Omics-based analysis of Akkermansia muciniphila cultivation in food-grade media
Abstract
Background and Aim: Over the past years, the gut microbiota and its correlation to health and disease has been studied extensively. In terms of beneficial microbes, an increased interest in Akkermansia muciniphila
Methods: The bioreactors were supplemented with varying carbon sources, including different ratios of
Results: Comparisons between growth on these media and that on mucin revealed differences at both transcriptome and proteome levels, including differences in the expression of glycosyltransferases, signaling proteins, and stress response. Furthermore, elongated cells and higher OD600 values were observed using the plant-based media as compared to cultivation media containing mucin.
Conclusion: These differences do not hamper growth, and therefore, our data suggest that the food-grade medium composition described here could be used to produce A. muciniphila with high yields for therapeutic purposes.
Keywords
INTRODUCTION
Over the past years, the gut microbiota and its correlation to health and disease have been studied extensively[1]. Notably, strong correlations have been made between the gut microbiota composition and diseases, such as obesity[2,3], pre-diabetes[4,5], type 2 diabetes, non-alcoholic fatty liver disease[6] and liver cirrhosis[7]. Moreover, a causal involvement of gut microbiota by fecal microbiota transplantation has been demonstrated in various inflammatory and metabolic diseases[8]. This is increasing the interest in the development of interventions aiming to alter the gut microbiota, including those with specific gut bacteria, also termed next-generation beneficial microbes[9-11].
While most gut bacteria inhabit the lumen of the colon and thrive on dietary leftovers, Akkermansia muciniphila (A. muciniphila) is an abundant gut symbiont feeding on the colonic mucosa[12-14].
Direct evidence for the role of A. muciniphila was provided in a series of mouse models where the administration of its cells was found to prevent diet-induced obesity[18]. This hallmark study was followed by many reports showing the beneficial effects of A. muciniphila administration in a variety of mouse models[19-23]. However, all these studies have been using mucin-based media to cultivate A. muciniphila, providing a potential bias since this animal-derived glycoprotein is not free from remnants of other bacteria. A breakthrough came with the development of metabolic models that showed the dependency of
Several studies have been focusing on the cultivation, storage, and delivery methods of either pasteurized or alive A. muciniphila for therapeutic purposes[26,28-31]. Moreover, the environmental conditions in which
For human interventions or supplementation with A. muciniphila cells, industrial-scale fermentations are needed and thus the used cultivation media should be not only free from mucin derived from animals but also food-grade, non-allergenic and enable efficient growth to high densities to provide cost-effective production platforms. Moreover, to address present consumer needs, such as increasing interest in flexitarian diets and sustainability, plant-based rather than animal-derived components are to be used. Finally, in these conditions, there should be sufficient production of signaling molecules that have been identified as interacting with the host. It is furthermore of importance to assess the safety of A. muciniphila in the development trajectory for its use in therapeutic applications. A recent study demonstrated the safety of pasteurized A. muciniphila cells in a variety of in vitro models and a 90-day rat trial[40]. This and other information was used by the European Food Safety Authority (EFSA) to approve the use of pasteurized
METHODS
Bacterial strain and culture conditions
The type-strain A. muciniphila MucT (ATCC BAA-835) was used for all cultivation experiments.
Basal medium was used in the fermentations for the initial experiments including soy medium and mucin medium and pre-cultures for the pea peptone bioreactors as described previously[12,43].
For the initial experiments, soy medium and mucin medium were prepared. To prepare soy medium, 16 g/L soy peptone (AM41, Organotechnie SAS) was added to basal medium. In addition, GlcNAc and glucose were added in equimolar amounts to a total of 25 mM (Sigma-Aldrich). Mucin medium was prepared by adding 0.5% hog gastric mucin (Sigma-Aldrich) to basal medium.
Pre-cultures grown for the anaerobic fermentations supplemented with pea peptone were cultivated in basal medium supplemented with tryptone (20 g/L) and L-threonine (4 g/L) with the following carbon source composition: 12.5 mM GlcNAc and 12.5 mM glucose. The cultures were grown in anaerobic conditions and incubated at 37 °C for 48 h (non-shaking).
Food-grade medium was used for the main experiments with the following composition: KH2PO4 (0.4 g/L), Na2HPO4 (0.669 g/L), NH4Cl (0.3 g/L), NaCl (0.3 g/L), MgCl2 6H2O (0.1 g/L), pea peptone A482 (OrganoTechnie SAS, 32 g/L), and L-threonine (4 g/L). After autoclaving 2 mL of reducing solution containing NaHCO3 (40 g/L) and L-cysteine*HCl (5 g/L) and 1% (v/v) of vitamin solution (see above) were added to the medium. The medium was inoculated with 1% (v/v) of the pre-culture. Anaerobic fermentations using this medium were performed as described in the next section.
Anaerobic fermentation
The fermentations were conducted in four parallel bioreactors (DasGip, Eppendorf, Germany) using
HPLC
Samples were obtained at different time points during the fermentation period for the analysis of fermentation products. Crotonate was used as the internal standard. The external standards were GlcNAc, glucose, acetate, propionate, succinate, lactate, and 1,2-propanediol. The substrates and fermentation products were measured using a Shimadzu LC_2030C equipped with a refractive index detector and a Shodex SH1011 column. Two runs were performed for each sample, employing oven temperatures of 45 and 75 °C, with pump flow rates of 1.0 and 0.9 mL/min, respectively. For both runs, 0.01N H2SO4 was used as eluent. All samples and standards (10 µL) ran for 15 min. The concentrations of the standards were ranging between 2.5 and 60 mM. Lastly, the fermentation profiles obtained with HPLC were used to calculate the carbon and energy balances at the endpoint of all fermentations.
RNA isolation and transcriptome analysis
RNA isolation was performed as described previously[44]. Further processing of the total RNA was performed by Novogene (Cambridge, United Kingdom) and paired-end sequences of 150 bp were obtained using an Illumina platform. Transcriptome analysis has been performed as previously described[33]. All further analysis was done using R version 3.6.3 in Rstudio version 1.2.5019.
RESULTS
Growth characteristics and metabolic activity
In the first series of experiments, we built on the metabolic modeling data that predicted A. muciniphila MucT to grow efficiently (growth rate of 0.13 h-1) on an equimolar mixture of glucose and GlcNAc in a minimal medium with threonine[24,25]. To increase cell yield, a food-grade and plant-based protein source was added to the minimal medium in the form of 16 g/L soy protein hydrolysate that resulted in a medium (soy medium) yielding a high growth rate of 0.53 h-1 exceeding that of A. muciniphila on mucin, which is approximately 0.41 h-1[12,35]. The cell densities in the soy peptone medium, as measured by absorption at OD600, were above 5, whereas the mucin medium supported growth to an OD of 2-2.5[24]. Even higher cell yields could be obtained by using pea peptone at a level of 32 g/L, which led to high densities of OD600 values above 10. Since the soy protein hydrolysate is derived from a plant source, it is an acceptable food-grade nitrogen source and applicable on a large scale. Moreover, as the growth rate on soy medium was higher than that on mucin and relatively high cell densities were obtained, we decided to further characterize A. muciniphila cells grown on this food-grade medium, the more so as these were highly active in protecting mice from diet-induced obesity[26]. A few observations were noted in the first series of experiments that needed to be further addressed. First, phase-contrast and scanning electron microscopy of cells grown on soy medium showed a significantly (P-value < 0.01) elongated shape with a length of 1.3
To further address the global differences between A. muciniphila cells grown in soy medium and mucin medium, transcriptome analysis was performed to reveal initial transcriptional changes between mucin and soy medium (Supplementary Table 1 and see below).
The main differences were found to be in the increased expression in soy medium of genes encoding transporters such as Major Facilitator Superfamily (MFS), biopolymer, anion and amino acid transporters (Amuc_1331, Amuc_0546, Amuc_0221 and Amuc_0037), as well as peptide, aliphatic sulfonate, nitrate/sulfonate/bicarbonate, cobalt and manganese ABC transporters (Amuc_0672, AMUC_1297, Amuc_0408, Amuc_1198, Amuc_1199, Amuc_0056, Amuc_1380 and Amuc_1186) with an increase > 5-fold. In addition, genes involved in oxygen stress response were found to be higher in soy medium including rubrerythrin (Amuc_2055 and Amuc_2056), peroxidase (Amuc_1321), and catalase (Amuc_2070). In mucin medium, genes involved in cell shape (Amuc_0540) and division (Amuc_0348) and mucin degradation genes such as alpha-N-acetylglucosaminidase (Amuc_0060), beta-glucanase (Amuc_0875), and sulfatases (Amuc_0491 and Amuc_0451) were found to have an > 5-fold increase.
High biomass yield reached on food-grade medium
Because of the apparent effect of equimolar amounts of glucose and GlcNAc on the morphology, viscosity, and gene expression of A. muciniphila cells, we further explored the effect of different carbon source ratios on the growth and physiology of A. muciniphila. For this purpose, we decided to use pea peptone as an additional food-grade nitrogen source rather than soy peptone to avoid potential issues associated with phytoestrogens present in soy. A total of four fermentations were characterized in detail, with three different glucose to GlcNAc carbon source ratios 3:1 (Condition A), 10:1 (Condition B), and 20:1 (Condition C) and one control, which was supplemented with mucin (Condition D). Interestingly, while the mucin medium enabled a rapid initiation of growth, an increase in the duration of the lag phase was observed along with the decreasing concentrations of GlcNAc in the bioreactors [Figure 1]. It is important to note that the pre-cultures were grown on pea peptone medium supplemented with equimolar concentrations of glucose and GlcNAc, which is most similar to condition A in terms of glucose to GlcNAc ratio. However, up to four transfers in food-grade medium supplemented with glucose and GlcNAc in a ratio of 20:1 were found to lead to growth adaptation of A. muciniphila and rapid initiation of growth [Supplementary Figure 2]. Microscopy results showed the formation of elongated cells in conditions A-C as compared to mucin (condition D) [Supplementary Figure 3]. The number of cells and increased cell length observed in these cultures reflect a higher biomass production. Condition A was found to have the fastest growth and highest biomass density, as deduced from the OD600 measurements.
Figure 1. Exponential growth of A. muciniphila as a function of glucose to GlcNAc ratios. Bioreactors at pH 7.0 were run with glucose to GlcNAc carbon source ratios of 3:1 (Condition A), 10:1 (Condition B), and 20:1 (Condition C) or on mucin (Condition D). Purple arrows indicate mid-log sampling, turquoise arrows indicate end-of-growth sampling, and the brown arrow indicates sampling of condition D. The growth rate is indicated in the graph for conditions A-C. Asterisks at the arrows indicate either glucose and GlcNAc were depleted (**) or only GlcNAc was depleted (*). Observed viscosity in the cultures is indicated with (++) meaning high viscosity, (+) meaning medium viscosity, or (-) no viscosity observed. A. muciniphila: Akkermansia muciniphila; GlcNAc: N-acetylglucosamine.
Transcriptome response in exponential and stationary phase
The growth curve of condition A showed two different growing phases, the first one until approximately
Figure 2. Volcano plot comparing the transcriptional response of A. muciniphila in fermentations of the mid-log phase and end-growth phase of condition A. Only the differentially expressed genes mentioned in the text are labeled here. The complete overview of differentially expressed genes and their annotation can be found in Supplementary File 1. A. muciniphila: Akkermansia muciniphila.
In addition to the transcriptional activity, the catabolism of A. muciniphila in the food-grade medium was assessed to determine the depletion of the glucose and GlcNAc [Figure 1 and Supplementary Figure 7]. In all conditions, the main fermentation products included acetate, propionate, and succinate. As shown previously, the propionate:acetate ratio may differ depending on the cultivation conditions used to grow A. muciniphila[25]. In the conditions tested in this study, the ratio of propionate:acetate varied between the different carbon source ratios used for cultivation [Supplementary Figure 8]. Comparing the final stages of the bioreactors, the lowest propionate:acetate ratio was found in condition D with a ratio of 0.88, followed by condition A with a ratio of 1.06, while the ratios in condition B and C were 1.25 and 1.23, respectively, coinciding with the ratios found in the initial experiments on soy medium. The carbon balances of the fermentations with glucose and various amounts of GlcNAc were calculated and amounted to approximately 72% [Supplementary Figure 9].
GlcNAc concentration affects the expression of glycosyltransferases and stress response genes
A limited number of genes were found to be differentially expressed between the mid-log phases of conditions with different GlcNAc concentrations. Due to the small number of differentially expressed genes between the mid-log phases of conditions B and C (two significant differentially expressed genes, Amuc_1139 and Amuc_1140, both of which belong to a glycosyltransferase cluster), here we only compare condition A (high GlcNAc) and condition C (low GlcNAc) (252 differentially expressed genes) [Figure 3].
Figure 3. Volcano plot comparing the transcriptional response of A. muciniphila in fermentations containing a high concentration of GlcNAc (mid-log condition A) and a low concentration of GlcNAc (mid-log condition C). The differentially expressed genes mentioned in the text are labeled here, as well as highly differentiated genes annotated as hypothetical proteins in italics. The complete overview of differentially expressed genes and their annotation can be found in Supplementary File 1. A. muciniphila: Akkermansia muciniphila; GlcNAc: N-acetylglucosamine.
A higher concentration of GlcNAc showed significantly higher expression of a glycosyltransferase cluster (Amuc_1139 until Amuc_1142) and genes possibly involved in exopolysaccharide or capsular polysaccharide production (Amuc_2077 until Amuc_2079). Furthermore, a higher concentration of GlcNAc led to overexpression of genes involved in stress response, including an anaerobic ribonucleoside triphosphate reductase cluster (Amuc_0860 until Amuc_0862), NAD(P)-dependent oxidoreductase (Amuc_0777), rubrerythrin (Amuc_2056), catalase (Amuc_2070), glutamate decarboxylase (Amuc_0372), and molecular chaperones DnaK (Amuc_1406), HtpG (Amuc_2002), and GroES (Amuc_1407). In low GlcNAc, many genes encoding hypothetical proteins were found among the upregulated genes. However, lactoylglutathione lyase (Amuc_1878), glutamate dehydrogenase (Amuc_2051), and phosphate ABC transporter permease protein PstA (Amuc_1304) were significantly upregulated in this condition as compared to high GlcNAc.
Proteomic and transcriptomic response of A. muciniphila in glucose/GlcNAc vs. mucin
Cultivating A. muciniphila in food-grade medium where mucin is substituted for a mixture of glucose and GlcNAc may induce transcriptional and translational changes that affect its physiology. Using the proteome data, we compared mid-log condition A (high GlcNAc) and condition D (mucin). In total, 116 proteins were identified to have a protein abundance ratio higher than 10. The protein abundance ratios indicate that in the mucin condition, most proteins involved in mucin degradation were upregulated [Supplementary Table 3]. In contrast, in high GlcNAc medium, the proteins that were upregulated in comparison to mucin were mainly stress-related proteins and glycosyltransferases, in line with the observations related to the transcriptional response.
In the following sections, we focus mainly on the transcriptome data since these revealed a higher number of differentially expressed genes than that found in the proteomics data [Supplementary Table 2]. This suggests that cells responded to differences in medium composition by adapting its regulations over its functional aspect, possibly minimizing differences in overall cell composition. In the following sections, we compared the transcriptional response of exponentially growing cells (mid-log) on high GlcNAc or low GlcNAc and mucin.
High GlcNAc induces stress response as compared to mucin
In the medium containing high GlcNAc (Condition A), several genes and complete gene clusters related to stress responses were found to be significantly upregulated as compared to the mucin condition [Figure 4]. This includes a gene cluster encoding for an ABC transporter and phosphate ABC transporter (Amuc_1294-1308), a gene cluster involved in the production of exopolysaccharides (Amuc_2077-Amuc_2096), and an additional significantly upregulated glycosyltransferase cluster (Amuc_1139-1142), a gene cluster containing multiple aldo/keto reductases (Amuc_1796-1809), and the gene for anaerobic ribonucleoside-triphosphate reductase activating protein (Amuc_0860). Moreover, additional complete gene clusters related to stress responses were found to be significantly upregulated in both high-GlcNAc and low-GlcNAc conditions, as compared to mucin, including a gene cluster encoding ribosomal proteins (Amuc_0294-0308), an iron transport cluster (Amuc_1930 until Amuc_1934), and a potential flavin biosynthesis gene cluster (Amuc_0421-0426) [Supplementary File 1]. Other genes that may be involved in stress response but not part of a gene cluster were also identified to be upregulated in both GlcNAc conditions. This includes genes for rubrerythrin (Amuc_2055-2056), catalase (Amuc_2070), oxidoreductases (Amuc_0116, Amuc_0777, Amuc_1072, Amuc_1176 and Amuc_1389), ribonucleoside-triphosphate reductase (Amuc_0862), and glutamate decarboxylase (Amuc_0372).
Figure 4. Volcano plot comparing the transcriptional response of A. muciniphila in fermentations containing a high concentration of GlcNAc (mid-log condition A) and mucin (condition D). Due to the high number of genes upregulated in high-GlcNAc, genes with a
Next to the transcriptomic stress response, elongated cells were observed in the bioreactors containing glucose and GlcNAc in comparison to the small oval-shaped cells visible when A. muciniphila was cultivated on mucin [Supplementary Figure 3]. Elongated cells were also observed in the previously mentioned soy medium cultures [Supplementary Figure 1], together with an increased expression of Amuc_0540 encoding cell shape-determining protein MreB in mucin as compared to soy medium. Following this observation, we performed a more in-depth analysis of the expression of genes involved in cell elongation and division [Table 1 and Supplementary File 1]. Overall, the transcriptomic stress response and elongated cells observed in the cultures grown in synthetic medium without mucin indicate that a stress response is triggered in the absence of mucin in this medium.
Differential expression of genes involved in cell division comparing high-GlcNAc to the mucin condition
Genes | Description | High-GlcNAc vs. mucin | ||
Fold change | P value | |||
Amuc_1176 | Cell division inhibitor | 2.62 | 6.57E-48 | Upregulated in high GlcNAc |
Amuc_0348 | Cell division protein FtsH | 1.50 | 4.32E-36 | |
Amuc_1052 | Cell division trigger factor | 1.43 | 7.41E-31 | |
Amuc_1558 | RIP metalloprotease RseP | 0.22 | 1.63E-06 | |
Amuc_0662 | Polypeptide-transport-associated domain-containing protein FtsQ-type | 0.31 | 4.58E-08 | Upregulated in mucin |
Amuc_0540 | Cell shape-determining protein MreB* | 0.45 | 6.6E-11 | |
Amuc_0652 | Peptidoglycan glycosyltransferase | 0.59 | 4.79E-19 | |
Amuc_0649 | Transcriptional regulator MraZ | 0.62 | 3.34E-17 | |
Amuc_0153 | Cell division protein FtsA | 0.64 | 1.97E-18 | |
Amuc_0152 | Tubulin/FtsZ GTPase | 0.88 | 1.24E-26 | |
Amuc_2076 | Cell division FtsK | 1.16 | 3.66E-34 | |
Amuc_0658 | Cell cycle protein | 1.50 | 3.38E-41 | |
Amuc_0514 | Peptidoglycan glycosyltransferase | 1.67 | 1.26E-41 | |
Amuc_1317 | Integral membrane protein CcmA involved in cell shape determination | 2.03 | 2.88E-46 |
DISCUSSION
In this study, we assessed the cultivation of A. muciniphila food-grade pea-peptone medium with different concentrations of carbon sources to produce cells suitable for therapeutic applications. The use of food-grade synthetic and non-allergenic medium supplemented with glucose and GlcNAc resulted in high cell yields and fast growth of A. muciniphila. Furthermore, we gained detailed insight into physiology by a combination of biochemical analysis as well as transcriptional and proteomic analysis. In addition, we compared the use of the food-grade synthetic medium to the mucin medium, which has been used in many studies to grow A. muciniphila cells for animal studies[18-23].
The highest growth rate and final optical density were reached in food-grade medium containing the highest concentration of GlcNAc. In the GlcNAc conditions, the cells were observed to be elongated, possibly affecting the optical density in these cultivations. Furthermore, PCA analysis showed that in both the proteome and transcriptome data, the mucin condition clusters separately from the conditions containing GlcNAc, whereas in the transcriptome data alone, the end-growth phase of condition A also clusters separately from the other conditions and time points. However, the KEGG metabolism on general level 1 did not reveal significant differences between fermentor conditions within the different metabolisms. It may be possible that the regulation of several genes evened out the impact and differences would be visible at a deeper level. Assessing the transcriptome and proteome data in more detail revealed the upregulation of proteins and genes involved in stress response in GlcNAc conditions and the pili-associated system, mucin degradation, and protein sorting systems in mucin conditions.
A shift in propionate to acetate production was observed between the condition containing mucin and the condition containing glucose and GlcNAc, as well as between the different glucose and GlcNAc conditions. At the end of the fermentation in mucin, a propionate:acetate ratio of 0.88 was observed. However, with the decreasing concentration of GlcNAc in the other conditions, the ratio shifted toward more propionate production. In condition A, the ratio was 1:1, which was similar to previous findings, where a 50:50 ratio of glucose and GlcNAc was used as a carbon source[25]. The shift toward a higher propionate:acetate ratio in conditions B and C is in line with the degradation reactions that were predicted using the genome-scale model of A. muciniphila[24]. Therefore, it is important to note that using a lower GlcNAc concentration in the cultivation of A. muciniphila causes a shift in the propionate:acetate ratio, resulting in an altered short-chain fatty acid profile.
The carbon recovery values indicated a gap between the carbon sources that were consumed and the energy and carbon sources that were produced. The carbon recovery values ranged between 70%-73%, excluding biomass and amino acid formation. Previously, a carbon recovery of 80%-90% has been described for
Cell elongation was observed in the fermentations without mucin. As described previously, the cells of
The protein Amuc_1100 is gaining increasing interest in several studies for its positive effect on host health[35,47]. The gene cluster associated with pili production, including Amuc_1100, showed significant upregulation in the condition supplemented with mucin compared to the GlcNAc conditions. The proteome data support this observation, but the protein abundance ratio observed was less than 10. However, as the proteome data sets relate to relative amounts of proteins, absolute amounts of proteins could be higher in the GlcNAc conditions as the OD600 value was approximately 8-fold higher in the high-GlcNAc condition than the mucus condition.
The upregulation of multiple stress-related genes and gene clusters was identified in fermentations on GlcNAc compared to mucin. First, the upregulation of the phosphate ABC transporter system may indicate a phosphate limitation in these cultures[48]. To overcome this limitation, additional phosphate sources could be added to the food-grade medium. Furthermore, several genes previously found to be involved in the oxygen stress response of A. muciniphila were found to be upregulated in conditions without mucin as well[32]. However, these fermentations were run anaerobically simultaneously, as was the case with the mucin condition. Therefore, it is likely that this stress response was not specific for oxygen, but rather a form of cross-protection against other stresses[49]. Another stress response that was activated in conditions containing glucose and GlcNAc was the production of EPS (Amuc_2077-2096). Interestingly, the expression of the genes involved in EPS production decreased along with the decreasing concentration of GlcNAc, as well as the glycosyltransferase cluster (Amuc_1139 until Amuc_1142). The production of EPS in bacteria is often a mechanism to cope with harsh environmental conditions, as extensively studied for lactic acid bacteria[50]. For A. muciniphila, the high-GlcNAc condition, with limiting medium components, may be sub-optimal. Additionally, within condition A, it was visible that during the mid-growth phase, stress-related genes were upregulated as compared to the end phase; this included metabolic gene cluster encoding a glutaminase and a likely glutamine-GABA antiporter (Amuc_0037-0038), as well as several stress proteins (Amuc_1406-1408, coding for DnaK, GroES and GroEL). However, the upregulation of stress-related genes does not limit growth rate and biomass production as observed in these fermentations. We showed that pea peptone-based food-grade medium supplemented with glucose and GlcNAc instead of mucin results in high biomass formation of A. muciniphila, which may be used for its production for therapeutic purposes.
Other comparisons of the growth of A. muciniphila on mucin versus specific carbon sources have been made[24,25]. A transcriptome comparison was conducted between cultivations supplemented with mucin and those supplemented with glucose[24]. Despite the differences in cultivation, cultures with glucose as a carbon source also showed upregulation of stress-related genes in comparison to mucin[24]. When comparing the data of the previous study[24] and the present one, we noted that the expression of many genes was similar. Furthermore, similar differences were also documented for genes and proteins involved in mucin degradation in both studies. In the present proteome analysis, we found that on the protein level, it was clearly visible that the mucin culture had an upregulation of proteins involved in mucin degradation, since these proteins were located in the top protein abundance ratios. Similar findings were documented previously[24]. In contrast, there were also differences between the previous study[24] and the present one, notably including differentially expressed stress-related genes. Moreover, the differences noted here in the expression of genes involved in cell division were not found in the earlier study. This may be due to the low growth rates and final biomass that were observed in the cultures with mucin only, while in our studies with synthetic media, high growth rates and biomass were observed.
In conclusion, we showed that pea peptone-based food-grade medium supplemented with glucose and GlcNAc instead of mucin results in high biomass formation of A. muciniphila, which may be used for its production for therapeutic purposes. The differences between growth on mucin and growth on glucose and GlcNAc were shown at transcriptome and proteome levels. However, the general level 1 sKEGG metabolism pathways showed high similarity between conditions and no major significant differences could be identified. Furthermore, if minimal EPS production is required, the transcriptome data indicate that this may be achieved by decreasing the GlcNAc concentration. Lastly, the use of synthetic medium affects the cell morphology of A. muciniphila, resulting in elongated cells. Overall, our data suggest that the food-grade synthetic medium composition described here could be used to produce A. muciniphila in high yields for therapeutic purposes. This has recently been shown in the first proof-of-concept study with A.muciniphila MucT grown on such a synthetic medium that showed the capacity of live and pasteurized cells to improve several metabolic parameters in overweight and obese volunteers[24].
DECLARATIONS
Acknowledgments
We are grateful to Dr Bart Smit (NIZO) for a gift of industrial peptones and Dr Martin Pabst for giving input in proteomics analysis. The content of the manuscript is derived from the dissertation.
Authors’ contributions
Designed experiments, conducted wet lab activities, analyzed data, constructed figures, and wrote the manuscript: Geerlings SY
Conducted initial experiments and wrote the manuscript: van der Ark K
Processed raw data for transcriptomics and proteomics: Nijsse B, Boeren S
Provided input in result interpretation, guided the writing of the manuscript, as well as provided input and critically reviewed the manuscript: van Loosdrecht M, Belzer C, de Vos WM
Availability of data and materials
Data supporting our findings are published as Supplementary Materials in this journal.
Financial support and sponsorship
This research was supported by the Netherlands Organization for Scientific Research (SIAM Gravity Grant 024.002.002).
Conflicts of interest
de Vos WM and Belzer C are inventors on patents related to the growth of Akkermansia spp. de Vos WM is a co-founder and shareholder of the Akkermansia Company, commercializing pasteurized Akkermansia muciniphila, while the other authors have declared that they have no conflicts of interest.
Ethical approval and consent to participate
Not applicable.
Consent for publication
Not applicable.
Copyright
© The Author(s) 2024.
Supplementary Materials
REFERENCES
1. de Vos WM, Tilg H, Van Hul M, Cani PD. Gut microbiome and health: mechanistic insights. Gut 2022;71:1020-32.
2. Tims S, Derom C, Jonkers DM, et al. Microbiota conservation and BMI signatures in adult monozygotic twins. ISME J 2013;7:707-17.
3. Le Chatelier E, Nielsen T, Qin J, et al; MetaHIT consortium. Richness of human gut microbiome correlates with metabolic markers. Nature 2013;500:541-6.
4. Allin KH, Tremaroli V, Caesar R, et al; IMI-DIRECT consortium. Aberrant intestinal microbiota in individuals with prediabetes. Diabetologia 2018;61:810-20.
5. Zhong H, Ren H, Lu Y, et al. Distinct gut metagenomics and metaproteomics signatures in prediabetics and treatment-naïve type 2 diabetics. EBioMedicine 2019;47:373-83.
6. Jiang W, Wu N, Wang X, et al. Dysbiosis gut microbiota associated with inflammation and impaired mucosal immune function in intestine of humans with non-alcoholic fatty liver disease. Sci Rep 2015;5:8096.
7. Qin N, Yang F, Li A, et al. Alterations of the human gut microbiome in liver cirrhosis. Nature 2014;513:59-64.
8. Hanssen NMJ, de Vos WM, Nieuwdorp M. Fecal microbiota transplantation in human metabolic diseases: from a murky past to a bright future? Cell Metab 2021;33:1098-110.
9. Fan Y, Pedersen O. Gut microbiota in human metabolic health and disease. Nat Rev Microbiol 2021;19:55-71.
10. Cani PD, de Vos WM. Next-generation beneficial microbes: the case of Akkermansia muciniphila. Front Microbiol 2017;8:1765.
11. Bui TPN, de Vos WM. Next-generation therapeutic bacteria for treatment of obesity, diabetes, and other endocrine diseases. Best Pract Res Clin Endocrinol Metab 2021;35:101504.
12. Derrien M, Vaughan EE, Plugge CM, de Vos WM. Akkermansia muciniphila gen. nov., sp. nov., a human intestinal mucin-degrading bacterium. Int J Syst Evol Microbiol 2004;54:1469-76.
13. Geerlings SY, Kostopoulos I, de Vos WM, Belzer C. Akkermansia muciniphila in the human gastrointestinal tract: when, where, and how? Microorganisms 2018;6:75.
14. Cani PD, Depommier C, Derrien M, Everard A, de Vos WM. Author Correction: Akkermansia muciniphila: paradigm for next-generation beneficial microorganisms. Nat Rev Gastroenterol Hepatol 2022;19:682.
15. Belzer C, de Vos WM. Microbes inside - from diversity to function: the case of Akkermansia. ISME J 2012;6:1449-58.
16. Dao MC, Everard A, Aron-Wisnewsky J, et al; MICRO-Obes Consortium. Akkermansia muciniphila and improved metabolic health during a dietary intervention in obesity: relationship with gut microbiome richness and ecology. Gut 2016;65:426-36.
17. Karcher N, Nigro E, Punčochář M, et al. Genomic diversity and ecology of human-associated Akkermansia species in the gut microbiome revealed by extensive metagenomic assembly. Genome Biol 2021;22:209.
18. Everard A, Belzer C, Geurts L, et al. Cross-talk between Akkermansia muciniphila and intestinal epithelium controls diet-induced obesity. Proc Natl Acad Sci U S A 2013;110:9066-71.
19. Wang F, Cai K, Xiao Q, He L, Xie L, Liu Z. Akkermansia muciniphila administration exacerbated the development of colitis-associated colorectal cancer in mice. J Cancer 2022;13:124-33.
20. Qu S, Fan L, Qi Y, et al. Akkermansia muciniphila alleviates dextran sulfate sodium (DSS)-induced acute colitis by NLRP3 activation. Microbiol Spectr 2021;9:e0073021.
21. Bian X, Wu W, Yang L, et al. Administration of Akkermansia muciniphila ameliorates dextran sulfate sodium-induced ulcerative colitis in mice. Front Microbiol 2019;10:2259.
22. Yaghoubfar R, Behrouzi A, Ashrafian F, et al. Modulation of serotonin signaling/metabolism by Akkermansia muciniphila and its extracellular vesicles through the gut-brain axis in mice. Sci Rep 2020;10:22119.
23. Wu F, Guo X, Zhang M, et al. An Akkermansia muciniphila subtype alleviates high-fat diet-induced metabolic disorders and inhibits the neurodegenerative process in mice. Anaerobe 2020;61:102138.
24. Ottman N, Davids M, Suarez-Diez M, et al. Genome-scale model and omics analysis of metabolic capacities of Akkermansia muciniphila reveal a preferential mucin-degrading Lifestyle. Appl Environ Microbiol 2017;83:e01014-17.
25. van der Ark KCH, Aalvink S, Suarez-Diez M, Schaap PJ, de Vos WM, Belzer C. Model-driven design of a minimal medium for Akkermansia muciniphila confirms mucus adaptation. Microb Biotechnol 2018;11:476-85.
26. Plovier H, Everard A, Druart C, et al. A purified membrane protein from Akkermansia muciniphila or the pasteurized bacterium improves metabolism in obese and diabetic mice. Nat Med 2017;23:107-13.
27. Depommier C, Everard A, Druart C, et al. Supplementation with Akkermansia muciniphila in overweight and obese human volunteers: a proof-of-concept exploratory study. Nat Med 2019;25:1096-103.
28. van der Ark KCH. Metabolic characterization and viable delivery of Akkermansia muciniphila for its future application. 2018. Available from: https://www.proquest.com/openview/d51699682401c1858ddc78c47dba7bfd/1?pq-origsite=gscholar&cbl=2026366&diss=y. [Last accessed on 13 Jun 2024].
29. Chang Y, Yang Y, Xu N, Mu H, Zhang H, Duan J. Improved viability of Akkermansia muciniphila by encapsulation in spray dried succinate-grafted alginate doped with epigallocatechin-3-gallate. Int J Biol Macromol 2020;159:373-82.
30. Marcial-Coba MS, Cieplak T, Cahú TB, Blennow A, Knøchel S, Nielsen DS. Viability of microencapsulated Akkermansia muciniphila and Lactobacillus plantarum during freeze-drying, storage and in vitro simulated upper gastrointestinal tract passage. Food Funct 2018;9:5868-79.
31. Marcial-Coba MS, Saaby L, Knøchel S, Nielsen DS. Dark chocolate as a stable carrier of microencapsulated Akkermansia muciniphila and Lactobacillus casei. FEMS Microbiol Lett 2019;366:fny290.
32. Ouwerkerk JP, van der Ark KCH, Davids M, et al. Adaptation of Akkermansia muciniphila to the oxic-anoxic interface of the mucus layer. Appl Environ Microbiol 2016;82:6983-93.
33. Hagi T, Geerlings SY, Nijsse B, Belzer C. The effect of bile acids on the growth and global gene expression profiles in Akkermansia muciniphila. Appl Microbiol Biotechnol 2020;104:10641-53.
34. Ottman N, Huuskonen L, Reunanen J, et al. Characterization of outer membrane proteome of Akkermansia muciniphila reveals sets of novel proteins exposed to the human intestine. Front Microbiol 2016;7:1157.
35. Ottman N, Reunanen J, Meijerink M, et al. Pili-like proteins of Akkermansia muciniphila modulate host immune responses and gut barrier function. PLoS One 2017;12:e0173004.
36. Yoon HS, Cho CH, Yun MS, et al. Akkermansia muciniphila secretes a glucagon-like peptide-1-inducing protein that improves glucose homeostasis and ameliorates metabolic disease in mice. Nat Microbiol 2021;6:563-73.
37. Meng X, Zhang J, Wu H, Yu D, Fang X. Akkermansia muciniphila Aspartic Protease Amuc_1434* inhibits human colorectal cancer LS174T cell viability via TRAIL-mediated apoptosis pathway. Int J Mol Sci 2020;21:3385.
38. Qian K, Chen S, Wang J, Sheng K, Wang Y, Zhang M. A β-N-acetylhexosaminidase Amuc_2109 from Akkermansia muciniphila protects against dextran sulfate sodium-induced colitis in mice by enhancing intestinal barrier and modulating gut microbiota. Food Funct 2022;13:2216-27.
39. Segers A, de Vos WM. Mode of action of Akkermansia muciniphila in the intestinal dialogue: role of extracellular proteins, metabolites and cell envelope components. Microbiome Res Rep 2023;2:6.
40. Druart C, Plovier H, Van Hul M, et al. Toxicological safety evaluation of pasteurized Akkermansia muciniphila. J Appl Toxicol 2021;41:276-90.
41. Turck D, Bohn T, Castenmiller J, et al; EFSA Panel on Nutrition, Novel Foods and Food Allergens (NDA). Safety of pasteurised Akkermansia muciniphila as a novel food pursuant to Regulation (EU) 2015/2283. EFSA J 2021;19:e06780.
42. Geerlings SY. A rising star: a comprehensive approach to Akkermansia muciniphila ecosystems, interactions and applications. 2023. Available from: https://research.wur.nl/en/publications/a-rising-star-a-comprehensive-approach-to-akkermansia-muciniphila. [Last accessed on 13 Jun 2024].
44. Shetty SA, Kostopoulos I, Geerlings SY, Smidt H, de Vos WM, Belzer C. Dynamic metabolic interactions and trophic roles of human gut microbes identified using a minimal microbiome exhibiting ecological properties. ISME J 2022;16:2144-59.
45. Chakraborty S, Gogoi M, Chakravortty D. Lactoylglutathione lyase, a critical enzyme in methylglyoxal detoxification, contributes to survival of Salmonella in the nutrient rich environment. Virulence 2015;6:50-65.
46. Guthrie B, Wickner W. Trigger factor depletion or overproduction causes defective cell division but does not block protein export. J Bacteriol 1990;172:5555-62.
47. Wang J, Xu W, Wang R, Cheng R, Tang Z, Zhang M. The outer membrane protein Amuc_1100 of Akkermansia muciniphila promotes intestinal 5-HT biosynthesis and extracellular availability through TLR2 signalling. Food Funct 2021;12:3597-610.
48. Hudek L, Premachandra D, Webster WA, Bräu L. Role of phosphate transport system component PstB1 in phosphate internalization by Nostoc punctiforme. Appl Environ Microbiol 2016;82:6344-56.
49. den Besten HM, Arvind A, Gaballo HM, Moezelaar R, Zwietering MH, Abee T. Short- and long-term biomarkers for bacterial robustness: a framework for quantifying correlations between cellular indicators and adaptive behavior. PLoS One 2010;5:e13746.
Cite This Article
How to Cite
Download Citation
Export Citation File:
Type of Import
Tips on Downloading Citation
Citation Manager File Format
Type of Import
Direct Import: When the Direct Import option is selected (the default state), a dialogue box will give you the option to Save or Open the downloaded citation data. Choosing Open will either launch your citation manager or give you a choice of applications with which to use the metadata. The Save option saves the file locally for later use.
Indirect Import: When the Indirect Import option is selected, the metadata is displayed and may be copied and pasted as needed.
About This Article
Special Issue
Copyright
Data & Comments
Data
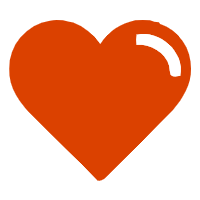
Comments
Comments must be written in English. Spam, offensive content, impersonation, and private information will not be permitted. If any comment is reported and identified as inappropriate content by OAE staff, the comment will be removed without notice. If you have any queries or need any help, please contact us at [email protected].