Carbon footprint of beef cattle systems in the Southeast United States
Abstract
Grasslands in the Southeast United States (SE US) cover 15.8 million ha and most of this area is dedicated to beef production systems. This region holds 6.3 million beef cows and 12.1 million cattle, including calves. Beef cattle systems in the SE US are mostly cow-calf based, and most of the greenhouse gas emission from cattle occurs during this phase (cow-calf) because of their forage-based diet. This review assessed the carbon footprint
Keywords
INTRODUCTION
A carbon footprint (C footprint) is the emission of all greenhouse gases (GHGs) during a given period by any activity or entity[1]. Assessing GHG emissions from agricultural activities is the first step to developing pathways for reducing these emissions. In 2020, agricultural activities in the United States (US) were responsible for emissions of 5981.4 million metric tons of CO2eq, or 11.2% of total US GHG emissions[2]. Enteric fermentation from ruminants is the largest anthropogenic source of methane emissions in the US, and in 2020 accounted for 175.2 million tons CO2eq of CH4, representing 26.9% of total CH4 emissions, or approximately 32% of all agricultural emissions in the US[2]. Because of the large energy sector in the US, agriculture emissions typically fluctuate between 9% and 11% of total emissions. Globally, however, agriculture represents a large proportion (31%) of total emissions, considering that developing countries have a major segment of their economy based on agricultural activities[3]. The absolute emission (not the proportion), however, is the most important indicator if the goal is to reduce atmospheric concentrations of GHGs.
The southeast region of the continental US, as defined by the US Geological Survey, includes 10 states (Florida, Georgia, Alabama, Louisiana, Arkansas, Mississippi, South Carolina, North Carolina, Tennessee, and Kentucky). These states cover almost 1.3 million km2 and have 6.3 million beef cows and 12.1 million cattle, including calves[4]. Beef production systems in these states are predominantly cow-calf operations and weaned calves are typically shipped to the mid-west and Southern Great Plains for finishing in feedlots. In some instances, stocker systems help to add cheaper gains before sending cattle to feed yards. A small proportion of the cattle are finished and processed in this region, mainly because of environmental conditions and distance from grain production areas. Grassland resources, including pasture, range, and crops used for grazing, cover 15.8 million ha in the SE US[5,6]. These resources are an important component of the US food system, producing animal-source food and generating income for numerous producers and allied industries.
The cow-calf phase of the North American beef cattle industry is responsible for a large portion of the enteric fermentation emissions produced in beef systems. In the Southeast US (SE US), the cow-calf phase is typically based on warm-season perennial C4 grasses along the Gulf Coast States, including species such as bahiagrass (Paspalum notatum Flüggé) and bermudagrass [Cynodon dactylon (L.) Pers.]. Factors that contribute to greater emissions from the cow-calf phase include maintaining the cow for the entire year and the calf until weaning date. In addition, these systems are typically extensive, and forage is often the only source of feed available. Forage-based diets produce a greater proportion of total volatile fatty acids as acetate, generating more hydrogen[6]. Therefore, it is expected that forage-based systems used in the cow-calf phase will generate more methane per unit of carcass weight (CW) than feedlot systems with high-grain diets. Rotz et al. estimated that the cow-calf phase is responsible for almost 70% of the GHG emissions in US beef systems[7].
It is important to note, however, the role of grassland systems in capturing GHG and storing organic carbon (C) in soil organic matter (SOM). Saggar et al. and Raposo et al. demonstrated that intensive grasslands can also be a significant sink for CH4[8,9]. In a broad assessment of Canadian beef farms, Alemu et al. concluded that the emission intensity averaged 23.9 kg CO2eq kg-1 live weight[10]. Rotz et al. investigated potential regional differences in the US beef systems[7]. The C footprint tended to increase from west to east because of greater use of inputs in the east, such as nitrogen (N) fertilizer and lime, related to greater production potential arising from greater rainfall. Soil type was also an important factor affecting the C footprint. Soils with greater clay content have a greater capacity to store soil organic carbon (SOC). Rotz et al. indicated that beef producers might be able to reduce the C footprint of their systems by improving some management aspects, but other factors such as rainfall and soil type are out of their control[7]. This review will address the C footprint of beef cattle systems in the SE US, focusing on C stocks and flows in these systems and practices to reduce GHG emissions.
CARBON STOCKS, FLOWS, AND POTENTIAL SEQUESTRATION RATES IN THE SE US
Beef cattle production in the SE US is predominantly cow-calf and pasture-based systems. Grassland ecosystems have many C pools, including SOM and soil organisms, belowground plant tissues (e.g., roots and rhizomes), aboveground vegetation, mesofauna, and herbivores. These pools interact and affect the C cycle, and C stocks and flows are key components of the C cycle in terrestrial systems. SOM followed by belowground biomass (roots and rhizomes) is the major organic C reservoir in the soil-plant-animal nexus in grassland ecosystems[11]. Aboveground biomass, including vegetation, litter, and excreta, accounts for less than 15% of total organic C pool. This estimate does not include livestock biomass; however, considering a stocking rate of 600 kg live weight per ha, this would be a negligible amount of organic C per ha (approx.
Organic carbon pools in an intensively managed (3 animal units ha-1 and 200 kg nitrogen ha-1 yr-1) bahiagrass (Paspalum notatum) pasture on a spodosol in Florida, United States
Pool | Total dry matter | Carbon stock |
kg ha-1 yr-1 | ||
Leaves | 6347 (5.0)* | 3173 (4.5) |
Stem | 1269 (1.0) | 635 (0.9) |
Roots + rhizomes | 31,967 (25.4) | 20,459 (29.1) |
Litter | 6084 (4.8) | 2738 (3.9) |
Cattle dung | 2700 (2.1) | 1350 (1.9) |
Urine (fresh matter) | 5400 (4.3) | 0 (0) |
kg ha-1 | ||
SOM**, horiz. A (0-15 cm) | 26,667 (21.2) | 15,467 (22.0) |
SOM, horiz. E (15-33 cm) | 16,316 (12.9) | 9463 (13.5) |
SOM, horiz. Bt (33-90 cm) | 29,245 (23.2) | 16,962 (24.1) |
Although the aboveground pool represents a minor portion of the total organic C pool, it decays faster and is more dynamic than the other portions[13]. Fresh deposited litter and dung typically decay approximately 50%-70% per year[14], much faster than SOM (3%-5%)[15]. In the short term, these fast-decaying pools are more affected by management practices than the total SOM. Management practices can shift the pathways of returns (i.e., litter vs. excreta) and affect the C footprint of livestock systems[16]. Increasing the stocking rate will result in a greater proportion of nutrients cycled via excreta, resulting not only in greater GHG emissions from dung and urine patches, but also increased methane emissions through enteric fermentation. Reducing stocking rate and improving grazing management has a powerful effect on the reduction of GHG emissions in a grazing system. The direct effect is the reduction of animal numbers per unit area, but these animals might have greater daily gains because of improved forage selection, less time needed to reach a similar body weight, and improved C sequestration because of greater primary productivity resulting from improved management[17]. Cardoso et al. compared five different pasture management systems and demonstrated that total carcass production, the area required to produce the same quantity of product (m2 kg-1 hot carcass weight, HCW), and the total GHG emissions per kg of HCW were affected by management intensity[18].
Major C inflows in a grassland ecosystem include photosynthesis (plants and autotrophic microorganisms) and C uptake by soil microorganisms. Methanotrophic bacteria in grassland soils contribute to reducing atmospheric methane, and the global uptake estimate is 3.73 Tg year-1[19]. Beef systems in the SE US include tropical/subtropical zones where the annual solar radiation is abundant, and receive greater rainfall than the Western US. These conditions are important for increasing primary ecosystem productivity because of greater photosynthetic rates. At the same time, C decomposition might be faster, leading to C losses. The balance between these processes and interactions with the livestock component are key to shifting the balance towards greater C sequestration rates. Carbon outflows include respiration from soil organisms, plant respiration (above- and belowground tissues), organic matter decomposition, and livestock gas emissions from enteric fermentation and excreta. Methane emissions from methanogenic organisms in wetlands might also be a large component of GHG emissions, and this ecosystem is present in some beef cattle systems along the coastline.
Land use has a marked effect on the C footprint of agroecosystems. In the SE US, major types of land use areas include natural rangelands, improved pastures, wetlands, pine tree plantations, or natural forests. At the farm level, these mosaics of land use areas affect the overall potential of the farm unit to offset GHG emissions from the livestock system. Annual C sequestration rates range from as low as 0.5 Mg C ha-1 yr-1 in low input pastures up to 8 Mg C ha-1 yr-1 when changing from cropland to intensive pine tree systems, including above- and belowground C [Table 2]. Therefore, combining distinct land use types within a farm makes it possible to increase the potential annual C sequestration rates and be able to offset the GHG emissions from livestock. Pine tree plantations are key to sequestering more C that will stay longer in the soil and generate products (e.g., lumber for construction) that will last longer in the sequestered form. Using 10%-30% of the farm for pine trees might generate enough C credits to offset the emissions. The establishment of trees should be coupled with the improved management of the remaining system if the goal is to sustain the same stocking rate. These practices have the potential to increase C sequestration in grassland ecosystems and offset GHG emissions. That includes integration of forage legumes, rotational stocking, and grazing management to optimize forage growth. If improved practices are not incorporated, the farm will have to reduce the stocking rate. Timber income can offset the reduction in livestock income[31]. Regarding the timber payback time, integrated systems such as silvopastoral or integrated crop-livestock systems could be used as alternative sources to maintain the profitability of the area over the years of tree growth. It is important to consider that several areas are not suitable for pine tree plantation. However, there are other options, including natural ecosystems in the region, that have a large potential to sequester C and could be used to offset emissions as well while providing synergies and trade-offs with the addition of other important ecosystem services. Natural rangelands, for example, not only sequester C but also have high biodiversity and serve as an important habitat for wildlife, as well as filtering water and recharging aquifers.
Major land use types in the Southeastern United States and their annual carbon (C) sequestration rates
Land use | C sequestration rates (Mg C ha-1yr-1) |
Pine forest1 | 6 |
Natural forest1 | 4 |
Natural rangeland2 | 0.9-3.2 |
Low input bahiagrass pasture3 | 0.5 |
Improved pasture3 | 1 |
Cover crop4 | 0.3-0.6 |
Land use change (crop to pasture)5 | 2.3 |
Land use change (crop to forest)1 | 4.0-8.0 |
Wetlands6 | 2 |
Climate-smart practices might also affect different sources and sinks of C in agroecosystems. Some of these practices also have synergies and trade-offs that can enhance the delivery of other important ecosystem services. Table 3 summarizes the different C sources and sinks, and how climate-smart practices could affect the overall C footprint of the system.
Climate-smart practices to offset greenhouse gas (GHG) emissions and/or increase carbon sequestration in forestry and livestock systems
Source/Sink | Climate smart practices | Net C sequestration and/or GHG emission reduction | Synergies and trade-offs |
Biomass C stock change | Afforestation and/or Silvopasture System with long-lasting products | 4 to 8 Mg C ha-1 yr-1(1) | Habitat for wildlife, water catchment and purification |
Soil organic C stock | Afforestation | 4 to 8 Mg C ha-1 yr-1(1) | Nutrient cycling, biological nitrogen fixation, habitat for wildlife, biodiversity |
Legume integration | |||
Reduced tillage | |||
Cover crop | 0.3 to 0.6 Mg C ha-1 yr-1(2) | ||
Native rangeland | 0.9 to 3.2 Mg C ha-1 yr-1(3) | ||
Wetland restoration | 0.5 to 3.3 Mg C ha-1 yr-1(4) | ||
Land use change from crop to pasture/forest | 2.3 to 8.0 Mg C ha-1 yr-1(5) | ||
Soil nutrient management | |||
Direct N2O emissions from mineral soils | Reduced tillage | Improve nutrient use efficiency | |
Slow-release N fertilizer | |||
Manure directly applied to pasture | |||
Biochar | |||
Methane uptake by soils | Afforestation | Carbon sequestration | |
Land change from cropland to pasture | |||
Enteric fermentation | Dietary changes including use of nitrates, condensed tannins, fibrous concentrate, addition of dietary fat, digestive enzymes, reproductive technology to enhance efficiency | 11% reduction in CH4 emission w/ nitrate(6); 26% reduction in CH4 w/ dietary fat(7); 10% reduction in CH4 emission w/ essential oil(8); 5.4% reduction in CH4 yield (g/kg DMI) w/ condensed tannins(9) | Improved livestock performance |
Methane emissions from facilities and/or storage | Manure storage management | Reduce nutrient losses and improve manure utilization | |
Animal diet | |||
Nitrous oxide emissions from excreta | Feed additives that might reduce emissions from excreta (e.g., condensed tannins); manure handling (direct application to fields as effluent irrigation ) | Reduce N losses and enhance nutrient cycling | |
GHG emissions from fossil fuels | Forage stockpiling Legume integration (BNF*) Local market | Development of local market |
HOW MUCH C SEQUESTRATION IS NEEDED TO OFFSET GHG EMISSIONS FROM BEEF CATTLE SYSTEMS IN THE SE US?
Methane emissions from livestock are considered to be major contributors to overall GHG emissions, affecting the sustainability of the beef industry. Measurements of methane emission in cow-calf systems under grazing conditions are very limited in the SE US, and on many occasions, estimates are based on modeling[10]. Rotz et al. indicated that the C footprint in SE US beef systems is 28.9 kg CO2eq kg-1 CW, which is greater than the average US beef system (21.3 kg CO2eq kg-1 CW)[7]. Predominance of cow-calf systems and greater use of fertilizer inputs are key components that increase the C footprint of SE US beef systems[37]. The cow-calf phase is by far the most important in terms of the amounts of GHG released to the atmosphere[7].
Producing locally could reduce the C footprint of beef systems. In the SE US, that would mean that systems would have not only to stay in the cow-calf phase, but also have backgrounding and finishing animals to process locally. On the basis of several assumptions, we estimated the necessary C sequestration per ha to finish one beef steer. If we use a mean of 28.9 kg CO2eq kg-1 CW, as suggested by Rotz et al., for SE US conditions, assuming one cow-calf pair per ha for the initial 240 days (birth to weaning)[7], and an additional 0.5 ha to background the steer from d 240 to d 706[38], 1.5 ha would be needed for the cow-calf and backgrounding/finishing phases. Adding 20% more land for heifer development to replace culled cows, the system would need 1.8 ha to produce a steer (cow-calf and backgrounding/finishing phases). Assuming a steer weight of 570 kg (1256 lb) at slaughtering (706 days of age) and a CW of 302 kg (666 lb; 53% dressing percentage[39], we calculate that the emissions would be 8728 kg CO2eq per steer
LAND USE AND LAND COVER MANAGEMENT
Beef cattle systems in the SE US are characterized by several different land cover management scenarios. Improved practices in these different management systems could be used as alternatives to reduce GHG emissions. Cattle and timber, for example, are two of the most common farm diversification practices we find in the SE US, and a number of farms explore both commodities. In addition to silvopastoral systems, it is common to find areas of timber plantation adjacent to pasture land within the same farm. In terms of preservation of natural ecosystems, it is common in Florida to use natural rangelands and to preserve wetlands. These are natural ecosystems that can help to offset the C footprint of the overall farm. Regarding grazing systems, different practices of grazing management are applied daily in most of the beef farms in the SE US, because the predominant system is pasture-based cow-calf operations. First and foremost is the adjustment of stocking rate to the herbage mass, which is also considered the main grazing management strategy. As another important management practice, stockpiled limpograss [Hemarthria altissima (Poir.) Stapf & C.E.Hubb] has been widely used in South Florida. Rotational stocking is less common, but this has recently been done in some beef farms. In addition, it is typical to overseed grass-legume mixtures on perennial pastures. In some regions, it is common to have tall fescue (Festuca arundinacea Schreb) and white clover (Trifolium repens L.). In South Florida, shyleaf (Aeschynomene americana L.) is commonly seeded on bahiagrass or limpograss pastures. Finally, it is becoming more popular to use alfalfa (Medicago sativa L.)-bermudagrass mixtures, and bahiagrass-perennial peanut (Arachis glabrata Benth) swards. The implement ation of conservation easements has also increased in recent years in Florida. Conservation of pristine land with natural ecosystems can be carried out alongside beef cattle production, provided the producer continues using the same practice without developing the land for housing. There are also examples in Alabama and Georgia of conversion from cropland to perennial pastures, resulting in a large increase in SOC. These practices are all found in beef cattle systems in the SE US, and are described below in detail as alternatives to reduce GHG emissions from beef cattle systems.
Afforestation
Forest ecosystems are key to climate change debate because of their potential role as a C sink or source according to their management, age, and environmental conditions[41]. Afforestation, which is the conversion of long-term non-forested land to forest land[42], can be an alternative practice to reduce the C footprint of beef cattle systems in the SE US by increasing C sequestration in portions of grazing lands[43,44]. In addition, this practice is also recognized as a cost-effective climate mitigation strategy, making C sequestration a "no-regrets" mitigation option[44,45]. Multiple economic studies have shown that farmers are able to avoid significant quantities of GHG emissions by exchanging portions of pasture and cropland for trees; nevertheless, they must be able to convert the biomass and the C stored in the soils into income to maintain this practice[46,47].
The C sequestration potential of an afforestation practice is associated with the amount of C that is sequestered by the soil and the belowground biomass[48]. Long-lasting forest products should also be considered (e.g., lumber for construction). Mazzetto et al. emphasized that when compared with agroforestry, the greater C footprint from beef production could be offset by C sequestration through afforestation on land spared from cattle production[49]. Mishurov et al. assessed N2O flux dynamics of grassland undergoing afforestation and found that during the establishment phase, mechanical disturbances increased N2O emissions at first, but the intensity of the flux dropped to one-third in the following three years[50]. However, an improved understanding of afforestation at a regional, national, and global level is still required, including socioeconomic viability, land-use changes, use of species, and future climate variations.
Preservation of natural ecosystems
Loss of agricultural land to urbanization and other land-use changes is a major threat to agricultural enterprises[51]. It is estimated that urban expansion will result in a 1.8%-2.4% loss of global croplands by 2030, with differences across regions[52]. The SE US is characterized by rapid population growth and increasing urban land use[52]. This presents a challenge, especially because many of the natural ecosystems, especially forests, in the SE US are considered net C sinks[21]. Therefore, land-use change towards urban development plays a direct role in decreasing the area of natural ecosystems. For instance, Florida is among the states facing the greatest threat of urbanization, with declining agricultural land, especially in the southern peninsula of the state[53]. Similarly, the conversion of riparian areas to grassland or forest can initially reduce SOC storage by removal of roots in the soil, leading to accelerated erosion. However, unlike urbanization, these effects often can be reversed in subsequent years through the growth of trees or grassland. Florida’s native rangeland has been shown to accumulate > 11 Mg C ha-1 in 4 years, even when fire is incorporated as a management practice[23]. These native rangelands extend over 3.1 million ha in the state, further indicating their importance for sustaining C balance[23].
Nutrient flows and hydrological balance are additionally important aspects for preservation of natural ecosystems. The potential loss of agricultural land to urbanization can have an effect on the water balance across entire regions. In North Carolina, intensive urbanization elevated peak watershed flow rates and increased the annual discharge volumes (rainwater) across an entire watershed[54]. In parallel, increasing land-use change in the metro-Atlanta region has also increased water demands from a growing population[55]. Agricultural water use of the Apalachicola-Chattahoochee-Flint River Basin has become disputed, with many users having reduced water resources and placed potential limits on production[55]. The threats of urbanization and other land-use changes will continue to be present, but it is critical to understand the value of natural ecosystems in terms of their benefits. Placing value on their ecosystem services is an important step to ensure conservation and longevity of natural ecosystems.
Agricultural practices
Agriculture defines the Southern culture, and this industry, including production, processing, and distribution, has an important role in the economy of the southern states. The region offers great potential for agricultural productivity because of favorable climatic conditions, such as mild winters, hot summers, and abundant precipitation[56]. In the past, tobacco, cotton, and rice were the most common cash crops in the region and have been more recently replaced by soybean and corn. In terms of animal agriculture, the southeast region, except Florida, is characterized by smaller production units of cow-calf operations
Conservation agriculture management has been implemented with positive results in terms of SOC, particularly in the SE US. The United States Department of Agriculture (USDA) agricultural inventory[4] shows an increase in conservation practices adopted by some southeastern states. As an example, Georgia has 0.7 million farms where owners place their land into conservation easements to protect it from development. Similarly, the implementation of agroforestry practices, such as alley cropping that produces trees and other crops on the same acreage, is an example of management technologies adopted to increase conservation. The benefits of alley cropping include wind protection, increased plant diversity, and wildlife habitat. Silvopasture is another practice that combines livestock, forage, and tree production on the same land, providing large quantities of C sequestered by the trees, greatly contributing to a reduction in the net emissions of the system. At the same time, the trees provide shade for the grazing animals, which in turn can decrease heat stress and increase growth performance and animal well-being[4].
Federal and state governments have programs to help farmers establish conservation practices. These programs are voluntary, with farmers entering into contracts with the Natural Resource and Conservation Services (NRCS) to meet conservation guidelines on their land. Consequently, the farmer earns monetary incentives, usually in the form of cost-share assistance, to establish and maintain the practices. These incentives can make conservation practices affordable and lead to sustained adoption over time[60].
Integration of legumes into grass pastures is a practice that is being increasingly adopted, mainly because of high fertilizer prices. Subtropical and tropical grasses are well adapted to the soil and weather conditions in the Southern US, and cow-calf operations rely heavily on these grasses. Several studies report that the integration of legumes in beef-forage systems increases forage production and animal weight gain, reduces the use of fertilizers and irrigation, and increases nutrient cycling[61-63]. The development of forage production systems that have less reliance on N fertilizer is an important step towards reducing C footprints and increasing economic sustainability. Furthermore, forage legumes could potentially benefit soil health by increasing the presence of certain microorganisms that enhance nutrient cycling, maintaining N that is readily available for plant uptake. Although legumes can emit N2O indirectly by microbial decomposition of plant material or by return of rich N excreta from grazing animals, these emissions are expected to be less than emissions from the N fertilizers, either because of the production energy costs of fertilizers or greater synchronism with plant demand, reducing the mineral N content available in the soil[64,65]. In addition, management practices to disperse excreta over the pasture can be useful to decrease the excess of mineral N applied in the same spot to reduce the N2O emissions by this pathway.
The adoption of cover crops is another management practice that is increasing in popularity because of the documented benefits to the system. Some of these benefits include pest control, increasing in residue persistence, N fixation, compaction reduction, nutrient scavenging, increased forage quality, attraction of beneficial insects, and weed and erosion control[66]. The selection of a plant or mixture of plants for a cover crop should meet the producer’s objectives while being easy to establish, having an early growth rate, optimal rooting depth, high biomass yield, pest resistance, ease of termination, and accessible cost. Considering all this, the contribution of cover crops to decreased net GHG emissions in beef-forage systems stems from their ability to increase SOC while decreasing fossil fuel fertilizer use.
Another strategy that plays a significant role in the reduction of GHG emissions from soils is the reduction of tillage practices in an effort to reduce SOC oxidation and release to the atmosphere as CO2. Tillage increases production costs, affects yield, increases soil compaction and erosion, and affects water quality via increased runoff and leaching. According to the USDA, tillage has been reduced since 2012 and the use of non-tillage methods has increased over the years. The benefits of non-tillage methods are well documented; such benefits include improving soil health, reducing labor costs, and contributing to annual fuel savings, which directly reduce the GHG emissions of the system[67,68]. No-till systems also reduce pollution of water systems, hence having additional benefits that extend beyond the farm site. The decrease in fossil fuel usage by reducing tillage practices may be one of the largest contributions to lowering the overall C footprint of food production systems. Furthermore, the additional environmental benefits of non-till methods, such as reduced soil erosion, increased soil biological activity, and increased SOM, all contribute directly to increased C sequestration by the soil. Several practices have been developed to increase SOC sequestration, such as the use of conservation tillage in crops, and perennial pastures[56]. For example, Franzluebbers[56] reported that the establishment of perennial pasture following conventional tilling of cropland can also lead to significantly positive SOC sequestration with a moderate stocking rate; however, when the stocking rate increased, SOC decreased. This highlights the effect of grazing management strategies on the potential for C sequestration of beef and forage systems. Farm policy is moving to more intensified on-farm conservation to further environmental stewardship while still promoting farm profitability and agricultural sustainability[60]. The interest and demand for carbon credits from the private sector is growing, and producers adopting non-tillage and cover crop practices may be eligible for additional economic revenues in terms of carbon credits. Although the carbon markets in the US are still being developed, this may greatly encourage the adoption of more sustainable agricultural practices.
Slow-release fertilizers can be another option used to decrease GHG and C footprints in grasslands. The benefits of this management practice in reducing N losses via NH3 volatilization and N2O emissions have been reported in multiple sources. For example, coated urea, urease inhibitor (N-(n-butyl) thiophosphoric triamide)-treated urea, and nitrification inhibitor (dicyandiamide)-treated urea reduced emission factors of NH3-N and N2O compared with conventional urea[69]. Furthermore, Liu et al. indicated that broadcast application of urea treated with urease or nitrification inhibitors was able to reduce CO2-C flux, but had no effect on CH4-C flux[69]. Moreover, controlling N release can increase N use efficiency in forage plants, thus increasing forage biomass for an extended period; however, this response may vary according to the source[70]. Grass-fed beef production systems usually have greater C footprints compared with feedlots, and one of the major factors affecting this output is the extended time required for cattle to reach finished body weight[71,72]. Increasing N utilization by forage plants by slowly providing this nutrient will improve the system efficiency[70], decrease the number of days to slaughter, and consequently decrease the C footprint.
Grazing management
From grazing lands to meat packing, beef production systems in the Southern US are striving to meet global demands without compromising environmental quality or local profitability. Both producers and consumers are concerned about the environmental sustainability of production systems, and for this reason, GHG mitigation strategies are quite relevant in grazing systems. Grazing management practices should be implemented to utilize the benefit of forages. Key factors such as leaf area before and after grazing and periods of rest may guarantee forage and livestock production. Proper management of rotational systems improves plant health, soil health, water infiltration, and forage production. Other benefits of proper grazing management include reduced runoff and soil erosion, and reduced production costs resulting from less use of fuel, machinery, fertilizer, and pesticides. Torres et al. simulated GHG emissions for a typical cow-calf farm in the SE US with 100 ha of cropland, a beef herd of 50 cows, and a forested area, where calculated emissions ranged from 348.8 t CO2eq yr-1 to 765.6 t CO2eq yr-1 for the different farm enterprise combinations and production practices[43]. The authors estimated that the forest area required to neutralize these emissions ranged from 19 to 40 ha, corresponding to 13%-27% of the area dedicated to cropland and pasture. This exercise provided a great example of how integration of forestry and cattle with proper grazing management can contribute to decreased GHG emissions (CO2eq) in the system.
The standard practice of finishing beef production in the US since the 1950s has been grain-feeding, which is supported by the increased rate of gain, improved feed efficiency, decreased land required per kg of CW and favorable carcass characteristics such as tenderness, juiciness, and marbling[39]. However, with increased consumer concerns about the environment, human health, and animal welfare, the practice of grass-fed beef is experiencing a greater demand. Fresh grass-fed beef sales in the US have grown from $17 million in 2012 to $272 million in 2016[73]. It is becoming very important to perform a life cycle assessment (LCA) on a regional level to evaluate the profitability and environmental effect of grazing systems, particularly for grass-fed beef. The LCA analysis should include animal performance, herd management practices, transportation, feed inputs, and machinery usage. The advantage of having a wide range of forages with different morphophysiological characteristics and adapted to different management conditions in the southern states brings the opportunity to produce grass-fed beef with a high potential for productivity, profitability, and sustainability outcomes[74].
Finally, the potential of advancements in plant breeding to reduce GHG emissions from beef-forage systems should not be overlooked. For example, the development of new cultivars that have less reliance on N fertilizer could make a great contribution in terms of the overall C footprint of the system. For example, Santos et al. evaluated the potential biological N fixation of six cultivars of bahiagrass under no N fertilization, and root-rhizome responses were detected[62]. This could lead to future cultivar selections based on N fixation capacity, and potentially increase N fixation in species not typically associated with such feature.
LIVESTOCK MANAGEMENT
Dietary changes and feed additives
Ruminant livestock production is among the main contributors to GHG emissions in the form of CH4 (i.e., enteric CH4) and N2O, either from enteric fermentation, manure management, or feed production[17]. Beef cattle production in the SE US is generally characterized as cow-calf production, where 60% of operations are cow-calf only and 23% function as both cow-calf and stocker operations[75,76]. Pastures comprise the main feed source for livestock operations in the region, where warm-season grasses tend to be the backbone of most operations, and some cool-season forages are incorporated into the production systems to alleviate herbage shortages.
Enteric CH4 production is driven by dry matter (DM) intake and forage nutritive value -there is a direct relationship between the feedstuff and microbial capacity to undergo methanogenesis in the rumen[76]. Feeding strategies can reduce the overall C footprint by reducing enteric CH4 emissions. Improvements in forage nutritive value are among the most important factors for reducing enteric CH4 emissions per unit of feed intake and animal product[17]. Comparing enteric CH4 emissions between warm-season (C4) and cool-season (C3) grasses, Archimède et al. reported that cattle consuming warm-season grasses produce 17% more CH4 per unit of intake, compared with cattle consuming cool-season grasses[77]. In North Florida, Garcia[78] reported a 58% decrease in CH4 emissions intensity [e.g., g of CH4 per unit of average daily gain (ADG)] for steers grazing cool-season forages (during the cool-season period), compared with that for the same steers grazing warm-season grass pastures (during the warm season period). On average, steers emitted 96 and 116 g CH4 d-1 when grazing cool-season or warm-season grasses, respectively. The chemical components in the structural carbohydrates, mainly lignin content, in cool-season vs. warm-season grasses are a main driver for the differences in enteric CH4 emissions[77].
Supplementation strategies to meet the nutritional demands of beef cattle are an option to mitigate CH4 emission intensities (kg CH4 kg-1 CW) and improve animal performance[79]. These strategies may not be specifically implemented for altering enteric CH4 emissions; however, emission intensities can be indirectly affected through increases in animal performance[80]. Stackhouse et al. demonstrated that increased animal performance using growth-promoting technologies is a cost-effective way to mitigate the C footprint of beef production, decreasing it by approximately 2.2 kg CO2eq kg-1 CW[81]. Grazing beef cattle are most commonly supplemented in the forms of energy and protein supplementation, which are critical, especially when poor-quality forages are used[80]. Energy supplements typically utilized in the SE US include corn grain, molasses, cottonseed hulls, soybean hulls, or oilseeds. Similarly, protein supplements commonly utilized in SE US beef cattle operations may include soybean meal, cottonseed meal, as well as other non-protein nitrogen (NPN) sources, including urea. Replacement beef heifers consuming poor-quality hays (low protein and high NDF) are recommended to receive low-starch, high protein supplements on a daily basis to improve reproductive development[82]. However, increasing the amount of supplementation does not always equate to increased performance if the proper energy-protein ratio is not provided. Overfeeding in heifers can lead to the animals becoming too fat and thus decreasing their reproductive performance. Beef heifers grazing annual ryegrass (Lollium multiflorum L.) and receiving greater than 1% BW of ground corn or soybean meal had adverse effects on body weight (BW) gain and reproductive performance, compared with heifers receiving 0.5% BW of ground corn or soybean meal as a supplement[83]. The addition of nitrates in place of urea has also been explored as an alternative to reduce CH4 emissions[33]. Supplementation with encapsulated ammonium nitrates and sugar cane molasses reduced nutrient digestibility in beef steers consuming bahiagrass hay, compared with urea supplementation[33]. In the same study, enteric CH4 emissions (g kg-1 BW) were reduced by 11% by the addition of nitrates; however, there is evidence of deleterious effects on performance with the inclusion of nitrates, and further investigations are still warranted[33]. Furthermore, fat supplementation can also be used to reduce CH4 emissions by reducing rumen hydrogen accumulation through the biohydrogenation process and consequently reducing the substrate for methanogenic microbes[84]. However, fat concentrations should not exceed 6%-7% in dietary DM to avoid impairing forage digestibility and animal performance[84].
Reproductive efficiency
Limited forage quality of warm-season grasses found in much of the SE US can often limit the reproductive performance of the cowherd[85]. Considering that the cow-calf sector comprises the majority of beef cattle operations in the region[6], strategies aimed at improving reproductive efficiency at the herd level are important for simultaneously increasing economic returns and decreasing environmental footprints. A cow’s body condition has a direct effect on reproductive traits, with a 30% reduction in pregnancy rates in cows with low body condition scores (≤ 4)[86]. Early weaning of beef replacement heifers has been shown to be an effective means of improving reproductive performance[85]. Many of the cowherds in the SE US have Brahman (Bos indicus) influence. Compared with traditional English breeds (Bos taurus), Brahman crossbred heifers have lower calving rates when bred to calve at 24 mo of age[87]. As a result, producers tend to wait to breed their Brahman crossbred heifers to calve at 3 yr of age[85]. Early weaning has been an effective strategy for improving cow reproduction[85], and consists of weaning calves between 70 and 90 d to reduce energy demands of lactation and improve body condition of the cow prior to rebreeding[88]. Reports in early weaned calf systems have indicated that this management practice improves the cow’s body condition score, increases pregnancy rates, and reduces the postpartum anestrus period by 24 d compared with normal-weaned systems[85,89].
Other management practices that improve cowherd reproductive efficiency include several biotechnological practices, including the use of estrus synchronization, ovulation synchronization, and artificial insemination[90]. These technologies can be employed to shorten the calving season, increase calf uniformity, and improve the genetic merit of the herd through the use of artificial insemination[90]. Adoption of these practices, however, remains low across beef cattle operations[91]. Overall, the National Animal Health Monitoring System (NAHMS) of the USDA Beef 2017 Report reported that most operations (58.7%) did not have a defined breeding season[92].
Breeding and genetics to enhance feed efficiency
Feed efficiency is a complex and multifaceted trait that is controlled by various biological processes[93]. Feed efficiency is the gain in body weight from the consumption of a given amount of feed[94]. Residual feed intake (RFI) is a method for evaluating feed efficiency in beef cattle and is defined as the difference between actual feed intake and the expected DM intake needed for an animal to meet its nutrient requirements for maintenance and production[95], normally as defined in NASEM[96]. Cattle that consume less feed than expected have a negative RFI, which indicates an improvement in feed efficiency[97]. Feeding costs are among the greatest production costs across beef cattle operations, having a direct effect on profitability; thus, selecting animals with low RFI can improve economic returns while also decreasing the environmental input of the production system[93]. RFI has been shown to be moderately heritable and moderately repeatable across diets, and independent of body size and production[93]. In general, animal selection based on RFI is shown to result in cattle that consume less feed DM, have improved feed conversion, and indirectly result in reduced enteric CH4 emissions at similar levels of production[98]. However, some studies have shown that feed efficiency may be genetically correlated with other traits that are beneficial or antagonistic to beef production, suggesting that this factor should be considered in breeding programs based on RFI[99].
Other breeding and genetic improvement tools for reducing methane emissions from livestock are being explored. Among these, evaluations of residual methane emissions (RME) have indicated a potential for providing a ranking index of the methanogenic potential of an animal[100]. The RME is the difference between the animal’s expected and actual methane production, based on the level of feed intake and BW[101]. Ranking cattle in terms of RME can be helpful in selecting low-emitting animals; however, ruminal fermentation and the rumen microbiome play an important role in fermentation pathways[100]. Further understanding of key ruminal microbes associated with phenotypic divergence for RME is necessary to enable the continuous improvement of the use of RME as a tool for selection[100].
FINAL CONSIDERATIONS AND CONCLUSIONS
Beef cattle production systems are one of the most important agroecosystems in the SE US, considering not only the area they cover, but also their economic importance. Livestock releases GHGs, but grasslands also sequester C, which might help to offset some of the GHG emissions from cattle. There are climate-smart practices that allow the reduction of the beef C footprint. These practices can directly reduce the emissions (e.g., dietary interventions) or indirectly by promoting greater C sequestration and reduction of off-farm inputs (e.g., forage legumes, silvopasture systems, afforestation). Producing local beef could be another way to reduce C footprints. Animal well-being can be improved with some of these practices, which is an added benefit. Overall, increasing the efficiency of beef production systems tends to reduce their C footprint because of the reduced time needed to finish the animals. Additionally, reproductive efficiency is key to improving the calf crop in cow-calf systems, which is considered the phase with the greatest GHG emissions. This review summarized potential practices to reduce the C footprint in beef cattle production systems in the SE US. There is potential to produce climate-smart beef that is climate neutral, as long as proper land use and management practices are in place. Beef is a very important food for humans, and it will continue to be for future generations. Developing sustainable beef systems is key for the future of humankind.
DECLARATIONS
Authors’ contributionsWrote a portion of the article, edited, and reviewed the entire manuscript: Dubeux JCB
Wrote a portion of the article: Garcia L, Queiroz LMD, Santos ERS, Oduor KT
Revised the manuscript: Bretas IL
Availability of data and materialsNot applicable.
Financial support and sponsorshipNone.
Conflicts of interestAll authors declared that there are no conflicts of interest.
Ethical approval and consent to participateNot applicable.
Consent for publicationNot applicable.
Copyright© The Author(s) 2023.
REFERENCES
2. Epa U, Change Division C. Inventory of U.S. greenhouse gas emissions and sinks: 1990-2020. Available from: https://www.epa.gov/ghgemissions/inventory-us-greenhouse-gas-emissions-and-sinks [Last accessed on 8 Dec 2022].
3. Crippa M, Solazzo E, Guizzardi D, Monforti-ferrario F, Tubiello FN, Leip A. Food systems are responsible for a third of global anthropogenic GHG emissions. Nat Food 2021;2:198-209.
4. USDA-NASS. Census of Agriculture. 2017. Available from: https://nass.usda.gov/AgCensus/ [Last accessed on 8 Dec 2022].
5. USDA-NASS. Census of Agriculture. 2012. Available from: https://nass.usda.gov/AgCensus/ [Last accessed on 8 Dec 2022].
6. Janssen PH. Influence of hydrogen on rumen methane formation and fermentation balances through microbial growth kinetics and fermentation thermodynamics. Anim Feed Sci Technol 2010;160:1-22.
7. Rotz CA, Asem-hiablie S, Place S, Thoma G. Environmental footprints of beef cattle production in the United States. Agric Syst 2019;169:1-13.
8. Saggar S, Tate KR, Giltrap DL, Singh J. Soil-atmosphere exchange of nitrous oxide and methane in New Zealand terrestrial ecosystems and their mitigation options: a review. Plant Soil 2008;309:25-42.
9. Raposo E, Brito LF, Janusckiewicz ER, et al. Greenhouse gases emissions from tropical grasslands affected by nitrogen fertilizer management. Agron J 2020;112:4666-80.
10. Alemu AW, Amiro BD, Bittman S, Macdonald D, Ominski KH. Greenhouse gas emission of Canadian cow-calf operations: A whole-farm assessment of 295 farms. Agric Syst 2017;151:73-83.
11. Angst G, Mueller KE, Nierop KG, Simpson MJ. Plant- or microbial-derived? Soil Biol Biochem 2021;156:108189.
12. Dubeux Jr JCB, Santos HQ, Sollenberger LE. Nutrient cycling: perspectives of improving sustainability of intensively managed pastures. Fertilidade Do Solo Para Pastagens Produtivas. Piracicaba, Sao Paulo: Luiz de Queiroz Agricultural Foundation; 2004. pp. 357-400.
13. Dubeux JCB, Sollenberger LE, Mathews BW, Scholberg JM, Santos HQ. Nutrient Cycling in Warm-Climate Grasslands. Crop Sci 2007;47:915-28.
14. Dubeux JCB, Sollenberger LE, Interrante SM, Vendramini JMB, Stewart RL. Litter decomposition and mineralization in bahiagrass pastures managed at different intensities. Crop Sci 2006;46:1305-10.
15. Haynes R, Williams P. Nutrient cycling and soil fertility in the grazed pasture ecosystem; 1993.
16. Dubeux JC, Sollenberger LE. Nutrient cycling in grazed pastures. In Management strategies for sustainable cattle production in southern pastures. Amsterdam: Elsevier; 2020. pp. 59-75.
17. Hristov AN, Oh J, Firkins JL, et al. Special topics-mitigation of methane and nitrous oxide emissions from animal operations: I. A review of enteric methane mitigation options. J Anim Sci 2013;91:5045-69.
18. Cardoso AS, Berndt A, Leytem A, et al. Impact of the intensification of beef production in Brazil on greenhouse gas emissions and land use. Agric Syst 2016;143:86-96.
19. Yu L, Huang Y, Zhang W, Li T, Sun W. Methane uptake in global forest and grassland soils from 1981 to 2010. Sci Total Environ 2017;607-608:1163-72.
20. Samuelson LJ, Stokes TA, Butnor JR, et al. Ecosystem carbon density and allocation across a chronosequence of longleaf pine forests. Ecol Appl 2017;27:244-59.
21. Bracho R, Starr G, Gholz HL, Martin TA, Cropper WP, Loescher HW. Controls on carbon dynamics by ecosystem structure and climate for southeastern U.S. slash pine plantations. Ecol Monogr 2012;82:101-28.
22. Vogel JG, Bracho R, Akers M, et al. Regional assessment of carbon pool response to intensive silvicultural practices in loblolly pine plantations. Forests 2022;13:36.
23. Bracho R, Silveira ML, Boughton R, et al. Carbon dynamics and soil greenhouse fluxes in a Florida’s native rangeland before and after fire. Agric For Meteorol 2021;311:108682.
24. Xu S, Silveira ML, Inglett KS, Sollenberger LE, Gerber S. Effect of land-use conversion on ecosystem C stock and distribution in subtropical grazing lands. Plant Soil 2016;399:233-45.
25. Poeplau C, Don A. Carbon sequestration in agricultural soils via cultivation of cover crops - A meta-analysis. Agric Ecosyst Environ 2015;200:33-41.
26. Jian J, Du X, Reiter MS, Stewart RD. A meta-analysis of global cropland soil carbon changes due to cover cropping. Soil Biol Biochem 2020;143:107735.
27. Rowntree JE, Stanley PL, Maciel ICF, et al. Ecosystem impacts and productive capacity of a multi-species pastured livestock system. Front Sustain Food Syst 2020;4:544984.
28. Villa JA, Mitsch WJ. Carbon sequestration in different wetland plant communities in the Big Cypress Swamp region of southwest Florida. Int J Biodivers Sci Ecosyst Serv Manag 2015;11:17-28.
29. Xiong X, Grunwald S, Myers DB, Ross CW, Harris WG, Comerford NB. Interaction effects of climate and land use/land cover change on soil organic carbon sequestration. Sci Total Environ 2014;493:974-82.
30. Gomez-casanovas N, Delucia NJ, Delucia EH, et al. Seasonal controls of CO2 and CH4 dynamics in a temporarily flooded subtropical wetland. J Geophys Res Biogeosci 2020:125.
31. Ghosh PK, Mahanta SK. Carbon sequestration in grassland systems. Range Manag Agrofor 2014;35:173-81. Available from: https://publications.rmsi.in/index.php/rma/article/view/382 [Last accessed on 8 Dec 2022]
32. Adewopo J, Silveira M, Xu S, Gerber S, Sollenberger L, Martin T. Management intensification impacts on soil and ecosystem carbon stocks in subtropical grasslands. Soil Sci Soc Am J 2014;78:977-86.
33. Henry DD, Ciriaco FM, Araujo RC, et al. Effects of bismuth subsalicylate and encapsulated calcium-ammonium nitrate on enteric methane production, nutrient digestibility, and liver mineral concentration of beef cattle. J Anim Sci 2020:98.
34. Patra AK. The effect of dietary fats on methane emissions, and its other effects on digestibility, rumen fermentation and lactation performance in cattle: a meta-analysis. Livest Sci 2013;155:244-54.
35. Belanche A, Newbold CJ, Morgavi DP, Bach A, Zweifel B, Yáñez-Ruiz DR. A meta-analysis describing the effects of the essential oils blend agolin ruminant on performance, rumen fermentation and methane emissions in dairy cows. Animals 2020;10:620.
36. Jayanegara A, Leiber F, Kreuzer M. Meta-analysis of the relationship between dietary tannin level and methane formation in ruminants from in vivo and in vitro experiments. J Anim Physiol Anim Nutr 2012;96:365-75.
37. Franzluebbers AJ. Cattle grazing effects on the environment: greenhouse gas emissions and carbon footprint. In Management strategies for sustainable cattle production in southern pastures. Amsterdam: Elsevier; 2019. pp. 11-34.
38. Desjardins R, Worth D, Vergé X, Maxime D, Dyer J, Cerkowniak D. Carbon footprint of beef cattle. Sustainability 2012;4:3279-301.
39. Klopatek SC, Marvinney E, Duarte T, Kendall A, Yang XC, Oltjen JW. Grass-fed
40. Beauchemin K, Janzen H, Little S, Mcallister T, Mcginn S. Mitigation of greenhouse gas emissions from beef production in western Canada - evaluation using farm-based life cycle assessment. Anim Feed Sci Technol 2011;166-167:663-77.
41. Rosenbaum KL, Schoene D, Mekouar A. Climate change and the forest sector. Possible national and subnational legislation. 2004. Available from: https://www.fao.org/documents/card/en/c/287cbda2-a217-4ca4-8aca-a9b8dc29e757/ [Last accessed on 8 Dec 2022].
42. Nielsen AS, Plantinga AJ, Alig RJ. Mitigating climate change through afforestation: New cost estimates for the United States. Resour Energy Econ 2014;36:83-98.
43. Torres CME, Kohmann MM, Fraisse CW. Quantification of greenhouse gas emissions for carbon neutral farming in the Southeastern USA. Agric Syst 2015;137:64-75.
44. Richards KR, Stokes C. A review of forest carbon sequestration cost studies: a dozen years of research. Clim Chang 2004;63:1-48.
45. Duffy C, O’Donoghue C, Ryan M, Styles D, Spillane C. Afforestation: replacing livestock emissions with carbon sequestration. J Environ Manag 2020;264:110523.
46. McCarl BA, Schneider UA. Climate change. greenhouse gas mitigation in U.S. agriculture and forestry. Science 2001;294:2481-2.
47. Lewandrowski J, Kim C, Aillery M. Carbon sequestration through afforestation under uncertainty. For Policy Econ 2014;38:90-6.
48. Nilsson S, Schopfhauser W. The carbon-sequestration potential of a global afforestation program. Clim Chang 1995;30:267-93.
49. Mazzetto AM, Bishop G, Styles D, Arndt C, Brook R, Chadwick D. Comparing the environmental efficiency of milk and beef production through life cycle assessment of interconnected cattle systems. J Clean Prod 2020;277:124108.
50. Mishurov M, Kiely G. Nitrous oxide flux dynamics of grassland undergoing afforestation. Agric Ecosyst Environ 2010;139:59-65.
51. Bren d’Amour C, Reitsma F, Baiocchi G, et al. Future urban land expansion and implications for global croplands. Proc Natl Acad Sci USA 2017;114:8939-44.
52. Zhang C, Tian H, Pan S, et al. Effects of forest regrowth and urbanization on ecosystem carbon storage in a rural–urban gradient in the Southeastern United States. Ecosystems 2008;11:1211-22.
53. Harveson PM, Lopez RR, Collier BA, Silvy NJ. Impacts of urbanization on Florida key deer behavior and population dynamics. Biol Conserv 2007;134:321-31.
54. Boggs JL, Sun G. Urbanization alters watershed hydrology in the piedmont of North Carolina. Ecohydrology 2011;4:256-64.
55. Ruhl JB. Water wars, eastern style: divvying up the apalachicola-chattahoochee-flint river basin. J Contemp Water Res Edu 2005;131:47-54. Available from: http://ssrn.com/abstract=1357762 [Last accessed on 8 Dec 2022]
56. Franzluebbers AJ. Achieving soil organic carbon sequestration with conservation agric syst in the Southeastern United States. Soil Sci Soc Am J 2010;74:347-57.
57. Mcbride WD, Mathews K. United States department of agriculture the diverse structure and organization of U.S. beef cow-calf farms. 2011. Available from: www.ers.usda.gov [Last accessed on 8 Dec 2022].
58. Causarano HJ, Franzluebbers AJ, Reeves DW, Shaw JN. Soil organic carbon sequestration in cotton production systems of the Southeastern United States: a review. J Environ Qual 2006;35:1374-83.
59. Rotz CA, Asem-Hiablie S, Dillon J, Bonifacio H. Cradle-to-farm gate environmental footprints of beef cattle production in Kansas, Oklahoma, and Texas. J Anim Sci 2015;93:2509-19.
60. Bergtold JS, Sailus M, Jackson T. Conservation tillage systems in the southeast: production, profitability and stewardship. Washington DC: USDA; 2020. pp. 19-25. Available from: https://www.sare.org/resources/conservation-tillage-systems-in-the-southeast/ [Last accessed on 8 Dec 2022].
61. Baxter LL, West CP, Brown CP, Green PE. Stocker beef production on low-water-input systems in response to legume inclusion: I. forage and animal responses. Crop Sci 2017;57:2294-302.
62. Santos ERS, Dubeux JCB, Mackowiak C, et al. Sward responses of bahiagrass cultivars under no nitrogen fertilization. Crop Sci 2019;59:2893-902.
63. Jaramillo DM, Dubeux JC, Sollenberger LE, et al. Water footprint, herbage, and livestock responses for nitrogen-fertilized grass and grass-legume grazing systems. Crop Sci 2021;61:3844-58.
64. Rochette P, Janzen HH. Towards a revised coefficient for estimating N2O emissions from legumes. Nutr Cycl Agroecosyst 2005;73:171-9.
65. Virkajärvi P, Maljanen M, Saarijärvi K, Haapala J, Martikainen PJ. N2O emissions from boreal grass and grass - clover pasture soils. Agric Ecosyst Environ 2010;137:59-67.
66. Daryanto S, Fu B, Wang L, Jacinthe P, Zhao W. Quantitative synthesis on the ecosystem services of cover crops. Earth Sci Rev 2018;185:357-73.
67. Nunes MR, van Es HM, Schindelbeck R, Ristow AJ, Ryan M. No-till and cropping system diversification improve soil health and crop yield. Geoderma 2018;328:30-43.
68. Mahboubi AA, Lal R, Faussey NR. Twenty-eight years of tillage effects on two soils in ohio. Soil Sci Soc Am J 1993;57:506-12.
69. Liu S, Wang JJ, Tian Z, Wang X, Harrison S. Ammonia and greenhouse gas emissions from a subtropical wheat field under different nitrogen fertilization strategies. J Environ Sci 2017;57:196-210.
70. Connell JA, Hancock DW, Durham RG, Cabrera ML, Harris GH. Comparison of enhanced-efficiency nitrogen fertilizers for reducing ammonia loss and improving bermudagrass forage production. Crop Sci 2011;51:2237-48.
71. Capper JL. Is the grass always greener? Comparing the environmental impact of conventional, natural and grass-fed beef production systems. Animals 2012;2:127-43.
72. Pelletier N, Pirog R, Rasmussen R. Comparative life cycle environmental impacts of three beef production strategies in the Upper Midwestern United States. Agric Syst 2010;103:380-9.
73. USDA. Livestock, poultry and grain market news. 2022. Available from: https://www.ams.usda.gov/market-news/livestock-poultry-and-grain-other-reports [Last accessed on 8 Dec 2022].
74. Bhandari BD, Gillespie J, Scaglia G, Wang J, Salassi M. Analysis of pasture systems to maximize the profitability and sustainability of grass-fed beef production. J Agric Appl Econ 2015;47:193-212.
75. Asem-hiablie S, Rotz CA, Stout R, Place S. Management characteristics of beef cattle production in the eastern United States. Prof Anim Sci 2018;34:311-25.
76. Rouquette M, Aiken GE. Introduction: management strategies for sustainable cattle production in Southern Pastures. In Management strategies for sustainable cattle production in southern pastures. Amsterdam: Elsevier; 2020. pp. 1-10.
77. Archimède H, Eugène M, Marie Magdeleine C, et al. Comparison of methane production between C3 and C4 grasses and legumes. Anim Feed Sci Technol 2011;166-167:59-64.
78. Garcia-Jimenez LM. Ecosystem services provided by contrasting grazing systems in North Florida. Ph.D Dissertation. Gainesville, FL: University of Florida; 2019.
79. DeRamus HA, Clement TC, Giampola DD, Dickison PC. Methane emissions of beef cattle on forages: efficiency of grazing management systems. J Environ Qual 2003;32:269-77.
80. Thompson L, Rowntree J. Invited Review: Methane sources, quantification, and mitigation in grazing beef systems. Appl Anim Sci 2020;36:556-73.
81. Stackhouse KR, Rotz CA, Oltjen JW, Mitloehner FM. Growth-promoting technologies decrease the carbon footprint, ammonia emissions, and costs of California beef production systems. J Anim Sci 2012;90:4656-65.
82. Moriel P, Cooke RF, Bohnert DW, Vendramini JMB, Arthington JD. Effects of energy supplementation frequency and forage quality on performance, reproductive, and physiological responses of replacement beef heifers 1; 2012.
83. Scaglia G. Supplementation of growing beef heifers with starch or highly digestible fiber supplements. J Agric Sci 2020;12:14.
84. Alvarez-Hess PS, Williams SRO, Jacobs JL, et al. Effect of dietary fat supplementation on methane emissions from dairy cows fed wheat or corn. J Dairy Sci 2019;102:2714-23.
85. Arthington JD, Kalmbacher RS. Effect of early weaning on the performance of three-year-old, first-calf beef heifers and calves reared in the subtropics. In J Anim Sci 2003;81. Available from: https://academic.oup.com/jas/article/81/5/1136/4789994 [Last accessed on 8 Dec 2022]
86. Rae D, Kunkle W, Chenoweth P, Sand R, Tran T. Relationship of parity and body condition score to pregnancy rates in Florida beef cattle. Theriogenology 1993;39:1143-52.
87. Derouen SM, Franke DE. Effects of sire breed, breed type and age and weight at breeding on calving rate and date in beef heifers first exposed at three ages. 1989. Available from: https://academic.oup.com/jas/article-abstract/67/5/1128/4705051 [Last accessed on 8 Dec 2022].
88. Arthington JD, Spears JW, Miller DC. The effect of early weaning on feedlot performance and measures of stress in beef calves 1,2. 2005. Available from: https://academic.oup.com/jas/article/83/4/933/4790800 [Last accessed on 8 Dec 2022].
89. Houghton PL, Lemenager RP, Horstman A, Hendrix KS, Moss GE. Effects of body composition, pre-and postpartum energy level, and early weaning on reproductive performance of beef cows and preweaning calf gain. 1990. Available from: https://academic.oup.com/jas/article-abstract/68/5/1438/4705735 [Last accessed on 8 Dec 2022].
90. Lamb GC, Dahlen CR, Larson JE, Marquezini G, Stevenson JS. Control of the estrous cycle to improve fertility for fixed-time artificial insemination in beef cattle: a review. J Anim Sci 2010;88:E181-92.
91. Binelli M, Bittar J, Gonella A. Analysis of the USDA’s 2017 cow-calf management practices results: part 2 - breeding practices/reproductive technologies. EDIS 2021:6.
92. Beef 2017 beef cow-calf management practices in the United States. 2020. Available from: http://www.aphis.usda.gov/nahms [Last accessed on 8 Dec 2022].
93. Kenny DA, Fitzsimons C, Waters SM, McGee M. Invited review: improving feed efficiency of beef cattle - the current state of the art and future challenges. Animal 2018;12:1815-26.
94. Koch RM, Swiger LA, Chambers D, Gregory KE. Efficiency of feed use in beef cattle. J Anim Sci 1963;22:486-94.
95. Arthur PF, Archer JA, Johnston DJ, et al. Genetic and phenotypic variance and covariance components for feed intake, feed efficiency, and other postweaning traits in Angus cattle 1,2. J Anim Sci 2001;79. Available from: https://academic.oup.com/jas/article/79/11/2805/4645243 [Last accessed on 8 Dec 2022]
96. Nutrient requirements of beef cattle, 8th revised edition. Washington, DC: National Academies Press; 2015.
97. Lamb GC, Maddock T. Feed efficiency in cows. Florida beef cattle short course; 2009. pp. 35-42. Available from: https://animal.ifas.ufl.edu/beef_extension/bcsc/2009/pdf/lamb.pdf [Last accessed on 8 Dec 2022].
98. Basarab JA, Beauchemin KA, Baron VS, et al. Reducing GHG emissions through genetic improvement for feed efficiency: effects on economically important traits and enteric methane production. Animal 2013;7:303-15.
99. Freetly HC, Kuehn LA, Thallman RM, Snelling WM. Heritability and genetic correlations of feed intake, body weight gain, residual gain, and residual feed intake of beef cattle as heifers and cows. J Anim Sci 2020:98.
100. Smith PE, Waters SM, Kenny DA, Kirwan SF, Conroy S, Kelly AK. Effect of divergence in residual methane emissions on feed intake and efficiency, growth and carcass performance, and indices of rumen fermentation and methane emissions in finishing beef cattle. J Anim Sci 2021:99.
Cite This Article

How to Cite
Download Citation
Export Citation File:
Type of Import
Tips on Downloading Citation
Citation Manager File Format
Type of Import
Direct Import: When the Direct Import option is selected (the default state), a dialogue box will give you the option to Save or Open the downloaded citation data. Choosing Open will either launch your citation manager or give you a choice of applications with which to use the metadata. The Save option saves the file locally for later use.
Indirect Import: When the Indirect Import option is selected, the metadata is displayed and may be copied and pasted as needed.
About This Article
Copyright
Data & Comments
Data
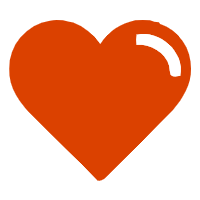
Comments
Comments must be written in English. Spam, offensive content, impersonation, and private information will not be permitted. If any comment is reported and identified as inappropriate content by OAE staff, the comment will be removed without notice. If you have any queries or need any help, please contact us at [email protected].