High-valence molybdenum-induced boundary-rich heterostructures for enhanced oxygen evolution reaction
Abstract
The green synthesis of hydrogen through electrochemical water splitting has been severely limited by the slow kinetics of the anodic oxygen evolution reaction (OER). However, the current benchmark electrocatalysts are still based on precious metals. Therefore, developing low-cost and highly efficient OER electrocatalysts is of great importance. Here, we design nanoscale multicomponent metal flakes with a crystalline/amorphous structure
Keywords
INTRODUCTION
Green hydrogen has been extensively studied as a renewable energy to replace fossil fuels[1-4]. Electrochemical water splitting is one of the most efficient strategies to enable the production of hydrogen with zero CO2 emission[5-7]. However, the slow kinetics of the oxygen evolution reaction (OER) at the anode side of water splitting severely hinders its further commercialization[8,9]. Currently, precious metal electrocatalysts based on ruthenium (Ru) and iridium (Ir) are still the most widely used and active OER electrocatalysts[10]. Nevertheless, their low abundance and high cost highly impede their further application. Alternatively, transition metal-based catalysts, e.g., iron/cobalt/nickel (Fe/Co/Ni), have exhibited promising OER activity and stability in alkaline conditions, with the advantage of abundant distribution and low-cost properties[11,12]. The intrinsic structures of transition metal compounds are suitable for OER, but their catalytic activity is still far from satisfactory[12,13]. Therefore, developing novel strategies to engineer
One strategy is to incorporate foreign elements to boost the OER catalytic performance by modulating the electronic structure of Fe/Ni/Co species and facilitating electron transportation[14,15]. Especially, the doped high valence state elements can induce strong electronic interaction between high valence state metal atoms and Fe/Co/Ni species, which can significantly modulate the corresponding properties to further effectively promote the pre-activation process of Fe/Co/Ni-based catalysts to optimize their OER performance. Recently, the high valence element Mo has been investigated as the doping agent to engineer Fe, Co or
Besides doping strategy, amorphization and defects in metal-based materials can also influence the electronic structure to engineer the active sites, and thus improve the OER performance[20,21]. However, excessive defect sites may result in poor conductivity and decrease the OER performance. Thus, alloying amorphous oxides with crystalline oxides forming crystalline/amorphous heterostructures may have excellent OER electrocatalytic activity due to their combined advantages[22]. For example, the crystalline FeOOH combined with amorphous FeOOH phase boundaries (a-c-FeOOH) has shown superior OER activity compared to that of amorphous FeOOH or crystalline FeOOH (a-c-FeOOH:300 mA cm-2@330 mV, a-FeOOH:47 mA cm-2@330 mV, c-FeOOH: 60 mA cm-2@330 mV)[23]. Moreover, Fe-doped Ni-Co photosensitive electrocatalyst (Fe-NiCoHPi) with the amorphous shell and crystal core has increased the active sites and conductivity, respectively[24]. As a result, Fe-NiCoHPi delivered current densities of
Herein, we successfully applied sol-gel and annealing strategies to prepare the nanoscale polymetallic crystalline/amorphous coexisting FeCoNiMo flasks with abundant boundary structures (ac-FeCoNiMo) induced by high valence state of the Mo element. It also engineers the electronic structure of Fe/Co and achieves decreased valence states due to the electron transfer between Fe/Co and Mo. Therefore, the resulting ac-FeCoNiMo has exhibited superior OER electrocatalytic activity compared to benchmark RuO2, with a low overpotential of 208 mV at the current density of 10 mA cm-2, and a Tafel slope of
EXPERIMENTAL
Materials
Chemicals including ethanol (C2H6O, A.R.) and acetone (C3H6O, A.R.) were purchased from China National Pharmaceutical Group Chemical Reagent Co., Ltd and used as received. Chemicals including iron chloride (FeCl3, A.R.), nickel chloride (NiCl2, A.R.), cobalt chloride (CoCl2, A.R.) and Nafion®117 (5 wt.%) were purchased from Sigma-Aldrich and used as received. Molybdenum (MoCl5, A.R.) and epoxy propane (C3H6O, A.R.) were purchased from Shanghai Titan Chemical Co., Ltd and used as received.
Synthesis of ac-FeCoNiMo
As shown in Supplementary Figure 1, ac-FeCoNiMo was prepared according to the reported methods[1,2]. Briefly, 0.2 mL of deionized water, 2 mL of ethanol and 1 mL of epoxy propane were added to a solution mixed with 0.07 mmol of anhydrous iron chloride, 0.07 mmol of anhydrous cobalt chloride, 0.07 mmol of anhydrous nickel chloride, and 0.07 mmol of anhydrous molybdenum chloride and 4 mL of anhydrous ethanol. The mixed solution was then stirred overnight and aged in acetone for one week to obtain the
Synthesis of the comparison materials
The synthesis procedures of the controls were the same as those of ac-FeCoNiMo, without the addition of anhydrous molybdenum chloride for FeCoNi, anhydrous nickel chloride for FeCoMo, and anhydrous cobalt chloride for FeNiMo, respectively.
Characterization
The material morphology details and structural information were obtained using a Transmission Electron Microscope (TEM, JEOL 2100F) and X-ray Diffraction (XRD, 3 KW D/MAX2200V, step size of 0.02°, scanning rate of 8° min-1), and the sample elemental content and distribution were studied by Energy Dispersive X-ray spectroscopy (EDX) combined with TEM and Aglient 7800 Inductively coupled
Electrocatalytic measurements
For electrode preparation, 500 μL of ethanol, 10 μL of Nafion and 2 mg of catalyst were used to prepare electrode slurry, then dripped onto carbon paper to obtain the working electrode with the catalyst load of
Using the Nernst equation to convert the EHg/HgO/OH to ERHE:
LSV test was measured in 1 M KOH at the scan rate of 10 mV s-1 over the range of 0.2 V to 0.9 V versus RHE. Here, the CV test was used for the stability test with the range of 0.2 V to 0.6 V versus RHE at the sweep rate of 100 mV s-1 for 10,000 cycles. Through the LSV before and after the CV, its stability was evaluated.
The turnover frequency (TOF) is a key metric to evaluate the intrinsic catalytic activity of an OER catalyst, which can be calculated using
where the factor 4 accounts for the four-electron process involved in O2 evolution from water, I is the current (A) at corresponding overpotential from LSV curves, F is Faraday constant (96,485 C mol-1), and n is number of active sites. We assume that all metal sites are active sites for OER, and we can derive
Where Sg is the specific surface area value. N represents the number of surface atoms, m is the mass of the load per square centimeter, and NA represents Avogadro’s constant.
The long stability test was conducted in a three-electrode system using a constant-current mode. The working electrode, counter electrode, and reference electrode were the same as those in the LSV polarization curve experiment.
RESULTS AND DISCUSSION
Structural analysis of materials
The ac-FeCoNiMo was prepared by a sol-gel method which relies on controlling the hydrolysis of metal chlorides in the mixture of water and ethanol [Supplementary Figure 1]. Epoxy propane was added as a complexing agent and a gel initiator to coordinate with metal ion hydrates while promoting gelation via subsequent metal salt hydrolysis and polycondensation[26]. As a result, the color of the mixture gradually turned from clear to black, and eventually brownish green. Followed by a subsequent calcination treatment, the nanoscale multicomponent metal flakes with crystalline/amorphous structure (ac-FeCoNiMo) were achieved. The ICP-MS analysis confirmed the elemental compositions of Fe, Co, Ni, and Mo are 16.16 wt.%, 16.41 wt.%, 14.61 wt.%, and 27.72 wt.%. FeCoNi with no Mo incorporation has also been prepared using the same method as a comparison.
The XRD was further performed on ac-FeCoNiMo and FeCoNi to investigate the crystal structure. The results in Figure 1A show the peaks of 31.1°, 36.7°, 44.6°, 59.1°, and 64.9° in FeCoNi correspond to the crystal planes of FeCoNi oxide. However, no sharp characteristic peaks are observed for FeCoNiMo, suggesting much lower crystallinity. Therefore, the introduction of high valence Mo element can efficiently cause the decreased crystallinity of FeCoNi, which may contribute to the catalytic activity.
Figure 1. (A) XRD spectra of ac-FeCoNiMo and FeCoNi (B and C) TEM images of ac-FeCoNiMo, with the red dashed frame showing the amorphous structure region; (D-G) elemental mappings of ac-FeCoNiMo. XRD: X-ray diffraction; TEM: transmission electron microscope.
The as-synthesized ac-FeCoNiMo is further characterized by TEM. The TEM images in Figure 1B and C and Supplementary Figure 2 confirm that the achieved FeCoNiMo nanosheets contain both crystalline and amorphous structures (red box), which is consistent with the XRD result (lower crystallinity)[27]. The formed crystalline and amorphous structures result in the formation of abundant atomic boundaries and the atoms located at the boundary sites may display distorted coordinating states, to boost the OER activity[28,29]. In contrast, FeCoNi with no Mo incorporation shows a similar nanosheet nanostructure to that of
BET analysis was conducted on ac-FeCoNiMo and FeCoNi to characterize their specific surface area and pore size distribution. The nitrogen adsorption and desorption curve of ac-FeCoNiMo in Figure 2A is the typical Type II isotherm curve, which is different from the Type IV isotherm curve of FeCoNi. In addition, ac-FeCoNiMo exhibits much higher surface area and much smaller pore size distribution than FeCoNi
Figure 2. (A) Nitrogen desorption curve and pore distribution diagram of ac-FeCoNiMo and FeCoNi; (B-F) The high-resolution of Co 2p XPS spectra, Fe 2p XPS spectra, Ni 2p XPS spectra, Mo 3d XPS spectra and O 1s XPS spectra of ac-FeCoNiMo and FeCoNi. XPS: X-ray photoelectron spectroscopy.
The survey X-ray Photoelectron Spectroscopy (XPS) spectrum of ac-FeCoNiMo verifies the presence of Fe, Ni, Co, Mo, and O elements, which is consistent with the results of ICP-MS and Energy Dispersive X-ray Spectroscopy (EDS) [Figure 2, Supplementary Tables 2 and 3]. The high-resolution Co 2p spectra of both ac-FeCoNiMo and FeCoNi [Figure 2B] show two pairs of peaks corresponding to Co2+ and Co3+. These peaks shift negatively compared to those of FeCoNi (781.13 eV, 783.05 eV)[36]. Moreover, the ratio of Co3+ to Co2+ in FeCoNi and ac-FeCoNiMo obviously decreased from 1.37 to 0.59. Similarly, a decreased trend in the Fe2+/Fe3+ is observed in ac-FeCoNiMo (1.71 to 1.23) [Figure 2C][37,38]. Therefore, the incorporation of
For comparison, FeNiMo and FeCoMo were also prepared to investigate the multi-elements induced synergistic effect. The XRD results in Supplementary Figure 6 show that both FeNiMo and FeCoMo possess the same broadened and weakened XRD peak shape, indicating the presence of Mo may play an important role in the formation of low crystallinity structure.
To further elucidate the electronic structure, chemical state, and coordination environment of the synthesized ac-FeCoNiMo at the atomic level, X-ray absorption near-edge structure (XANES) and extended X-ray absorption fine structure (EXAFS) analyses were conducted on ac-FeCoNiMo. As depicted in Figure 3A, the K-edge absorption energies of the Fe for both ac-FeCoNiMo and FeCoNi are situated between the Fe foil and metal oxide, indicating that the valence state of Fe lies between 0 and +3. Moreover, the ac-FeCoNiMo shows a left position compared to FeCoNi, indicating a lower valence state of Fe species in ac-FeCoNiMo, which is consistent with the XPS results. Similarly, the lower valence state of Co species has also been observed for ac-FeCoNiMo [Figure 3B]. The XANES results further confirm the incorporation of high valence Mo induce the decreased valence state of Fe and Co species in ac-FeCoNiMo, suggesting electron transfer from the Mo element to the transition metals Fe and Co. The Fourier transforms of the EXAFS spectra (R space) of both Fe and Co species in Figure 3C and D show the dominant peak between 1-2 Å corresponding to the M-O (Fe/Co-O) coordination shell. Moreover, these peaks for Fe and Co all show obvious right peak shifts compared to the standard metal oxides (Fe2O3 and Co3O4), indicating the elongation of the M-O (Fe/Co) coordination bonds. The ac-FeCoNiMo with the incorporation of high valence Mo exhibits even enlarged bonds, which may be attributed to the formation of boundary-rich crystalline/amorphous heterostructures and Fe/Co-O-Mo. The enlarged bond lengths may result in the weakened metal-oxygen bond and activate the OL to facilitate the OER process[43].
Electrocatalytic performance
The electrochemical performance was conducted using a three-electrode device in 1 M KOH solution at room temperature. As shown in Figure 4A and Supplementary Figure 7, ac-FeCoNiMo exhibits much lower overpotentials among all the samples with overpotentials of 222 mV and 253 mV to achieve the current densities of 20 mA cm-2 and 100 mA cm-2, respectively, whereas 256 mV and 290 mV for FeCoNi, 274 mV and 332 mV for FeNiMo, 239 mV and 283 mV for FeCoMo, and 252 mV for RuO2 at current densities of
Figure 4. LSV curves (A) and Tafel curves (B) for ac-FeCoNiMo, FeCoNi, and RuO2; (C) EIS diagram of ac-FeCoNiMo and FeCoNi (D) Coordinate diagram of OER electrocatalyst overpotential and Tafel slope; (E) The calculated TOF of ac-FeCoNiMo and FeCoNi at certain overpotentials; (F) LSV diagram of ac-FeCoNiMo before and after 10000 CV cycles of ac-FeCoNiMo and FeCoNi; (G) the long-term stability test of ac-FeCoNiMo. LSV: Linear sweep voltammetry; EIS: electrochemical impedance spectroscopy; OER: oxygen evolution reaction; TOF: turnover frequency.
TOF has been calculated to assess the intrinsic OER catalytic activity. As shown in Figure 4E, ac-FeCoNiMo exhibits the TOF of 0.0225 s-1 and 0.0375 s-1 at potentials of 1.47 V and 1.48 V, respectively, which is much higher than that of FeCoNi (0.012 s-1 and 0.0205 s-1), demonstrating the superior intrinsic OER catalytic activity of ac-FeCoNiMo[46,47].
We further performed long-term stability tests on ac-FeCoNiMo by continuously running LSV tests. A comparison of LSV curves of ac-FeCoNiMo before and after 10000 CV cycles at the current density of
The study of OER catalytic mechanism
The LOM involves non-coordinated proton-electron transfer steps at metal cation active sites and OL. This mechanism is characterized by the presence of specific reaction intermediates Figure 5A, which serve as a basis for our assessment of the catalytic mechanism[48,49]. The superior catalytic activity of ac-FeCoNiMo over FeCoNi suggests the formed boundaries, decreased Fe/Co species and weakened Fe/Co-O bonds play a vital role in the OER process, which may change the catalytic mechanism. We have done the electrochemical performance test with and without TMA+. A noticeable attenuation in the polarization curves is observed, suggesting the generation of *O22- intermediates during the catalytic process on
Figure 5. (A) LOM Mechanism (B)TMAOH Poisoning Experiment and (C) pH-Dependence Experiment of ac-FeCoNiMo; (D) In situ ATR-SEIRAS spectra at various applied potentials for ac-FeCoNiMo. LOM: Lattice oxygen mediated; ATR-SEIRAS: attenuated total reflection surface-enhanced infrared absorption spectroscopy; TMAOH: tetramethylammonium hydroxide.
CONCLUSIONS
In summary, we designed a high OER electrocatalyst ac-FeCoNiMo by creating a boundary-rich crystalline/amorphous heterostructure, modulated electronic structure of Fe/Co species and defects via introducing high valence metal Mo into a multicomponent transition metal matrix. Furthermore, ac-FeCoNiMo exhibited an increased specific surface area and optimized pore size distribution. As a result, ac-FeCoNiMo achieved low overpotentials of 222 mV and 253 mV at current densities of 20 mA cm-2 and 100 mA cm-2, respectively, while displaying excellent stability, which was superior to the benchmark RuO2. The mechanism investigation indicates ac-FeCoNiMo follows LOM mechanism during the OER process, which can be ascribed to the induced weakened metal-oxygen bonds with the enlarged M-O-M units and the formation of the abundant rich boundaries. This work opens a new venue for developing highly efficient multi-element electrocatalysts and potential high-entropy materials for OER.
DECLARATIONS
Acknowledgments
All authors appreciate the support from the “Joint International Laboratory on Environmental and Energy Frontier Materials” and “Innovation Research Team of High-Level Local Universities in Shanghai”.
Authors’ contributions
Contributed equally to this work: Li, L.; Wu, J.
Supervised the research: Liu, Hao.; Zhao, Y.; Zhang, J.
Designed the research: Li, L.; Wu, J.; Zhao, Y.
Conducted the various in situ measurements: Sun, L.
Participated in catalyst synthesis and characterization: Cao, X.; Pan, F.; Gao, L.
Analyzed the data: Zhao, Y.; Gao, H.
Co-wrote the paper: Wu, J.; Li, L.
Participated in the discussion: Li, D.; Zhang, Y.; Li, Q.
All authors revised the manuscript and have given approval to the final version of the manuscript.
Availability of data and materials
The data supporting the findings of this study are available within this Article and its Supplementary Materials. Further data are available from the corresponding authors upon request.
Financial support and sponsorship
This work was supported by the National Natural Science Foundation of China (22209103). Zhao, Y. and Li, Q. thank the UTS-HUST Key Technology Partner Seed Fund for their support. Zhang, Y. also thanks the S&T Program of Hebei for their support. Zhang, J. acknowledges support from the Australian Research Council (DE240100868). Liu, H. thanks CSIRO for support through the “International Hydrogen Research Collaboration Program-Research Fellowship”.
Conflicts of interest
Liu, H. is listed as an Associate Editor of the journal Energy Materials. However, Hao Liu was not involved in any aspect of the editorial process, including reviewer selection, manuscript handling, or decision-making. The other authors declare that there are no conflicts of interest.
Ethical approval and consent to participate
Not applicable.
Consent for publication
Not applicable.
Copyright
© The Author(s) 2025.
Supplementary Materials
REFERENCES
1. Edgington, J.; Vispute, S.; Li, R.; Deberghes, A.; Seitz, L. C. Quantification of electrochemically accessible iridium oxide surface area with mercury underpotential deposition. Sci. Adv. 2024, 10, eadp8911.
2. Cao, X.; Huo, J.; Li, L.; et al. Recent advances in engineered Ru-based electrocatalysts for the hydrogen/oxygen conversion reactions. Adv. Energy. Mater. 2022, 12, 2202119.
3. Guo, J.; Huo, J.; Liu, Y.; et al. Nitrogen-doped porous carbon supported nonprecious metal single-atom electrocatalysts: from synthesis to application. Small. Methods. 2019, 3, 1900159.
4. Pei, Z.; Tan, H.; Gu, J.; et al. A polymeric hydrogel electrocatalyst for direct water oxidation. Nat. Commun. 2023, 14, 818.
5. Chen, X.; Zhang, Z.; Chen, Y.; et al. Research advances in earth-abundant-element-based electrocatalysts for oxygen evolution reaction and oxygen reduction reaction. Energy. Mater. 2023, 3, 300031.
6. Zhao, Y.; Shen, Z.; Huo, J.; et al. Epoxy-rich Fe single atom sites boost oxygen reduction electrocatalysis. Angew. Chem. Int. Ed. 2023, 62, e202308349.
7. Wang, P.; Lin, Y.; Xu, Q.; et al. Acid-corrosion-induced hollow-structured NiFe-layered double hydroxide electrocatalysts for efficient water oxidation. ACS. Appl. Energy. Mater. 2021, 4, 9022-31.
8. Chen, X.; Liu, J.; Yuan, T.; et al. Recent advances in earth-abundant first-row transition metal (Fe, Co and Ni)-based electrocatalysts for the oxygen evolution reaction. Energy. Mater. 2022, 2, 200028.
9. Zhao, J.; Guo, Y.; Zhang, Z.; et al. Out-of-plane coordination of iridium single atoms with organic molecules and cobalt-iron hydroxides to boost oxygen evolution reaction. Nat. Nanotechnol. 2025, 20, 57-66.
10. He, B.; Deng, Q.; Wang, Y.; et al. Modification of surface electronic structure via Ru-doping: porous Ru-CoFeP nanocubes to boost the oxygen evolution reaction. J. Power. Sources. 2022, 537, 231506.
11. Lei, Y.; Zhang, L.; Xu, W.; et al. Carbon-supported high-entropy Co-Zn-Cd-Cu-Mn sulfide nanoarrays promise high-performance overall water splitting. Nano. Res. 2022, 15, 6054-61.
12. Xu, H.; Yang, J.; Ge, R.; et al. Carbon-based bifunctional electrocatalysts for oxygen reduction and oxygen evolution reactions: optimization strategies and mechanistic analysis. J. Energy. Chem. 2022, 71, 234-65.
13. Luo, Y.; Wei, L.; Geng, H.; Zhang, Y.; Yang, Y.; Li, C. C. Amorphous bimetallic oxides Fe-V-O with tunable compositions toward rechargeable Zn-Ion batteries with excellent low-temperature performance. ACS. Appl. Mater. Interfaces. 2020, 12, 11753-60.
14. Wang, Y.; Zhang, L.; Meng, X.; et al. Scalable processing hollow tungsten carbide spherical superstructure as an enhanced electrocatalyst for hydrogen evolution reaction over a wide pH range. Electrochim. Acta. 2019, 319, 775-82.
15. Gultom, N. S.; Abdullah, H.; Hsu, C.; Kuo, D. Activating nickel iron layer double hydroxide for alkaline hydrogen evolution reaction and overall water splitting by electrodepositing nickel hydroxide. Chem. Eng. J. 2021, 419, 129608.
16. Hao, H.; Li, Y.; Wu, Y.; et al. In-situ probing the rapid reconstruction of FeOOH-decorated NiMoO4 nanowires with boosted oxygen evolution activity. Mater. Today. Energy. 2022, 23, 100887.
17. Xiao, Y.; Chen, X.; Li, T.; et al. Mo-doped cobalt hydroxide nanosheets coupled with cobalt phosphide nanoarrays as bifunctional catalyst for efficient and high-stability overall water splitting. Int. J. Hydrogen. Energy. 2022, 47, 9915-24.
18. Jin, Y.; Huang, S.; Yue, X.; Du, H.; Shen, P. K. Mo- and Fe-modified Ni(OH)2/NiOOH nanosheets as highly active and stable electrocatalysts for oxygen evolution reaction. ACS. Catal. 2018, 8, 2359-63.
19. Xiong, S.; Wang, L.; Chai, H.; Xu, Y.; Jiao, Y.; Chen, J. Molybdenum doped induced amorphous phase in cobalt acid nickel for supercapacitor and oxygen evolution reaction. J. Colloid. Interface. Sci. 2022, 606, 1695-706.
20. Su, X.; Wang, Y.; Zhou, J.; Gu, S.; Li, J.; Zhang, S. Operando spectroscopic identification of active sites in nife prussian blue analogues as electrocatalysts: activation of oxygen atoms for oxygen evolution reaction. J. Am. Chem. Soc. 2018, 140, 11286-92.
21. Shi, Z.; Yu, Z.; Guo, J.; et al. Lattice distortion of crystalline-amorphous nickel molybdenum sulfide nanosheets for high-efficiency overall water splitting: libraries of lone pairs of electrons and in situ surface reconstitution. Nanoscale 2022, 14, 1370-9.
22. Chen, Z. J.; Zhang, T.; Gao, X. Y.; et al. Engineering microdomains of oxides in high-entropy alloy electrodes toward efficient oxygen evolution. Adv. Mater. 2021, 33, 2101845.
23. Qiu, Y.; Jia, Q.; Yan, S.; Liu, B.; Liu, J.; Ji, X. Favorable amorphous-crystalline iron oxyhydroxide phase boundaries for boosted alkaline water oxidation. ChemSusChem 2020, 13, 4911-5.
24. Sheng, H.; Qu, H.; Zeng, B.; et al. Enriched Fe doped on amorphous shell enable crystalline@amorphous core-shell nanorod highly efficient electrochemical water oxidation. Small 2023, 19, 2300876.
25. Li, D.; Qin, Y.; Liu, J.; et al. Dense crystalline-amorphous interfacial sites for enhanced electrocatalytic oxygen evolution. Adv. Funct. Mater. 2022, 32, 2107056.
26. Zhang, B.; Zheng, X.; Voznyy, O.; et al. Homogeneously dispersed multimetal oxygen-evolving catalysts. Science 2016, 352, 333-7.
27. Zhang, Y.; Gao, F.; Wang, D.; et al. Amorphous/crystalline heterostructure transition-metal-based catalysts for high-performance water splitting. Coord. Chem. Rev. 2023, 475, 214916.
28. Lyons, M. E.; Floquet, S. Mechanism of oxygen reactions at porous oxideelectrodes. Part 2-oxygen evolution at RuO2, IrO2 and IrxRu1-xO2 electrodes in aqueous acid and alkaline solution. Phys. Chem. Chem. Phys. 2011, 13, 5314-35.
29. Fan, X.; Liu, Y.; Chen, S.; et al. Defect-enriched iron fluoride-oxide nanoporous thin films bifunctional catalyst for water splitting. Nat. Commun. 2018, 9, 1809.
30. Luo, M.; Zhao, Z.; Zhang, Y.; et al. PdMo bimetallene for oxygen reduction catalysis. Nature 2019, 574, 81-5.
31. Li, S.; Bai, L.; Shi, H.; et al. Mo-doped CoP nanosheets as high-performance electrocatalyst for HER and OER. Ionics 2021, 27, 3109-18.
32. Liu, Y.; Liu, P.; Men, Y. L.; et al. Incorporating MoO3 patches into a Ni oxyhydroxide nanosheet boosts the electrocatalytic oxygen evolution reaction. ACS. Appl. Mater. Interfaces. 2021, 13, 26064-73.
33. Yu, L.; Wu, L.; Mcelhenny, B.; et al. Ultrafast room-temperature synthesis of porous S-doped Ni/Fe (oxy)hydroxide electrodes for oxygen evolution catalysis in seawater splitting. Energy. Environ. Sci. 2020, 13, 3439-46.
34. Wang, H.; Qi, J.; Yang, N.; et al. Dual-defects adjusted crystal-field splitting of LaCo1-xNixO3-δ hollow multishelled structures for efficient oxygen evolution. Angew. Chem. Int. Ed. 2020, 59, 19691-5.
35. Pan, Y.; Gao, J.; Lv, E.; et al. Integration of alloy segregation and surface Co-O hybridization in carbon-encapsulated CoNiPt alloy catalyst for superior alkaline hydrogen evolution. Adv. Funct. Mater. 2023, 33, 2303833.
36. Jeghan, S. M. N.; Kim, J.; Lee, G. Hierarchically designed CoMo marigold flower-like 3D nano-heterostructure as an efficient electrocatalyst for oxygen and hydrogen evolution reactions. Appl. Surf. Sci. 2021, 546, 149072.
37. Zhang, H.; Xia, B.; Wang, P.; et al. From waste to waste treatment: mesoporous magnetic NiFe2O4/ZnCuCr-layered double hydroxide composite for wastewater treatment. J. Alloys. Compd. 2020, 819, 153053.
38. He, R.; Li, M.; Qiao, W.; Feng, L. Fe doped Mo/Te nanorods with improved stability for oxygen evolution reaction. Chem. Eng. J. 2021, 423, 130168.
39. Huang, Y.; Wu, Y.; Zhang, Z.; Yang, L.; Zang, Q. Rapid electrodeposited of self-supporting Ni-Fe-Mo film on Ni foam as affordable electrocatalysts for oxygen evolution reaction. Electrochim. Acta. 2021, 390, 138754.
40. Wei, Y.; Li, W.; Li, D.; Yi, L.; Hu, W. Amorphous-crystalline cobalt-molybdenum bimetallic phosphide heterostructured nanosheets as Janus electrocatalyst for efficient water splitting. Int. J. Hydrogen. Energy. 2022, 47, 7783-92.
41. Ali, U.; Sohail, K.; Liu, Y.; Yu, X.; Xing, S. Molybdenum and phosphorous dual-doped, transition-metal-based, free-standing electrode for overall water splitting. ChemElectroChem 2021, 8, 1612-20.
42. Ma, Y.; Dong, X.; Wang, Y.; Xia, Y. Decoupling hydrogen and oxygen production in acidic water electrolysis using a polytriphenylamine-based battery electrode. Angew. Chem. Int. Ed. 2018, 57, 2904-8.
43. Zhang, Z.; Jia, C.; Ma, P.; et al. Distance effect of single atoms on stability of cobalt oxide catalysts for acidic oxygen evolution. Nat. Commun. 2024, 15, 1767.
44. Zhang, M.; Liu, Y.; Liu, B.; Chen, Z.; Xu, H.; Yan, K. Trimetallic NiCoFe-layered double hydroxides nanosheets efficient for oxygen evolution and highly selective oxidation of biomass-derived 5-hydroxymethylfurfural. ACS. Catal. 2020, 10, 5179-89.
45. Ye, C.; Wang, M. Q.; Bao, S. J.; Ye, C. Micropore-boosted layered double hydroxide catalysts: EIS analysis in structure and activity for effective oxygen evolution reactions. ACS. Appl. Mater. Interfaces. 2019, 11, 30887-93.
46. Sun, S.; Li, H.; Xu, Z. J. Impact of surface area in evaluation of catalyst activity. Joule 2018, 2, 1024-7.
47. Wei, C.; Xu, Z. J. The Comprehensive Understanding of
48. Yang, H.; Wang, C.; Zhang, Y.; Wang, Q. Green synthesis of NiFe LDH/Ni foam at room temperature for highly efficient electrocatalytic oxygen evolution reaction. Sci. China. Mater. 2019, 62, 681-9.
Cite This Article
How to Cite
Download Citation
Export Citation File:
Type of Import
Tips on Downloading Citation
Citation Manager File Format
Type of Import
Direct Import: When the Direct Import option is selected (the default state), a dialogue box will give you the option to Save or Open the downloaded citation data. Choosing Open will either launch your citation manager or give you a choice of applications with which to use the metadata. The Save option saves the file locally for later use.
Indirect Import: When the Indirect Import option is selected, the metadata is displayed and may be copied and pasted as needed.
About This Article
Copyright
Data & Comments
Data
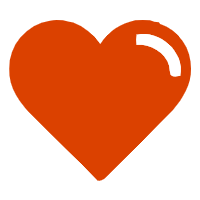
Comments
Comments must be written in English. Spam, offensive content, impersonation, and private information will not be permitted. If any comment is reported and identified as inappropriate content by OAE staff, the comment will be removed without notice. If you have any queries or need any help, please contact us at [email protected].