61:160-71.
124. Wang J, Ye W, Jiang M, Zhou Y, Zheng J. Therapeutic potential of exosome derived from hepatocyte growth factor-overexpressing adipose mesenchymal stem cells in TGFβ1-stimulated hepatic stellate cells. Cytotechnology. 2024;76:217-29.
125. Domínguez LM, Bueloni B, Cantero MJ, et al. Chromatographic scalable method to isolate engineered extracellular vesicles derived from mesenchymal stem cells for the treatment of liver fibrosis in mice. Int J Mol Sci. 2023;24:9586.
126. Fiore E, Domínguez LM, Bayo J, et al. Human umbilical cord perivascular cells-derived extracellular vesicles mediate the transfer of IGF-I to the liver and ameliorate hepatic fibrogenesis in mice. Gene Ther. 2020;27:62-73.
127. Zhu D, Sun Z, Wei J, et al. BMP7-loaded human umbilical cord mesenchymal stem cell-derived small extracellular vesicles ameliorate liver fibrosis by targeting activated hepatic stellate cells. Int J Nanomedicine. 2024;19:3475-95.
128. Wang Y, Chen Y, Yang F, et al. MiR-4465-modified mesenchymal stem cell-derived small extracellular vesicles inhibit liver fibrosis development via targeting LOXL2 expression. J Zhejiang Univ Sci B. 2024;25:594-604.
129. Li T, Li X, Han G, et al. The therapeutic potential and clinical significance of exosomes as carriers of drug delivery system. Pharmaceutics. 2022;15:21.
130. Herrmann IK, Wood MJA, Fuhrmann G. Extracellular vesicles as a next-generation drug delivery platform. Nat Nanotechnol. 2021;16:748-59.
131. Xie H, Sun L, Zhang L, et al. Mesenchymal stem cell-derived microvesicles support ex vivo expansion of cord blood-derived CD34+ cells. Stem Cells Int. 2016;2016:6493241.
132. Azizsoltani A, Hatami B, Zali MR, Mahdavi V, Baghaei K, Alizadeh E. Obeticholic acid-loaded exosomes attenuate liver fibrosis through dual targeting of the FXR signaling pathway and ECM remodeling. Biomed Pharmacother. 2023;168:115777.
133. Niknam B, Baghaei K, Mahmoud Hashemi S, Hatami B, Reza Zali M, Amani D. Human Wharton’s jelly mesenchymal stem cells derived-exosomes enriched by miR-124 promote an anti-fibrotic response in an experimental model of liver fibrosis. Int Immunopharmacol. 2023;119:110294.
134. Tang M, Chen Y, Li B, et al. Therapeutic targeting of STAT3 with small interference RNAs and antisense oligonucleotides embedded exosomes in liver fibrosis. FASEB J. 2021;35:e21557.
135. Ashour AA, El-Kamel AH, Mehanna RA, Mourad G, Heikal LA. Luteolin-loaded exosomes derived from bone marrow mesenchymal stem cells: a promising therapy for liver fibrosis. Drug Deliv. 2022;29:3270-80.
136. Tang M, Guo C, Sun M, et al. Effective delivery of osteopontin small interference RNA using exosomes suppresses liver fibrosis via TGF-β1 signaling. Front Pharmacol. 2022;13:882243.
137. You DG, Oh BH, Nguyen VQ, et al. Vitamin A-coupled stem cell-derived extracellular vesicles regulate the fibrotic cascade by targeting activated hepatic stellate cells in vivo. J Control Release. 2021;336:285-95.
138. Yang H, Wang W, Xiao J, et al. ROS-responsive injectable hydrogels loaded with exosomes carrying miR-4500 reverse liver fibrosis. Biomaterials. 2025;314:122887.
139. Luo N, Li J, Chen Y, et al. Hepatic stellate cell reprogramming via exosome-mediated CRISPR/dCas9-VP64 delivery. Drug Deliv. 2021;28:10-8.
140. Wan T, Zhong J, Pan Q, Zhou T, Ping Y, Liu X. Exosome-mediated delivery of Cas9 ribonucleoprotein complexes for tissue-specific gene therapy of liver diseases. Sci Adv. 2022;8:eabp9435.
141. Zhao Y, Yu Y, Wang S, Li J, Teng L. Small extracellular vesicles encapsulating lefty1 mRNA inhibit hepatic fibrosis. Asian J Pharm Sci. 2022;17:630-40.
142. Yu F, Liu Z, Feng J, et al. Hyaluronic acid modified extracellular vesicles targeting hepatic stellate cells to attenuate hepatic fibrosis. Eur J Pharm Sci. 2024;198:106783.
143. Gong L, Zhou H, Zhang S, et al. CD44-targeting drug delivery system of exosomes loading forsythiaside a combats liver fibrosis via regulating NLRP3-mediated pyroptosis. Adv Healthc Mater. 2023;12:e2202228.
144. Gong L, Zhou H, Zhang Y, et al. Preparation of phillygenin-hyaluronic acid composite milk-derived exosomes and its anti-hepatic fibrosis effect. Mater Today Bio. 2023;23:100804.
145. Albaladejo-García V, Morán L, Santos-Coquillat A, et al. Curcumin encapsulated in milk small extracellular vesicles as a nanotherapeutic alternative in experimental chronic liver disease. Biomed Pharmacother. 2024;173:116381.
146. Zhang G, Huang X, Xiu H, et al. Extracellular vesicles: natural liver-accumulating drug delivery vehicles for the treatment of liver diseases. J Extracell Vesicles. 2020;10:e12030.
147. Cecchin R, Troyer Z, Witwer K, Morris KV. Extracellular vesicles: the next generation in gene therapy delivery. Mol Ther. 2023;31:1225-30.
148. Hu C, Zhao L, Zhang L, Bao Q, Li L. Mesenchymal stem cell-based cell-free strategies: safe and effective treatments for liver injury. Stem Cell Res Ther. 2020;11:377.
149. Chakraborty D, Šumová B, Mallano T, et al. Activation of STAT3 integrates common profibrotic pathways to promote fibroblast activation and tissue fibrosis. Nat Commun. 2017;8:1130.
150. Xiang DM, Sun W, Ning BF, et al. The HLF/IL-6/STAT3 feedforward circuit drives hepatic stellate cell activation to promote liver fibrosis. Gut. 2018;67:1704-15.
151. Li J, Li X, Xu W, et al. Antifibrotic effects of luteolin on hepatic stellate cells and liver fibrosis by targeting AKT/mTOR/p70S6K and TGFβ/Smad signalling pathways. Liver Int. 2015;35:1222-33.
152. Cummins CB, Wang X, Nunez Lopez O, et al. Luteolin-mediated inhibition of hepatic stellate cell activation via suppression of the STAT3 pathway. Int J Mol Sci. 2018;19:1567.
153. Lorena D, Darby IA, Gadeau AP, et al. Osteopontin expression in normal and fibrotic liver. altered liver healing in osteopontin-deficient mice. J Hepatol. 2006;44:383-90.
154. Tang M, Jia H, Chen S, et al. Significance of MR/OPN/HMGB1 axis in NAFLD-associated hepatic fibrogenesis. Life Sci. 2021;264:118619.
155. Nevzorova YA, Bangen JM, Hu W, et al. Cyclin E1 controls proliferation of hepatic stellate cells and is essential for liver fibrogenesis in mice. Hepatology. 2012;56:1140-9.
156. Chiangjong W, Netsirisawan P, Hongeng S, Chutipongtanate S. Red blood cell extracellular vesicle-based drug delivery: challenges and opportunities. Front Med. 2021;8:761362.
157. Rodríguez DA, Vader P. Extracellular vesicle-based hybrid systems for advanced drug delivery. Pharmaceutics. 2022;14:267.
158. Piffoux M, Silva AKA, Wilhelm C, Gazeau F, Tareste D. Modification of extracellular vesicles by fusion with liposomes for the design of personalized biogenic drug delivery systems. ACS Nano. 2018;12:6830-42.
159. der Koog L, Gandek TB, Nagelkerke A. Liposomes and extracellular vesicles as drug delivery systems: a comparison of composition, pharmacokinetics, and functionalization. Adv Healthc Mater. 2022;11:e2100639.
160. Zhang YW, Hou LS, Xing JH, Zhang TR, Zhou SY, Zhang BL. Two-membrane hybrid nanobiomimetic delivery system for targeted autophagy inhibition of activated hepatic stellate cells to synergistically treat liver fibrosis. ACS Appl Mater Interfaces. ;2023:50863-77.
161. Ji K, Fan M, Huang D, et al. Clodronate-nintedanib-loaded exosome-liposome hybridization enhances the liver fibrosis therapy by inhibiting Kupffer cell activity. Biomater Sci. 2022;10:702-13.
Cite This Article

How to Cite
Download Citation
Export Citation File:
Type of Import
Tips on Downloading Citation
Citation Manager File Format
Type of Import
Direct Import: When the Direct Import option is selected (the default state), a dialogue box will give you the option to Save or Open the downloaded citation data. Choosing Open will either launch your citation manager or give you a choice of applications with which to use the metadata. The Save option saves the file locally for later use.
Indirect Import: When the Indirect Import option is selected, the metadata is displayed and may be copied and pasted as needed.
About This Article
Copyright
Data & Comments
Data
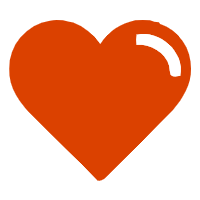
Comments
Comments must be written in English. Spam, offensive content, impersonation, and private information will not be permitted. If any comment is reported and identified as inappropriate content by OAE staff, the comment will be removed without notice. If you have any queries or need any help, please contact us at [email protected].