Improved thermoelectric performance by grain connectivity in bismuth antimony telluride composite with metallic high-entropy alloy nanoparticles
Abstract
We investigated the anisotropic thermoelectric properties of Bi0.4Sb1.6Te3 (BST) composites with heavy metallic high-entropy alloy TaNb2HfZrTi (HEAx) (x = 0, 0.1, 0.5, and 1.0 vol%), synthesized by ball-milling, mixing, and
Keywords
INTRODUCTION
Thermoelectric (TE) devices, which can directly enable waste heat recovery or eco-friendly solid-state cooling through the Seebeck effect and Peltier effect, respectively, have a wide range of interesting application fields. These include thermoelectric generators (TEGs), thermoelectric cooling devices, wearable TE devices, power systems for the Internet of Things (IoT), medical devices requiring sensitive temperature control, radioisotope thermoelectric generators (RTGs), battery thermal management systems for electric vehicles, and more[1-3]. Despite their advantages, such as direct energy conversion between heat and electricity, absence of moving parts, scalability, portability, eco-friendly operation, and low-grade heat recovery, the use of TE devices is limited in certain applications due to their relatively low energy conversion efficiency compared to other electricity generation or cooling systems[1,2,4]. Therefore, improving the thermoelectric performance of TE devices is essential to expand their range of applications.
The energy conversion efficiency of a TE device strongly depends on the dimensionless figure of merit (ZT) of the p- and n-type TE materials, defined as ZT = S2σT/κtotal, where S is the Seebeck coefficient, σ is the electrical conductivity, T is the absolute temperature and κtotal is the total thermal conductivity. Since these parameters are strongly interrelated, careful control of the material properties is required to enhance TE performance[1,2,4]. Various approaches can improve materials’ TE performance, such as optimizing carrier concentration, reducing lattice thermal conductivity (κL), manipulating the band structure, employing
Bismuth tellurides are among the most well-known thermoelectric materials, exhibiting high performance near room temperature[1,4,6,7]. Their high ZT values have been achieved through various innovative approaches for the p-type bismuth antimony tellurides such as the hot-deformed Bi0.3Sb1.7Te3 (1.3 at
During two decades, the phonon-glass and electron-crystal (PGEC) concept has been investigated as of critical importance in thermoelectric materials development[15]. The PGEC in Bismuth Tellurides has been adopted by incorporating nanoparticle distribution in the matrix. However, the nanoparticle distribution in the Bi0.4Sb1.6Te3 (BST) matrix mainly focused on the decreasing κL rather than the increase of electrical transport property. Here we adopt the heavy metallic high entropy alloy composite to realize the PGEC in the BST matrix.
High-entropy alloys (HEAs), which consist of the multi-component mixing of five or more elements in near-equimolar concentrations, are considered promising candidates for high-performance thermoelectric materials. Their potential arises from low κL due to lattice distortions caused by atomic mass and size mismatches[16,17]. HEAs exhibit unique properties such as exceptional strength, ductility, and thermal stability, making them attractive for thermoelectric applications in extreme environmental conditions[18-20].
Recent advances in TE performance using the HEA concept have been introduced, including the n-type PbSe-based high-entropy material (Pb0.89Sb0.012Sn0.1Se0.5Te0.25S0.25, ZT = 1.8 at 900 K)[21], p-type PbSe-based high-entropy material (Pb0.935Na0.025Cd0.04Se0.5S0.25Te0.25, ZT = 2.0 at 900 K)[22], and p-type GeTe based
Here we investigated the anisotropic thermoelectric properties of the composites consisting of p-type bismuth antimony telluride (BST: Te-excess Bi0.4Sb1.6Te3) and heavy metallic HEA nanoparticles (HEA: TaNb2HfZrTi). Since the Te-excess effectively enhances the TE performance of the p-type bismuth antimony telluride[9,11], the composition of the Bi0.4Sb1.6Te3 with additional Te was used for the BST matrix. The TaNb2HfZrTi is one of the representative HEAs with a body-centered cubic (BCC) phase and high mechanical strength[24]. To minimize a chemical doping effect, which can decrease the ZT value by deviating from the optimized carrier concentration, a mixed powder with the BST and HEA was used in the hot-press sintering process. The carrier concentrations, Seebeck coefficients (S), and Fermi energies (EF) of theBST+HEAx (x = 0, 0.1, 0.5, 1.0 vol%) samples are not significantly affected by the addition of HEA. On the other hand, the significantly enhanced electrical conductivity σ and reduced κL are observed in the x = 0.1 vol% sample for the parallel (Pa) direction to the hot-press direction. The enhanced Pa-direction σ and the decrease of κL of theBST+HEAx samples are mainly affected by the enlarged carrier mean free paths λe and decrease of phonon mean free path λph, which is a realization of PGEC[15]. The increased λe can be explained by the improved grain connectivity due to the HEA nanoparticles for the Pa-direction of the BST grains. Grain connectivity, which refers to the interconnection between grains, has a significant impact on transport characteristics[25-29]. The increased λph in theBST+HEAx samples further supports that the HEA nanoparticles can improve the grain connectivity of the BST grains. As a result, the ZT values of theBST+HEAx samples are enhanced from 1.09 (x = 0) to 1.33 (x = 0.1 vol%) at 350 K in the Pa-direction due to the improved σ and reduced κL. These results clearly show that incorporating HEA nanoparticles is a promising strategy for improving the performance of the anisotropic thermoelectric materials. Additionally, controlling of the grain connectivity could be a good concept to improve the transport characteristics in various materials such as superconductors[30], batteries[31,32], solar cells[33,34], and semiconductors[35,36].
EXPERIMENTAL
Materials
The polycrystalline Te-excess BST samples were synthesized using the conventional melting method. The stoichiometric amounts of Bi (99.999%), Sb (99.999%), and Te (99.999%) were sealed in evacuated quartz tubes under high vacuum with the excess tellurium (~7 W%)[9]. The elements in the quartz ampule were melted at 923 K for 24 h and then water-quenched. The obtained ingots were pulverized into the powder using an agate mortar and pestle under an argon atmosphere. The TaNb2HfZrTi HEA powders were synthesized by the planetary ball milling method using powder elements. The stoichiometric ratios of Ta (99.98%), Nb (99.8%), Hf (99.6%), Zr (99.5%), and Ti (99.5%) powders were loaded into a stainless-steel jar with stainless-steel balls under an argon atmosphere. The ball milling process was carried out at a rotation speed of 400 rpm for 12 h[24].
The obtained BST and HEA (0, 0.1, 0.5 and 1.0 vol%) powders were mixed using a physical multimixer in vibration mode for 24 h. The mixed powders were loaded into a graphite die with an inner diameter of
Measurements
Since the BST exhibits anisotropic characteristics due to its layer structure, the thermoelectric properties and X-ray diffraction (XRD) patterns of the sintered samples were measured along the Pa and perpendicular (Pe) directions to the applied pressure direction of the hot press. The XRD patterns for the Pa- and Pe-directions were obtained using the Cu-κα radiation (D8 advance, Bruker, Germany).
The temperature-dependent electrical resistivities (ρ = 1/σ) and S were simultaneously measured under a helium atmosphere using a thermoelectric properties measurement system (ZEM-3, ULVAC-RIKO, Japan). The Hall carrier concentrations (nH) were obtained by the relations of nH = -1/(RHe), where RH (= ρxy/H) is the Hall coefficient, e is the elementary charge, ρxy is the Hall resistivity, and H is the applied magnetic field. The ρxy was measured by the fore-probe contact method under sweeping magnetic fields from -1 T to 1 T using a physical property measurement system (PPMS Dynacool 14 T, Quantum Design, USA). The κtotal was obtained using κ = ρsλCp, where ρs, λ, and Cp are sample density, thermal diffusivity, and specific heat, respectively. The λ was measured using a laser flash method (LFA-457, NETZSCH, Germany). The densities of the sintered samples were determined from their mass and dimensions. The Cp was measured using differential scanning calorimetry (DSC, NEXTA DSC200, HITACHI, Japan).
RESULTS AND DISCUSSION
Figure 1 shows the XRD patterns of the hot-pressedBST+HEAx (x = 0, 0.1, 0.5, and 1.0 vol%) samples for the Pa and Pe -directions. The XRD peaks of the BST+HEAx samples are normalized to the highest (015) peaks to compare the intensity of the (00l) peaks. The XRD patterns of the BST+HEAx samples exhibit a rhombohedral structure with the lattice parameters (a ~4.296 Å, c ~30.496 Å), without any obvious impurities and significant changes in lattice parameters due to the HEA additions. The XRD peaks of the HEA powder show the BCC structure with the lattice parameter (a ~3.37 Å). The XRD patterns and the lattice parameters of the BST and HEA powder are consistent with previously reported results in the literature.[9,11,12,24,37] The intensities of the (00l) peaks for the Pa-direction are higher than those of the
Figure 1. XRD patterns of the BST+HEAx (x = 0, 0.1, 0.5, and 1.0 vol%) for the Pa- and Pe-directions, the ball-milled TaNb2HfZrTi high entropy alloy (HEA) powder and the calculated data. XRD: X-ray diffraction.
Figure 2A and B shows the HR-TEM image of the HEA nanoparticles and the BST matrix in the x =
Figure 2. (A) High-resolution transmission electron microscopy (HR-TEM) image of the HEA nanoparticles and BST matrix in the BST+HEAx (x = 0.1 vol%) sample; (B) the lattice sparing corresponding to the (009) plane of the BST structure; (C) the TEM image of the x = 0.1 vol% sample with the elemental mapping of bismuth (Bi), antimony (Sb), tellurium (Te), tantalum (Ta), niobium (Nb), hafnium (Hf), zirconium (Zr), and titanium (Ti). HEA: High entropy alloy; BST: Bi0.4Sb1.6Te3.
Figure 3A presents the temperature-dependent electrical conductivities [σ(T)] of the hot-pressed BST+HEAx samples for the Pa- and Pe-directions. The obtained σ(T) values exhibit highly degenerate semiconducting behavior for the Pa- and Pe-directions. The σ of the pristine BST (550 S cm-1 for the
Figure 3. (A) Temperature-dependent electrical conductivities σ(T); (B) Seebeck coefficients S(T); (C) temperature-dependent power factors S2σ(T) of the BST+HEAx (x = 0, 0.1, 0.5, and 1.0 vol%) samples for the Pa- and Pe-directions; (D) room-temperature electrical conductivities and Seebeck coefficients as a function of the HEA concentrations (error bar: 5%). BST:
The temperature-dependent Seebeck coefficients [S(T)] of the hot-pressed BST+HEAx (x = 0, 0.1, 0.5, and 1.0 vol%) samples for the Pa-direction and Pe-direction are presented in Figure 3B. The S(T) values show maxima due to the bipolar effect caused by the narrow bandgap (~0.1 eV) of the bismuth tellurides[40]. The S values of the pristine BST (224 for the Pa-direction, 216 μV K-1 for the Pe-direction at room temperature) are comparable with the literature values (235~245 μV K-1 for the Pa-direction, 215~230 μV K-1 for the
The temperature-dependent power factors [S2σ(T)] of the BST+HEAx samples for the Pa- and Pe-directions are presented in Figure 3C. The S2σ(T) values of the Pe-direction are higher than those of the Pa-direction due to the higher electrical conductivity resulting from the preferred orientations of the BST layers. The S2σ of the pristine BST (2.7 mW m-1 K-2 for the Pa-direction, 3.6 mW m-1 K-2 for the Pe-direction at room temperature) are comparable to the literature values (3.0~3.6 mW m-1 K-2 for the Pa-direction,
The enhanced S2σ by the additions of the HEA nanoparticles are primarily attributed to the increased σ, as shown in Figure 3D. The S of the BST+HEAx samples do not show significant changes (within a 5% error) by the additions of the HEA below 1.0 vol%. However, the σ values of the BST+HEAx samples are significantly affected by the HEA additions. Notably, the highest σ is observed in the low HEA concentration sample (x = 0.1 vol%), and the σ values for the Pa-direction are significantly enhanced compared to those for the Pe-direction with the HEA additions. The enhanced σ for the Pa-direction clearly indicates that the metallic HEA nanoparticles effectively increase the electrical conductivity in the
The different parts of the BST+HEAx (x = 0.1 vol%) were measured for the Pa-direction as shown in Supplementary Figure 2. The comparable σ(T), S(T) and S2σ(T) of the Pa-1 and Pa-2 of the BST+HEAx
Figure 4A shows that the nH of the hot-pressed BST+HEAx (x = 0, 0.1, 0.5, and 1.0 vol%) samples are slightly enhanced at the 0.1 vol% sample, but are not significantly affected in the range from 0.5 vol% to 1.0 vol%. The trends in the nH values of the BST with the HEA additions are notably different compared to those with other doping elements such as Ni, Sn[41], Pb, Fe, Co[37] and Ag[10], as shown in Figure 4B. The less susceptible nH values of the BST+HEAx samples suggest that the HEA nanoparticles exist in the BST matrix without significant chemical reactions that would alter the carrier concentrations. This behavior is attributed to the structural stability of the HEA nanoparticles[20]. The stable structural phase of the HEA nanoparticles may promote the formation of an ideal composite with BST, without inducing chemical doping effects. Further detailed investigations are required to understand the unchanged carrier concentrations in the BST+HEA composite.
Figure 4. (A) Room-temperature Hall carrier concentrations nH as a function of the HEA concentrations; (B) carrier concentrations nH for the various doping elements: Ni, Sn[41], Pb, Fe, Co[37], Ag[10]; (C) room-temperature Hall mobilities μH as a function of the HEA concentrations; (D) mobilities μH for the various doping elements: Ni, Sn[41], Pb, Fe, Co[37], Ag[10]. HEA: High entropy alloy.
Using σ = neμ, where n, e, and μ are the carrier concentration, elementary charge, and carrier mobility, respectively[2,5], the Hall mobilities (μH) of the BST+HEAx samples were investigated. Figure 4C shows that the enhancements in the μH are observed in the Pa-direction at low HEA concentrations, in contrast to the less susceptible μH of the Pe-direction. Although the μH is enhanced to the Pa-direction, the μH values of the BST with the HEA additions are not significantly changed compared to those with other doping elements such as Ni, Sn[41], Pb, Fe, Co[37] and Ag[10], as shown Figure 4D.
Figure 5A presents the effective masses of carrier m* of the hot-pressed BST+HEAx samples, obtained by the Pisarenko relation[2,5]. Although the S change of the BST+HEAx is insignificant, the m* is slightly enhanced at the lower HEA concentration (x = 0.1 vol%). Still, it has slightly decreased at the higher HEA concentrations (x = 0.5 and 1.0 vol%). The enhanced m* at the lower HEA concentration (x = 0.1 vol%) can be attributed to the resonant state effect[2,5]. However, further detailed investigations are needed to clarify these effects.
Figure 5. (A) Seebeck coefficients as a function of nH with the Pisarenko plots of the hot-pressed BST+HEAx (x = 0, 0.1, 0.5, and 1.0 vol%) samples for the Pa- and Pe-directions; (B) Fermi energies EF and the mean free paths of the carrier λe; (C) room-temperature power factors S2σ; (D) electrical grain connectivity Gconn. as a function of the HEA concentrations at room temperature. HEA: High entropy alloy; BST: Bi0.4Sb1.6Te3.
Using the S, Hall carrier mobilities μH, and effective masses of carrier m*, the mean free paths of carrier λe, and the EF of the hot-pressed BST+HEAx (x = 0, 0.1, 0.5, and 1.0 vol%) samples were calculated [Figure 5B] using
where r is the scattering factor (r = -1/2 for acoustic phonon scattering)[39,42]. The EF is not significantly affected by the additions of the HEA nanoparticles in the BST matrix, as presented in Table 1. In contrast to the less susceptible λe for the Pe-direction, the λe in the Pa-direction of the BST+HEA is increased compared to the pristine BST. The results of the λe clearly show that the HEA nanoparticle distribution is effective for enhancing the σ in the Pa-direction of the BST through the increased λe.
Mean free paths of the carrier λe, Fermi energies EF, the phonon mean free path λPh, and average phonon scattering time τph of the BST+HEAx samples
Sample | Ef (eV) | λ e (nm) | λ Ph (nm) | τ ph (s 10-12) |
Pa - 0 | 32.7 | 10.4 | 5.3 | 2.88 |
Pa - 0.1 | 33.1 | 12.6 | 4.9 | 2.88 |
Pa - 0.5 | 33.3 | 13.6 | 5.6 | 2.94 |
Pa - 1.0 | 33.3 | 12.3 | 6.1 | 2.95 |
Pe - 0 | 33.9 | 14.5 | 6.1 | 2.49 |
Pe - 0.1 | 33.4 | 14.4 | 5.8 | 2.16 |
Pe - 0.5 | 34.4 | 13.6 | 6.3 | 2.51 |
Pe - 1.0 | 33.7 | 14.7 | 6.6 | 3.08 |
The enhanced λe, without the changes in the nH and EF, can be attributed to the effect of the enhanced electrical grain connectivity[25,27,28]. Since the BST grains and the HEA nanoparticles are homogeneously mixed in the BST+HEA samples, the electrical resistivities of the BST+HEA are strongly affected by the metallic HEA nanoparticles.
The temperature-dependent scattering of the electrical resistivity can be roughly estimated by the characteristic change in the resistivity Δρ = ρ(Thigh) - ρ(Tlow)[25,27,28]. Using the temperature-dependent electrical resistivities ρ(T) of the BST+HEAx samples, the characteristic change in the resistivities Δρ was obtained, as shown in Supplementary Figure 3A and B. However, the Δρ does not provide a clear understanding of the electrical transport characteristics of the composites. To address this, a modified factor for the electrical grain connectivity Gconn. is introduced to quantify the contribution of the grain connectivity to the electrical conductivity,
where Δσ = σ(Tlow) - σ(Thigh) is the characteristic change in the electrical conductivity for the BST+HEA samples. In this work, the Tlow = 300 K and Thigh = 500 K are used for the σ(T) of the pristine BST and BST+HEA samples.
The tendencies of the S2σ of the BST+HEAx samples with the HEA additions for the Pa- and Pe-directions are shown in Figure 5C. The trends in the S2σ align well with the Gconn. as a function of the HEA concentrations for the Pa- and Pe-directions, as shown in Figure 5D. The Pa-direction Gconn. is enhanced by the HEA nanoparticle, whereas the Pe-direction Gconn. is not significantly affected. These trends are consistent with the results for the λe. The enhanced Gconn and λe clearly indicate that the HEA nanoparticles are more effective in increasing the electrical grain connectivity of BST in the Pa-direction, which originally exhibits lower electrical conductivity compared to the Pe-direction. Therefore, the power factor can be enhanced through increased electrical conductivity without a change in carrier concentration due to the improved Gconn. induced by the addition of HEA nanoparticles in the BST matrix.
The temperature-dependent κtotal [κtotal(T)] of the hot-pressed BST+HEAx (x = 0, 0.1, 0.5, and 1.0 vol%) samples for the Pa- and Pe-directions is shown in Figure 6A. The thermal conductivity of the sintered HEA (TaNb2HfZrTi) sample is κ = 10.25 W m-1K-1 at 300 K. The κtotal(T) of the BST+HEAx samples decreases with increasing temperature near room temperature. The κtotal(T) is increased with an increasing temperature above 350 K due to the bipolar effect, consistent with the previous reports[9,11]. The lower Pa-direction
Figure 6. (A) Temperature-dependent total thermal conductivities κtotal(T); (B) temperature-dependent Lorenz numbers L(T); (C) temperature-dependent lattice and bipolar thermal conductivities κL + κb(T) of the hot-pressed BST+HEAx (x = 0, 0.1, 0.5, and 1.0 vol%) samples for Pa- and Pe-directions; (D) room-temperature lattice thermal conductivities κL as a function of the HEA concentrations. HEA: High entropy alloy; BST: Bi0.4Sb1.6Te3.
The κtotal of the bismuth telluride is primarily affected by the phonons κL, electrons κe, and bipolar diffusion
where S is the temperature-dependent Seebeck coefficient, Fn(η) is the n-th order Fermi integral, r is scattering parameter (r = -1/2 for the acoustic phonon scattering), and η = EF/kBT is the reduced EF[38,39].
By subtracting the κe from κtotal, the temperature-dependent lattice and bipolar thermal conductivities
Figure 6D shows the lattice thermal conductivities κL of the BST+HEAx samples for the Pa- and
The lattice thermal conductivities κL can be described by the Debye-Callaway model using
where Θ is the Debye temperature, τC is the total scattering time rate, and ν is the sound velocity. The scattering time τC, which is associated with boundary scattering, point defect scattering, and Umklapp scattering, can be expressed as follows
where L is the average diameter of grain boundary, ω is phonon frequency, V is the lattice volume, Γ is the scattering parameter of a point defect, AN is the free parameter of Umklapp scattering, γ is the Grüneisen parameter, and M is the average atomic mass.[43]
The thermal conductivity due to the bipolar contribution can be expressed as follows
where B is a fitting parameter[44].
Based on the theoretical equations for the κL and κb along with the parameters (in Supplementary Table 1) such as the average group velocity of a phonon (ν = 2147 /s), acoustic Debye temperature (Θ = 94 K),
From the Debye-Callaway model and the obtained fitting parameters, the λPh and average phonon scattering time τph can be obtained using
The obtained λPh and τph are listed in Table 1. The λPh follows the behavior of κL with HEA concentrations as presented in Table 1 and Figure 6D. The λPh values decreased for x = 0.1 and monotonically increased with increasing the HEA concentration for both the Pa- and Pe-directions of the BST+HEAx samples. The decreasing λPh for x = 0.1 is due to the phonons’ scattering between the BST matrix and nanoprecipitation of HEA. The increasing λPh is consistent with the grain connectivity increase between the HEA nanoparticles and BST matrix.
Temperature-dependent ZT values of the hot-pressed BST+HEAx (x = 0, 0.1, 0.5, and 1.0 vol%) samples for the Pa- and Pe-directions are presented in Figure 7A. The ZT values are increased with increasing temperature near room temperature but decrease above 350 K due to the bipolar effect. The ZT values of the BST+HEAx samples are enhanced with the additions of the HEA nanoparticles below 0.5 vol%. The ZT value of the sintered HEA (TaNb2HfZrTi) sample is ZT = 0.0012 at 300 K. Figure 7B clearly shows that the HEA additions in the BST matrix improve the TE performances below 0.5 vol%. In particular, the HEA nanoparticles are more effective at enhancing the ZT values of the BST in the Pa-direction. The ZT values in the BST with the HEA additions are increased from 0.93 (x = 0) to 1.23 (x = 0.1 vol%) for the Pa-direction at 300 K. Furthermore, the maximum ZT value (1.33 at 350 K) is observed in the 0.1 vol% sample for the
Figure 7. (A) Temperature-dependent figure of merit ZT of the hot-pressed BST+HEAx (x = 0, 0.1, 0.5, and 1.0 vol%) samples for the Pa- and Pe-direction; (B) ZT values as a function of the HEA concentrations at 300 and 350 K; (C) maximum ZTavg. of the BST+HEAx samples at the Thot = 425K and Tcold = 300 K; (D) average ZT with the literature values[37,41,45-51]. BST: Bi0.4Sb1.6Te3; HEA: high entropy alloy.
The HEA nanoparticles effectively increase the electronic MFP λe in the Pa-direction, and decrease the λPh in both Pe- and Pa-directions for x ≤ 0.5, indicating the effective phonon glass and electron crystal by enhancing the grain connectivity and nanoparticle distribution in the BST matrix. The enhanced ZT value of the Pa-direction in the 0.1 vol% sample is caused by the increased electrical conductivity and decreased
Figure 7C and D shows the average ZT (ZTavg.), which can be obtained by[3]
where Thot and Tcold are the temperatures on the hot side and cold side, respectively. The ZTavg. is an important factor for real applications when the thermoelectric materials are exposed to thermal gradients. The maximum ZTavg. of the BST+HEAx samples is 1.26 (0.1 vol%, Pa-direction) at the Thot = 425K and Tcold = 300 K. The ZTavg. of the BST+HEAx samples show higher TE performance compared to other reported values in the literature[37,41,45-51]. The high ZTavg. values achieved with the HEA additions in the BST indicate strong potential for practical applications under temperature gradients.
CONCLUSIONS
In summary, the anisotropic thermoelectric properties of the sintered BST+HEAx (x = 0, 0.1, 0.5, and
DECLARATIONS
Authors’ contributions
Investigation, data curation, formal analysis, methodology, writing - original draft: Kumar, A.
Investigation, data curation, formal analysis, writing - original draft: Thoravat, S.
Conceptualization, characterization, visualization, writing - review and editing: Yun, J. H.
Investigation, formal analysis: Park, J.; Jin, H.
Investigation, data curation, formal analysis: Hidayati, R.
Conceptualization, characterization, visualization, methodology, formal analysis, writing - original draft, writing - review and editing: Kim, J. H.
Conceptualization, funding acquisition, resources, supervision, writing - review and editing: Rhyee, J. S.
All authors analyzed the data and contributed to the discussions.
Availability of data and materials
Some results of supporting the study are presented in the Supplementary Materials. Other raw data that support the findings of this study are available from the corresponding author upon reasonable request.
Financial support and sponsorship
This research was supported by the National Research Foundation of Korea (NRF), funded by the Ministry of Education, Science and Technology (RS-2023-00247622 and NRF-2022M3C1A3091988).
Conflicts of interest
All authors declared that there are no conflicts of interest.
Ethical approval and consent to participate
Not applicable.
Consent for publication
Not applicable.
Copyright
© The Author(s) 2025.
Supplementary Materials
REFERENCES
1. Yan, Q.; Kanatzidis, M. G. High-performance thermoelectrics and challenges for practical devices. Nat. Mater. 2022, 21, 503-13.
2. Shi, X. L.; Zou, J.; Chen, Z. G. Advanced thermoelectric design: from materials and structures to devices. Chem. Rev. 2020, 120, 7399-515.
3. Hameed, M. M.; Mansor, M.; Azrin, Mohd., Azau., M.; Muhsin, S. Thermoelectric cooler performance enhancement using thermoelectric generators and their use as a single model to improve the performance of thermal battery management systems for electric vehicles. Energy. Storage. 2023, 5, e406.
5. Wu, Z.; Zhang, S.; Liu, Z.; Mu, E.; Hu, Z. Thermoelectric converter: strategies from materials to device application. Nano. Energy. 2022, 91, 106692.
6. Hao, F.; Qiu, P.; Tang, Y.; et al. High efficiency Bi2Te3-based materials and devices for thermoelectric power generation between 100 and 300 °C. Energy. Environ. Sci. 2016, 9, 3120-7.
7. Witting, I. T.; Chasapis, T. C.; Ricci, F.; et al. The thermoelectric properties of bismuth telluride. Adv.. Electron. Mater. 2019, 5, 1800904.
8. Hu, L.; Zhu, T.; Liu, X.; Zhao, X. Point defect engineering of high-performance bismuth-telluride-based thermoelectric materials. Adv. Funct. Mater. 2014, 24, 5211-8.
9. Kim, Y. M.; Lydia, R.; Kim, J.; Lin, C.; Ahn, K.; Rhyee, J. Enhancement of thermoelectric properties in liquid-phase sintered Te-excess bismuth antimony tellurides prepared by hot-press sintering. Acta. Mater. 2017, 135, 297-303.
10. Zhu, B.; Xie, W.; Huang, R.; et al. High thermoelectric performance in Ag-doped Bi0.5Sb1.5Te3 nanocomposites synthesized via low-temperature liquid phase sintering. Mater. Today. Energy. 2024, 46, 101717.
11. Deng, R.; Su, X.; Zheng, Z.; et al. Thermal conductivity in Bi0.5Sb1.5Te3+x and the role of dense dislocation arrays at grain boundaries. Sci. Adv. 2018, 4, eaar5606.
12. Cho, H.; Kim, J. H.; Back, S. Y.; Ahn, K.; Rhyee, J.; Park, S. Enhancement of thermoelectric properties in CuI-doped Bi2Te2.7Se0.3 by hot-deformation. J. Alloys. Compd. 2018, 731, 531-6.
13. Haruna, A. Y.; Luo, Y.; Li, W.; et al. High thermoelectric performance in multiscale Ag8SnSe6 included n-type bismuth telluride for cooling application. Mater. Today. Energy. 2023, 35, 101332.
14. Luo, K.; Chen, H.; Hu, W.; et al. Tailoring interfacial states for improved n-type bismuth telluride thermoelectrics. Nano. Energy. 2024, 128, 109845.
15. Beekman, M.; Morelli, D. T.; Nolas, G. S. Better thermoelectrics through glass-like crystals. Nat. Mater. 2015, 14, 1182-5.
16. Wang, X.; Guo, W.; Fu, Y. High-entropy alloys: emerging materials for advanced functional applications. J. Mater. Chem. A. 2021, 9, 663-701.
17. Ghosh, S.; Raman, L.; Sridar, S.; Li, W. High-entropy engineering in thermoelectric materials: a review. Crystals 2024, 14, 432.
18. Ye, Y.; Wang, Q.; Lu, J.; Liu, C.; Yang, Y. High-entropy alloy: challenges and prospects. Mater. Today. 2016, 19, 349-62.
20. Yao, Y.; Dong, Q.; Brozena, A.; et al. High-entropy nanoparticles: synthesis-structure-property relationships and data-driven discovery. Science 2022, 376, eabn3103.
21. Jiang, B.; Yu, Y.; Cui, J.; et al. High-entropy-stabilized chalcogenides with high thermoelectric performance. Science 2021, 371, 830-4.
22. Jiang, B.; Yu, Y.; Chen, H.; et al. Entropy engineering promotes thermoelectric performance in p-type chalcogenides. Nat. Commun. 2021, 12, 3234.
23. Jiang, B.; Wang, W.; Liu, S.; et al. High figure-of-merit and power generation in high-entropy GeTe-based thermoelectrics. Science 2022, 377, 208-13.
24. Kim, J. H.; Hidayati, R.; Jung, S.; et al. Enhancement of critical current density and strong vortex pinning in high entropy alloy superconductor Ta1/6Nb2/6Hf1/6Zr1/6Ti1/6 synthesized by spark plasma sintering. Acta. Materialia. 2022, 232, 117971.
25. Martínez, E.; Mikheenko, P.; Martínez-lópez, M.; Millán, A.; Bevan, A.; Abell, J. S. Flux pinning force in bulk MgB2 with variable grain size. Phys. Rev. B. 2007, 75.
26. Abou, El., Hassan., A.; Labrag, A.; Taoufik, A.; et al. Magnetic penetration depth and coherence length in a single-crystal YBa2Cu3O7-δ. Physica. Status. Solidi. (b). 2021, 258, 2100292.
27. Rowell, J. M. The widely variable resistivity of MgB2 samples. Supercond. Sci. Technol. 2003, 16, R17-27.
28. Jiang, J.; Senkowicz, B. J.; Larbalestier, D. C.; Hellstrom, E. E. Influence of boron powder purification on the connectivity of bulk MgB2. Supercond. Sci. Technol. 2006, 19, L33-6.
29. Muhammad, Y.; Rahim, M.; Hussain, N.; Iqbal, Z.; Naseem, A. Enhanced transport properties of (Ag)x/CuTl-1223 nano-composites with the application of high pelletization pressure. Appl. Phys. A. 2024, 130, 7801.
30. Matthews, G. A. B.; Mousavi, T.; Santra, S.; Grovenor, C. R. M.; Grant, P. S.; Speller, S. Improving the connectivity of MgB2 bulk superconductors by a novel liquid phase sintering process. Supercond. Sci. Technol. 2022, 35, 065005.
31. Xu, Z.; Jiang, Z.; Kuai, C.; et al. Charge distribution guided by grain crystallographic orientations in polycrystalline battery materials. Nat. Commun. 2020, 11, 83.
32. Wang, Q.; Zhao, C.; Hu, X.; et al. Grain-boundary-rich interphases for rechargeable batteries. J. Am. Chem. Soc. 2024, 146, 31778-87.
33. Son, D.; Lee, J.; Choi, Y. J.; et al. Self-formed grain boundary healing layer for highly efficient CH3NH3PbI3 perovskite solar cells. Nat. Energy. 2016, 1, 16081.
34. Sherkar, T. S.; Momblona, C.; Gil-Escrig, L.; et al. Recombination in perovskite solar cells: significance of grain boundaries, interface traps, and defect ions. ACS. Energy. Lett. 2017, 2, 1214-22.
35. Choi, H. H.; Paterson, A. F.; Fusella, M. A.; et al. Hall effect in polycrystalline organic semiconductors: the effect of grain boundaries. Adv. Funct. Mater. 2020, 30, 1903617.
36. Sarkar, P.; Muhammed, Ali., A. V.; Ghorai, G.; et al. On the grain boundary charge transport in p-type polycrystalline nanoribbon transistors. Nanoscale 2024, 16, 16611-21.
37. Wei, Z.; Wang, C.; Zhang, J.; et al. Precise regulation of carrier concentration in thermoelectric BiSbTe alloys via magnetic doping. ACS. Appl. Mater. Interfaces. 2020, 12, 20653-63.
38. Kim, J. H.; Cho, H.; Back, S. Y.; Yun, J. H.; Lee, H. S.; Rhyee, J. Lattice distortion and anisotropic thermoelectric properties in hot-deformed CuI-doped Bi2Te2·7Se0.3. J. Alloys. . Compd. 2020, 815, 152649.
39. Kim, J. H.; Back, S. Y.; Yun, J. H.; Lee, H. S.; Rhyee, J. S. Scattering mechanisms and suppression of bipolar diffusion effect in Bi2Te2.85Se0.15Ix compounds. Materials 2021, 14, 1564.
40. Cao, T.; Shi, X.; Li, M.; et al. Advances in bismuth-telluride-based thermoelectric devices: progress and challenges. eScience 2023, 3, 100122.
41. Hu, X.; Xiang, Q.; Kong, D.; et al. The effect of Ni/Sn doping on the thermoelectric properties of BiSbTe polycrystalline bulks. J. Solid. State. Chem. 2019, 277, 175-81.
42. Zhu, T.; Gao, H.; Chen, Y.; Zhao, X. Ioffe-regel limit and lattice thermal conductivity reduction of high performance (AgSbTe2)15(GeTe)85 thermoelectric materials. J. Mater. Chem. A. 2014, 2, 3251-6.
43. Kim, H.; Kim, S. I.; Lee, K. H.; Kim, S. W.; Snyder, G. J. Phonon scattering by dislocations at grain boundaries in polycrystalline Bi0.5Sb1.5Te3. Physica. Status. Solidi. (b). 2017, 254, 1600103.
44. Bahk, J.; Shakouri, A. Minority carrier blocking to enhance the thermoelectric figure of merit in narrow-band-gap semiconductors. Phys. Rev. B. 2016, 93, 165209.
45. Wei, Z.; Yang, Y.; Wang, C.; Li, Z.; Zheng, L.; Luo, J. Enhanced room-temperature thermoelectric performance of p-type BiSbTe by reducing carrier concentration. RSC. Adv. 2019, 9, 2252-7.
46. Cao, S.; Huang, Z. Y.; Zu, F. Q.; Xu, J.; Yang, L.; Chen, Z. G. Enhanced thermoelectric properties of Ag-modified Bi0.5Sb1.5Te3 composites by a facile electroless plating method. ACS. Appl. Mater. Interfaces. 2017, 9, 36478-82.
47. Dharmaiah, P.; Lee, K.; Song, S. H.; Kim, H. S.; Hong, S. Enhanced thermoelectric performance of Bi0.5Sb1.5Te3 composites through potential barrier scattering at heterogeneous interfaces. Mater. Res. Bull. 2021, 133, 111023.
48. Wu, G.; Yan, Z.; Wang, X.; et al. Optimized thermoelectric properties of Bi0.48Sb1.52Te3 through AgCuTe doping for low-grade heat harvesting. ACS. Appl. Mater. Interfaces. 2021, 13, 57514-20.
49. Li, Y.; Ren, M.; Sun, Z.; Yao, Z. Nanoarchitectonics of p-type BiSbTe with improved figure of merit via introducing PbTe nanoparticles. RSC. Adv. 2021, 11, 36636-43.
50. Maksymuk, M.; Dzundza, B.; Matkivsky, O.; Horichok, I.; Shneck, R.; Dashevsky, Z. Development of the high performance thermoelectric unicouple based on Bi2Te3 compounds. J. Power. Sources. 2022, 530, 231301.
Cite This Article

How to Cite
Download Citation
Export Citation File:
Type of Import
Tips on Downloading Citation
Citation Manager File Format
Type of Import
Direct Import: When the Direct Import option is selected (the default state), a dialogue box will give you the option to Save or Open the downloaded citation data. Choosing Open will either launch your citation manager or give you a choice of applications with which to use the metadata. The Save option saves the file locally for later use.
Indirect Import: When the Indirect Import option is selected, the metadata is displayed and may be copied and pasted as needed.
About This Article
Special Issue
Copyright
Data & Comments
Data
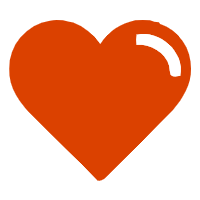
Comments
Comments must be written in English. Spam, offensive content, impersonation, and private information will not be permitted. If any comment is reported and identified as inappropriate content by OAE staff, the comment will be removed without notice. If you have any queries or need any help, please contact us at [email protected].