The untapped potential of cell culture in disentangling insect-microbial relationships
Abstract
Cell culture is a powerful technique for the investigation of molecular mechanisms fundamental to health and disease in a diverse array of organisms. Cell lines offer several advantages, namely their simplistic approach and high degree of reproducibility. One field where cell culture has proven particularly useful is the study of the microbiome, where cell culture has led to the illumination of microbial influences on host immunity, nutrition, and physiology. Thus far, researchers have focused cell culture work predominantly on humans, but the growing field of insect microbiome research stands to benefit greatly from its application. Insects constitute one of Earth’s most diverse and ancient life forms and, just as with humans, possess microbiomes with great significance to their health. Insects, which play critical roles in supporting food security and ecological stability, are facing increasing threats from agricultural intensification, climate change, and pesticide use. As the microbiome is closely tied to host health, gaining a more robust understanding is of increasing importance. In this review, we assert that the cultivation and utilization of insect gut cell lines in microbiome research will bridge critical knowledge gaps essential for informing insect management practices in a world under pressure.
Keywords
INTRODUCTION
Cell culture typically refers to the growth and maintenance of eukaryotic cells outside of their native environment under controlled laboratory conditions[1]. Cultured cells may be derived from humans, insects, other animals, plants, and fungi. By breaking down the organism into discrete parts, cell culture models offer a high-resolution view of the current subject, free of in vivo confounders[1]. As well, the homogeneity of cell culture, with a singular cell type and a well-defined genetic profile, allows for exceptional reproducibility[1]. The application of cell culture has revolutionized in vitro research endeavors, especially in genetics, pathology, and microbiology.
In vivo animal models are a commonly used approach to scientific research, serving as valuable tools for confirming in vitro findings and investigating various biological processes, diseases, and potential treatments. These models allow researchers to study complex physiological and pathological phenomena in a controlled environment. Although insect animal models raise less ethical concerns and debate than vertebrate models, there are several limitations when completing in vivo research. Practical barriers, encompassing the acquisition and maintenance of these insects, can prove daunting for researchers. Such issues include obtaining administrative approval, securing sufficient space and equipment for rearing insects, or possessing the knowledge to supply the environmental conditions and nutritional needs required. Further, in studies that investigate microbe-host tissue interactions, researchers face the intricate task of mitigating confounding factors linked to the host. This involves addressing challenges like immunological response and the potential off-target effects of other microbes within the host. Creating germ-free insect models, a crucial step to limit these confounding factors, is labour-intensive and may induce detrimental changes to the form and function of the host. Another notable concern is the overwintering of insects in temperate or continental climates, which can impact research timelines and reproducibility. Recognizing these challenges, researchers are exploring alternative models, such as cell lines, for preliminary and mechanistic studies within controlled environments, offering a potential workaround to some of the obstacles inherent in using live insect models.
One field where cell culture models have proven to be uniquely powerful is in the study of the microbiome, which is the community of microorganisms (and their genetic potential, metabolites, structural elements, and environmental conditions) living within the intestinal tract of the host[2]. Cell culture of human epithelial cells, particularly of intestinal origin, has improved our understanding of how microbes (or microbial metabolites) impact intestinal transepithelial permeability[3], immune signaling[4], and cellular proliferation mechanisms relevant to cancer[5].
Despite great promise, researchers have not yet adopted this approach concerning the insect microbiome. Similar to the human gut microbiome, the insect gut microbiome serves as a critical intermediary between the environment and host health, aiding in digestion, detoxification, and combating pathogens[6,7], yet it remains poorly understood. Crucial to both ecosystem health and global food security, insects are facing rising threats of habitat destruction, pesticide use, and antimicrobials[8,9]. Given their importance and vulnerability, it is essential to understand the microbes so inextricably tied to their health. In order to study host-gut microbiome interactions in insects, tissue-specific intestinal cell lines must be obtained from insect hosts. Overall, cell lines can be separated into two major categories: primary and immortalized. Primary cell lines are obtained directly from insects and maintained under stringent laboratory conditions for short periods of time before they eventually die out. Immortalized lines are an asset because they allow for laboratory manipulation without the need for available live insect sources. Of the 1,500 immortalized insect cell lines openly available, a mere 0.012% represent midgut tissue[10]. Of this fraction, only 9 of the over
A BRIEF HISTORY OF INSECT CELL CULTURE
The roots of insect research at the cellular level can be traced back to 1912, when Glaser and Chapman examined how wilt disease affected spongy moth (Lymantria dispar) hemocytes - key immune effector cells of invertebrates that participate in phagocytosis, encapsulation, and clotting systems[12]. In the following decades, insect cell culture techniques continued to advance, resulting in the successful establishment of multiple primary cell lines[13]. Continuous cell lines, however, remained elusive during this early work, as cell lines were unable to survive beyond 3 months[13]. This milestone is generally accepted to have been finally achieved by Grace (1962) when he derived a continuous cell line from the ovarian tissue of emperor gum moth (Opodipthera eucalypti) pupae[14]. Since this time, insect cell culture has blossomed along with advancements in aseptic techniques and complex media formulations. A notable cell line, Sf-21, which was produced by Vaughn et al. in 1977, is used in the mass production of baculovirus stocks and recombinant proteins for human vaccine production to date[15,16]. Insect cells have also been used in infectious disease research, pesticide screening, and “bio-bots” (small robots incorporating animal biomass), and have the potential to be used as a food source[13,17,18]. At present, over 1,500 insect cell lines spanning 7 orders of taxonomy are cataloged on Cellosaurus[11], displaying significant progress in the field over 80 years.
THE GROWTH REQUIREMENTS OF INSECT CELL LINES
Insect cell lines exhibit broad adaptability to culture conditions and many lines can be successfully cultivated in temperatures ranging from 22-34 °C, 0%-5% CO2, pH 6.0-6.8, and ambient humidity[15,19]. In contrast, mammalian cells typically require tight adherence to in vivo conditions during incubation, including a consistent temperature of 35-37 °C, 5% CO2, pH 7.0-7.3, and 95% relative humidity
Figure 1. Venn diagram illustrating culture condition requirements of insect and mammalian cell lines. Insect and mammalian cell lines share many nutritional requirements, but differ in their environmental conditions, with insect cell lines notably requiring a lower resource demand. Created with BioRender.com[20].
Several types of media have been developed that satisfy the nutritional requirements and chemical conditions needed by insect cells[15,21]. In general, insect cells require essential amino acids, inorganic salts, sugars, and vitamins[21]. Additionally, media is often supplemented with growth-promoting substances, namely insect hemolymph or fetal bovine serum[21]. These conditions are similar to mammalian cells and are subject to modification depending on the cell line.
The control of pH in insect cell lines is primarily achieved with a phosphate buffer system, which does not require a CO2 incubator to function[15]. Mammalian cells, by contrast, commonly utilize either HEPES [4-(2-hydroxyethyl)-1-piperazineethanesulfonic acid], a CO2-independent buffer system, or a bicarbonate buffer, which relies on regulated CO2 conditions to effectively control pH[22]. HEPES is not usually recommended for insect cell culture; however, some literature has reported its use, namely in AmE-711, a spontaneous cell line derived from embryonic western honey bee (Apis mellifera) tissue[15,19]. Insect cell lines display a markedly elevated rate of oxygen consumption and a decreased rate of lactate production in comparison to mammalian cells. This discrepancy may be exaggerated in cell culture, as a large proportion of mammalian cell lines are derived from cancerous tissue. A well-documented phenomenon, the Warburg effect, is the observation that cancerous tissue exhibits reduced oxygen consumption and heightened lactate production compared to healthy tissue[23,24]. Nonetheless, insect cells’ increased gas exchange (and therefore CO2 accumulation) necessitates the introduction of base to increase pH[15]. The addition of base increases the osmolarity of the media and, in turn, increases the risk of osmotic shock in cell culture[15]. The degree to which low lactate production ameliorates the acidic effect of heightened CO2 production in insect cells is unknown and could be a promising area to explore in future studies.
ZOOMING IN: THE POTENTIAL OF INSECT GUT CELL LINES TO IMPROVE OUR UNDERSTANDING OF THE MICROBIOME
Cell lines have served as valuable tools in the understanding of host-microbe interactions in insects. The predominant focus of these studies, however, has been the investigation of host-pathogen relationships; nominal research has explored the impact of symbionts on the host and no research has studied microbial communities, including those of the gut. In this review, we separate the study of pathogens from the study of the microbiome and emphasize studies using cell lines to understand host-symbiont relationships and the impact of microbial communities on insect health. Studies have yet to explore the use of insect gut cell lines to understand host-microbial relationships, although several studies utilize other tissues for this purpose.
One study that utilized insect cell culture to investigate a symbiont-host interaction in mosquitos (Aedes fluviatilis) observed that cultured embryonic cells infected with the obligate endosymbiont Wolbachia pipientis displayed improved energy performance and innate immune system activation[25]. Other studies have employed cell lines to elucidate the molecular mechanisms of bacterial infiltration into host cells. For example, a study found that an unclassified Rickettsia sp., an endosymbiont of rice green leafhopper
The insect gut microbiome has evolved alongside its host over millions of years, forming an intimate and often life-sustaining bond[29,30]. Microbes provide insect hosts with essential nutrient provisioning, aid in digestion, and confer resistance to pathogens[6,7]. Illustrating this, bacterial strains isolated from the gut of the bark beetle (Dendroctonus rhizophagus) have been shown to hydrolyze pectin, cellulose, xylan, starch, lipids, and esters - thereby allowing the host organism to derive nutrition from otherwise indigestible substrates[31]. By increasing metabolic capacity and diversity, the insect microbiome thus allows the exploitation of a wider variety of food sources. Further, bacterial metabolites isolated from the gut of the fruit fly (Drosophila melanogaster) have been shown to increase immune gene expression of pathways that promote the activity of Relish/NF-κB - a family of pleiotropic transcription factors that are highly conserved across Animalia[32,33]. As innate immunity is greatly conserved across flies and mammals, understanding the microbial modulation of these pathways in insects can also enrich our comprehension of immune signaling in diverse animal systems. Altogether, the simple growth requirements and efficiency of insect cell lines, as well as the applicability of many conserved cellular systems across insects and vertebrates, position insect cell culture as a powerful model system for studying host-microbe interactions relevant to a very wide range of host species.
Despite their applicability, a significant constraint in the use of insect cell lines for microbiome research is the limited availability of continuous cell lines derived from relevant insect gut tissue. Gut cell lines have been established for only nine insect species, and many of the most heavily studied insects, such as
An additional factor to consider when investigating the microbiome using cell lines is how closely a cell line approximates the gut environment. Primary cell lines, derived from isolated tissue, retain most of their
Recently, insect cell lines derived from various tissues have been subjected to detailed genome or transcriptome profiling to gain insights into characteristics important for recombinant protein expression[38-40]. However, when considering insect gut cell lines, genotypic and phenotypic characterization is lacking.
The RP-HzGUT-AW1 cell line, derived from Lepidoptera member Helicoverpa zea, is one of the first insect cell lines to be characterized via transcriptomics for expression of insect intestinal epithelial cell gene and intestinal stem cell markers and, therefore, is the most characterized in gut approximation[41].
The lack of basic insect gut cell line characterization is a major hurdle in the development of biologically relevant cell lines that respond to stimuli (e.g., microbial metabolites, chemical treatments of interest) in a manner that is predictable and comparable to in vivo conditions. Thus, while current insect cell cultures offer unique insights, their limited representation of genuine, characterized gut tissues can constrain the depth and overall breadth of host-microbe interaction studies. Here, we highlight lessons learned from human gut-derived cell lines and describe several of the latest techniques that show promise for enabling targeted derivation of insect gut cell lines.
Caco-2 and the microbiome: an exemplar approach
Cell culture techniques have been effectively leveraged to gain insight into mammalian epithelial cell-microbial relationships over the past several decades. The human cell line model of the gut epithelium most widely used for this purpose is likely the Caco-2 line, derived by Fogh in 1977 from a human colon adenocarcinoma biopsy. Despite their derivatization from the colon, these cells behave most similarly to small intestine epithelial tissue, both biochemically and morphologically[42]. Demonstrating their broad-spectrum application, Caco-2 cells have been used in thousands of studies to illuminate the pathogenesis of deleterious microbes, probiotic formulations, and immune-microbe interactions[43-45]. For example, Caco-2 cells have been employed to confirm both the invasiveness of, and the cytokine response to Fusobacterium nucleatum - a bacterium associated with colorectal cancer - in a relevant intestinal cell model[45,46]. Additionally, this cell line has been used to evaluate the potential beneficial properties of the yeast Kluyveromyces marxianus, strain B0299[44], where it was observed that the yeast dampened the release of pro-inflammatory cytokines by cells treated with lipopolysaccharide, an inflammatory stimulus, which ultimately led to the yeast being considered as a therapeutically useful probiotic[44].
A further aspect that extends the applicability of the Caco-2 cell model to biomedical science is that the cells can be co-cultured with other cell types and incorporated into several advanced biomimetic models. For instance, the co-culture of Caco-2 and Raji B cells, a lymphoblast-like cell line, facilitates the production of a model of specialized microfold epithelial cells, which overlay the gut-associated lymphoid tissues[47]. This model can subsequently be utilized to study the transport of microbial-derived micro- and nano-compounds across this epithelial barrier cell type[47,48]. Caco-2 cells have also been integrated into organ-on-chip models, which are micro-biomimetic systems that incorporate multiple cell types and provide a framework for cellular growth[49]. This model system more closely represents in vivo tissue, improving the efficacy of in vitro studies.
Considering the extensive insights gained from using mammalian cell lines such as Caco-2, similar methodologies should be applied to insect models. However, since spontaneously immortalized tissue is rare to identify in insects under natural circumstances, the pivotal challenge of deriving gut-specific cell types lies primarily in the isolation procedure rather than the general maintenance steps. The tailored methodologies that show the most promise for isolating gut-derived cell lines able to grow continuously (i.e., which exhibit immortalization) under laboratory conditions are discussed below.
PRODUCING IMMORTALIZED INSECT GUT CELL LINES
Cell types relevant to insect gut cell line development
Contrasting the human gut epithelium, the insect gut epithelium is relatively simple, consisting of two major cell types: columnar epithelial cells and endocrine cells [Figure 2][50]. Additionally, stem cells, goblet cells, and copper cells (responsible for acidification of the digestive tract) are often present [Figure 2][50,51]. Columnar epithelial cells closely resemble the stereotypical enterocytes found in humans and function similarly. We suggest that columnar epithelial cells, as the most prevalent cell type in the insect gut epithelium, represent a good target for successful cultivation during cell line isolation procedures. It is important to note that non-target cell types, such as muscle cells, exist within the insect gut as well[50]. As a result, cell lines are not always composed of their target cell type and should be verified by, at minimum, microscopy prior to further experimentation.
Figure 2. Targeted zones to produce insect gut cell lines. Schematic representation of the insect digestion system, which can be broken down into three regions: the foregut, midgut, and hindgut. The midgut is the primary choice from which to derive cell lines on account of it playing a key role in shaping the gut microbiome and being the principal location of nutrient absorption, pathogen defense, and the production of signaling molecules[50]. The midgut has several cell types, including columnar epithelial cells (CC), endocrine cells (EC), goblet cells (GC), and stem cells (SC)[50]. Only the most relevant of the top five cell types are shown. Columnar epithelial cells are the most common, followed by endocrine cells and stem cells, making these cells the first selection for cell culture[50]. Created with BioRender.com[20].
Methods to attain immortalized insect gut cell lines
Cell lines are considered immortalized once they no longer possess the ability to respond to cell cycle controls. These controls, present at the G1 and G2 cell cycle checkpoints, limit cellular proliferation and, when required, lead to cellular senescence or apoptosis[52]. Bypassing these controls can, on rare occasions, occur naturally in a process known as spontaneous immortalization[50]. Alternatively, this can be achieved deliberately, for example, through the insertion of proto-oncogenes, pathogen invasion, Clustered Regularly Interspaced Short Palindromic Repeats (CRISPR)-Cas9, and exposure to carcinogenic chemicals or ionizing radiation[53-56]. Immortalization is particularly difficult to achieve in mature tissue, such as the gut epithelium, because of its limited replicative potential and greater likelihood of senescence[48]. Notwithstanding these difficulties, many insect cell lines have been successfully produced from mature tissue. Table 1 lists the currently available insect cell lines that have been immortalized. Several common techniques for the production of insect gut cell lines are relevant to discuss.
Categorization of the 17 available immortalized insect gut cell lines
Cell line name(s) | Species | Age at isolation | Suggested cell type | Date published | Cellosaurus accession #(s) |
BCIRL-HZ-MG8 | Corn earworm moth (Helicoverpa zea) | Unspecified | Unspecified (untested) | 2003[57] | CVCL_ZF01 |
BPH22 | Painted grasshopper (Poekilocerus pictus) | Nymph | Stem cells (differentiates into goblet and epithelial cells) | 2010[58] | CVCL_A2PR |
BTI-Tn-MG1, MG1-ht33, MG1-ht35 | Cabbage looper (Trichoplusia ni) | Fifth instar larva | Unspecified, mixed morphology | 1991[59] | CVCL_Z093, CVCL_UF21, CVCL_UF22 |
CT/BCIRL-SfMG-0617-KZ, CT/BCIRL-SfMG1-0611-E7-KZ, CT/BCIRL-SfMG1-0611-E8-KZ, CT/BCIRL-SfMG1-0611-KZ | Fall armyworm (Spodoptera frugiperda) | Fifth instar larval stage, sixth instar larva | Unspecified, mixed morphology (muscle and epithelial origin, has residual epithelial cells) | 2019[60] | CVCL_UZ18, CVCL_C2T6, CVCL_C2T7, CVCL_UZ19 |
FPMI-CF-200, FPMI-CF-203, FPMI-CF-204, FPMI-CF-205, IPRI-CF-1 | Spruce budworm moth (Choristoneura fumiferana) | Adult, neonate larva | Unspecified, mixed morphology | 1993[61] | CVCL_WV83, CVCL_Z469, CVCL_WV85, CVCL_WV84, CVCL_Z473 |
HNU-Ha-MG1 | Cotton bollworm (Helicoverpa armigera) | Fourth instar larva | Unspecified, mixed morphology | 2015[62] | CVCL_HF52 |
LSTM-AG-55 | African malaria mosquito (Anopheles gambiae) | First instar larva | Epithelial-type cells | 1972[63] | CVCL_Z360 |
RPW-1 | Red palm weevil (Rhynchophorus ferrugineus) | Fifth instar larva | Midgut epithelial cells (untested) | 2013[64] | CVCL_A2PS |
Spontaneous immortalization
Spontaneous immortalization occurs when a cell line is immortalized without exposure to carcinogens or direct genetic manipulation[50]. Continuous passaging of a primary cell line increases the likelihood of random epigenetic changes or chromosomal rearrangements[50]. Often, a mutation occurs in the p53 pathway[50,65]. Although considered a rare event, spontaneous immortalization has led to the production of all currently existing insect gut cell lines[10]. Notably, midgut cell lines have been successfully produced in this manner, including CT/BCIRL-SfMG1-0611-E8-KZ (Fall armyworm midgut)[10].
Malignant neoplasms
Neoplasia has been reported in various insect tissues, including hemolymph, epithelial tissue, and neural tissue[66-68]. Many human cell lines, including Caco-2, have been derived from a malignant neoplasm, but no insect gut cell lines have been established using this approach. This is particularly notable, as
Insertion of proto-oncogenes
Proto-oncogenes play essential roles in the normal growth and development of cells, however, they can potentially create malignant neoplasms if excessive copies accumulate or mutations occur, creating an oncogene[70]. Proto-oncogenes can be introduced to cells through a method known as lipofection, which transfects DNA using a synthetic cationic lipid complex[71,72]. Lipofection of the human C-myc proto-oncogene into embryonic A. mellifera cells successfully produced the MYN9 cell line[54]. Considered a master regulator, excessive C-myc can lead to immortalization by inciting reactive oxygen species production and promoting p53 phosphorylation, disrupting normal cell cycle function[71,73]. Another proto-oncogene used in cell line production is ras, an important regulator of proliferation, growth, and survival[71]. Both the Ras[V12]-H1 and Ras[V12]-H7 D. melanogaster cell lines include mutations in the ras proto-oncogene[74].
Entomopathogens
Certain pathogens incite tumorigenesis or reduce apoptosis in the gut epithelial tissue of insects. In one study, the European spruce sawfly (Gilpinia hercyniae) developed multiple midgut tumors after exposure to a polyhedral virus[75]. Additionally, Nosema cerenae, a fungal pathogen of A. mellifera, reduces apoptosis of midgut epithelial cells by increasing the transcription of the inhibitor of apoptosis-2 gene[76]. The direct application of entomopathogens in insect cell line production has yet to be explored; however, this is not to suggest that pathogens may not have already played a critical role. Both existing A. mellifera cell lines (AmE-711 and MYN9) are known to be infected with deformed wing virus[77], which could have contributed to their immortalization.
CRISPR-Cas9
CRISPR-Cas9 allows for the targeted insertion and deletion of genes from a host genome. CRISPR-Cas9 technology has recently been used to immortalize mature human prostate epithelial cells of the PrEC cell line[56]. In this cell line, the inactivation of CDKN2A at two loci induced immortalization, but also maintained the characteristics of normal cells, including a normal p53 response[56]. CRISPR-Cas9 has also been used to edit several existing insect cell lines, including insect cell lines Sf-21 and S2R+[78,79]. Despite great potential, CRISPR-Cas9 has not yet been employed to produce a novel insect cell line. Future research endeavors may benefit from editing genes that have been empirically linked to midgut tumorigenesis in
Radiation
Ionizing radiation (X-rays and Gamma-rays) induces direct DNA damage in the form of single- and double-stranded breaks and indirect DNA damage through the production of reactive oxygen species[80]. Following the theory of radiation hormesis, low-dose radiation is purported to improve cellular health, but high-dose radiation is extremely damaging and results in DNA breaks[81]. Radiation causes apoptosis in the majority of exposed cells, but has the potential to produce providential mutations, leading to immortalization in a subset of these cells. The KMST-6 human fibroblast cell line was produced in this way using Gamma radiation[53], illustrating the promise of this technique. Furthermore, literature from as early as 1928 has associated X-ray exposure with genetic mutation in insects, indicating promise for future work using radiation to deliberately transform insect cells to immortalize them[69].
INSECT GUT CELL LINES HAVE THE POTENTIAL TO ADDRESS MICROBIOME-RELATED KNOWLEDGE GAPS
Defining a “healthy” insect microbiome
A substantial knowledge gap that could be addressed by insect gut cell lines is the balance of microbes that constitutes a healthy, or “eubiotic,” gut microbiome[82]. Eubiosis in insects is poorly defined, as is the opposite state, dysbiosis. This, in large part, is due to the context-dependent nature and inherent subjectivity of the gut microbiome[83]. Additionally, very few insect species have well-studied microbiomes, furthering this lack of understanding.
One theory that attempts to define microbiome health in insects follows the Anna Karenina principle of “Happy families are all alike; every unhappy family is unhappy in its own way”[84,85]. According to this framework, once a eubiotic species balance is achieved, any deviation from this species balance will destabilize the system, leading to dysbiosis. This principle applies well to social insect species, which possess highly conserved and vertically transmitted microbiome compositions[86,87]. However, the theory fails to consider solitary insect microbiomes, which more often mimic the composition of their environment[6,88]. Other researchers have argued that the level of connectedness determines the health of the microbiome, rather than the balance of species[84]. Just as for the human microbiome, it is unknown what truly constitutes a “healthy” insect microbiome, especially when the correct balance differs from species to species. Insect gut cell lines hold the potential to elucidate the roles of microbes and their contributions towards eubiosis or dysbiosis within the insect gut, and the animal as a whole.
Culturing the uncultured
For most animals, the gut houses the greatest number of microorganisms in the body, many of which remain uncultured[72,89], meaning culture cannot be achieved using traditional microbiological methods, likely because agars and broths utilized in laboratory settings do not replicate the complex physiological and chemical conditions of the gut environment[29]. One option to improve culture conditions is the employment of bioreactor models, which show great promise in cultivating entire gut-derived communities, but still lack the host cell component[90]. In future studies, bioreactors could be linked to cell culture through microbial metabolites. Cell-free supernatant from bioreactors is a source of microbial metabolites, which can be filter-sterilized and applied to insect cell lines to replicate host-microbial metabolite interactions. Since much of the interaction between a microbe and an animal cell is mediated through metabolite signaling, the use of metabolites alone helps preserve cell line integrity while understanding host-microbe interactions. This may be achieved by assessing cytokine release, localization within a host cell, and the up- or downregulation of host gene expression.
In addition, since cell culture replicates the gut tissue microenvironment, it allows for the cultivation and isolation of sensitive microbes that are strictly dependent on the presence of host cells[29]. For example, obligate intracellular microbes, which rely entirely on eukaryotic host cells, must be cultured in this manner[29], including the endosymbiont W. pipientis, which was first cultured and isolated utilizing the Aa23 cell line, derived from the Asian tiger mosquito (Aedes albopictus)[28]. Through the use of insect gut cell lines, previously uncultured bacteria, archaea, and fungi can be isolated, enhancing our comprehension of insect pathogens, commensals, and symbionts. Further, undiscovered constituents of the insect microbiome may possess characteristics valuable to human industry and pharmaceuticals.
In highlighting the efficacy of cell lines for investigating facultative symbionts, the 2018 study by Chevignon et al. stands out as a notable example[91]. To explore the “unculturable” aphid symbiont Hamiltonella defensa, crucial for safeguarding its host against parasitoid wasps, Chevignon et al. leveraged the immortalized TN5 insect cell line[91]. The utilization of cell lines in cultivating H. defensa yielded a higher abundance of the symbiont compared to in vivo cultures[91]. This enhancement enabled the researchers to successfully generate the complete genomes for various H. defensa strains, thereby unraveling the genetic variance of strains and genome elements responsible for the protective abilities exhibited by H. defensa[91].
Understanding the microbiome’s toxin defense systems
The insect microbiome serves as a critical barrier between toxins and host tissue. The scientific literature has documented the ability of insect gut microbes to mitigate toxin exposure to the host through the breakdown of external compounds, such as insecticides, as well as host-secreted wastes[6]. The insect microbiome also exhibits a capacity to adapt to toxin exposure. Namely, microbes of the brown plant hopper (Nilaparvata lugens), an agricultural pest, displayed heightened expression of detoxifying genes, including NlCYP6ER1, after repeated exposure to insecticides[92]. Additionally, a commensal bacterial species of D. melanogaster, Lacticaseibacillus (formerly Lactobacillus) rhamnosus, reduced the absorption of organophosphate insecticides by the host[93]. Furthermore, several lactic acid bacteria strains, isolated from the gut of managed A. mellifera, had the ability to detoxify representative insecticides in cell line models[94]. While encouraging, it is important to note that none of the cell lines used (ovarian insect Sf-9, rat intestinal IEC-6, and human intestinal Caco-2) were of A. mellifera origin and the detoxification abilities observed were contingent on the cell lines employed[94]. The relevance of cross-species studies is thus context-dependent, underscoring the importance of deriving and employing species-specific cell lines in insect-related research to ensure the accuracy of results.
Despite progress in the field, a significant knowledge gap remains regarding the impact of specific toxins on host tissue in isolation as well as in the presence of various resident and foreign microbes. This gap may be addressed by insect gut cell lines, which in turn could help to advance pest management strategies, and to shield vulnerable species from the noxious effects of toxin exposure.
Exploring specific symbiont-derived pathogen defense in host environment
The application of cell lines in the investigation of host-pathogen-symbiont interactions will promote a more comprehensive understanding of symbiont-derived defense and its impact on the host, while avoiding confounders associated with host models. These confounders include the inherent colonization of the host by other microbiome members, which could make any interaction studies non-specific, or would require the use of germ-free hosts (which often are not available). Few studies have used cell line models to look at such interactions. One study of note used a rotating wall vessel-derived (RWV) 3-D HT29 organotype model, composed of a human adenocarcinoma cell line with epithelial morphology (HT-29), to investigate the interactions between symbiont, human host, and pathogen during infection[95]. The RWV model was coinfected with commensal bacteria Lactobacillus reuteri and the pathogen Salmonella enterica, in order to determine the protective ability of L. reuteri and its impact on the host[95]. The study revealed that L. reuteri reduced pathogen growth and adhesion to HT-29 cells, leading to L. reuteri being considered a potential candidate for probiotic therapy against Salmonella infection[95]. This study highlights the potential of cell culture to elucidate mechanisms underlying symbiont-derived pathogen defense, as well as the repercussions for all cells involved.
A wider range of host-specific gut cell lines could improve insect work by creating an accurate environment to inoculate both pathogen and potential symbiont, resulting in more natural responses to what would happen within the specific host. With the use of insect gut cell lines, research of this nature could help to identify safe probiotic candidate bacteria against the most relevant “incurable” insect pathogens, such as Paenibacillus larvae in honeybees, gain insights on microbiome members important for host defense, and the impact of both pathogen and symbiont presence in host tissues[96].
LIMITATIONS
The key limitation associated with cell culture is its inability to fully recapitulate in vivo organ and tissue environments[1]. While the simplistic model of cell culture is efficacious in the study of isolated variables, it consequently cannot assess the impact of the same variables on complex systems, such as the reproductive and immune systems. This discrepancy could be addressed in the future through the production of
CONCLUSION
Insect gut cell lines offer a high-resolution and relevant model for the exploration of the microbiome and its complex interactions with host nutrition, immunity, and toxicology. Despite decades of research in the fields of the insect microbiome and insect cell culture, limited integration has occurred between the two. This starkly contrasts the study of the human microbiome, which has utilized cell culture extensively. The time has come to explore novel ways to study host-microbiome relationships in insects in the laboratory, and cell lines represent an important tool to exploit. This is becoming more important as insects continue to face unrelenting threats as a result of human development and habitat encroachment.
DECLARATIONS
Authors’ contributions
Contributed to the conceptualization and ideas presented, drafted the manuscript and revised it: Macpherson CV, Daisley BA, Mallory E, Allen-Vercoe E
Produced all tables and figures: Macpherson CV
All authors have read and agreed to the published version of the manuscript.
Availability of data and materials
Not applicable.
Financial support and sponsorship
This work was supported by an Ontario Ministry of Agriculture, Food and Rural Affairs (OMAFRA) Special Initiatives grant (UG-SI-2022-102161), a Canada First Research Excellence Fund (CFREF) Food from Thought (FfT) Thematic III Research Grant (THE3-00525), a Banting Postdoctoral Fellowship Award (BPF-489532-402947), an Abell Pest Control Scholarship in Pollinator Research, and a University of Guelph College of Biological Sciences Graduate Tuition Scholarship.
Conflicts of interest
All authors declared that there are no conflicts of interest. Allen-Vercoe E is an Editorial Board member of the journal Microbiome Research Reports.
Ethical approval and consent to participate
Not applicable.
Consent for publication
Not applicable.
Copyright
© The Author(s) 2024.
REFERENCES
1. Segeritz C-P, Vallier L. Chapter 9 - cell culture: growing cells as model systems in vitro. In: Jalali M, Saldanha FYL, Jalali M, editors. Basic science methods for clinical researchers. Boston: Academic Press; 2017. pp. 151-72.
2. Berg G, Rybakova D, Fischer D, et al. Microbiome definition re-visited: old concepts and new challenges. Microbiome 2020;8:103.
3. Lopez-Escalera S, Wellejus A. Evaluation of Caco-2 and human intestinal epithelial cells as in vitro models of colonic and small intestinal integrity. Biochem Biophys Rep 2022;31:101314.
4. van Nuenen MHMC, de Ligt RAF, Doornbos RP, van der Woude JCJ, Kuipers EJ, Venema K. The influence of microbial metabolites on human intestinal epithelial cells and macrophages in vitro. FEMS Immunol Med Microbiol 2005;45:183-9.
5. Jahani-Sherafat S, Azimirad M, Ghasemian-Safaei H, et al. The effect of intestinal microbiota metabolites on HT29 cell line using MTT method in patients with colorectal cancer. Gastroenterol Hepatol Bed Bench 2019;12:S74-9.
6. Engel P, Moran NA. The gut microbiota of insects - diversity in structure and function. FEMS Microbiol Rev 2013;37:699-735.
7. Jing TZ, Qi FH, Wang ZY. Most dominant roles of insect gut bacteria: digestion, detoxification, or essential nutrient provision? Microbiome 2020;8:38.
9. Janicki J, Dickie G, Scarr S, Chowdhury J. The collapse of insects. 2022. Available from: https://www.reuters.com/graphics/GLOBAL-ENVIRONMENT/INSECT-APOCALYPSE/egpbykdxjvq/. [Last accessed on 26 Feb 2024].
10. Cellosaurus - a knowledge resource on cell lines. Available from: https://www.cellosaurus.org/. [Last accessed on 26 Feb 2024].
11. Stork NE. How many species of insects and other terrestrial arthropods are there on earth? Annu Rev Entomol 2018;63:31-45.
12. Glaser RW, Chapman JW. Studies on the wilt disease, or “flacheria” of the gypsy moth. Science 1912;36:219-24.
13. Smagghe G, Goodman CL, Stanley D. Insect cell culture and applications to research and pest management. In Vitro Cell Dev Biol Anim 2009;45:93-105.
14. Grace TDC. Establishment of four strains of cells from insect tissues grown in vitro. Nature 1962;195:788-9.
15. Drugmand JC. Characterization of insect cell lines is required for appropriate industrial processes: case study of high-five cells for recombinant protein production. 2007. Available from: https://dial.uclouvain.be/pr/boreal/en/object/boreal%3A4586. [Last accessed on 26 Feb 2024]
16. Vaughn JL, Goodwin RH, Tompkins GJ, McCawley P. The establishment of two cell lines from the insect Spodoptera frugiperda (lepidoptera; noctuidae). In Vitro 1977;13:213-7.
17. Rubio NR, Fish KD, Trimmer BA, Kaplan DL. Possibilities for engineered insect tissue as a food source. Front Sustain Food Syst 2019;3:24.
18. Gisder S, Möckel N, Linde A, Genersch E. A cell culture model for Nosema ceranae and Nosema apis allows new insights into the life cycle of these important honey bee-pathogenic microsporidia. Environ Microbiol 2011;13:404-13.
19. Goblirsch MJ, Spivak MS, Kurtti TJ. A cell line resource derived from honey bee (Apis mellifera) embryonic tissues. PLoS One 2013;8:e69831.
20. Create professional science figures in minutes. BioRender. Available from: https://www.biorender.com/. [Last accessed on 26 Feb 2024].
21. Mitsuhashi J. Cell culture of insects. In: Encyclopedia of entomology. Dordrecht: Springer Netherlands; 2005. pp. 480.
22. Michl J, Park KC, Swietach P. Evidence-based guidelines for controlling pH in mammalian live-cell culture systems. Commun Biol 2019;2:144.
23. Singh L, Nair L, Kumar D, et al. Hypoxia induced lactate acidosis modulates tumor microenvironment and lipid reprogramming to sustain the cancer cell survival. Front Oncol 2023;13:1034205.
25. Conceição CC, da Silva JN, Arcanjo A, et al. Aedes fluviatilis cell lines as new tools to study metabolic and immune interactions in mosquito-Wolbachia symbiosis. Sci Rep 2021;11:19202.
26. Watanabe K, Yukuhiro F, Matsuura Y, Fukatsu T, Noda H. Intrasperm vertical symbiont transmission. Proc Natl Acad Sci U S A 2014;111:7433-7.
27. Brandt JW, Chevignon G, Oliver KM, Strand MR. Culture of an aphid heritable symbiont demonstrates its direct role in defence against parasitoids. Proc Biol Sci 2017;284:20171925.
28. O’Neill SL, Pettigrew MM, Sinkins SP, Braig HR, Andreadis TG, Tesh RB. In vitro cultivation of Wolbachia pipientis in an Aedes albopictus cell line. Insect Mol Biol 1997;6:33-9.
29. Rajagopal R. Beneficial interactions between insects and gut bacteria. Indian J Microbiol 2009;49:114-9.
30. Menezes C, Vollet-Neto A, Marsaioli AJ, et al. A Brazilian social bee must cultivate fungus to survive. Curr Biol 2015;25:2851-5.
31. Briones-Roblero CI, Rodríguez-Díaz R, Santiago-Cruz JA, Zúñiga G, Rivera-Orduña FN. Degradation capacities of bacteria and yeasts isolated from the gut of Dendroctonus rhizophagus (Curculionidae: Scolytinae). Folia Microbiol 2017;62:1-9.
33. Buchon N, Silverman N, Cherry S. Immunity in Drosophila melanogaster - from microbial recognition to whole-organism physiology. Nat Rev Immunol 2014;14:796-810.
34. Marian B. In vitro models for the identification and characterization of tumor-promoting and protective factors for colon carcinogenesis. Food Chem Toxicol 2002;40:1099-104.
35. Langerholc T, Maragkoudakis PA, Wollgast J, Gradisnik L, Cencic A. Novel and established intestinal cell line models - an indispensable tool in food science and nutrition. Trends Food Sci Technol 2011;22:S11-20.
36. Costa J, Ahluwalia A. Advances and current challenges in intestinal in vitro model engineering: a digest. Front Bioeng Biotechnol 2019;7:144.
37. Cencic A, Langerholc T. Functional cell models of the gut and their applications in food microbiology - a review. Int J Food Microbiol 2010;141:S4-14.
38. Koczka K, Peters P, Ernst W, Himmelbauer H, Nika L, Grabherr R. Comparative transcriptome analysis of a Trichoplusia ni cell line reveals distinct host responses to intracellular and secreted protein products expressed by recombinant baculoviruses. J Biotechnol 2018;270:61-9.
39. Fu Y, Yang Y, Zhang H, et al. The genome of the Hi5 germ cell line from Trichoplusia ni, an agricultural pest and novel model for small RNA biology. Elife 2018;7:e31628.
40. Wei L, Cao L, Miao Y, et al. Transcriptome analysis of Spodoptera frugiperda 9 (Sf9) cells infected with baculovirus, AcMNPV or AcMNPV-BmK IT. Biotechnol Lett 2017;39:1129-39.
41. Vorgia E, Lamprousi M, Denecke S, et al. Functional characterization and transcriptomic profiling of a spheroid-forming midgut cell line from Helicoverpa zea (Lepidoptera: Noctuidae). Insect Biochem Mol Biol 2021;128:103510.
42. Sun H, Chow ECY, Liu S, Du Y, Pang KS. The Caco-2 cell monolayer: usefulness and limitations. Expert Opin Drug Metab Toxicol 2008;4:395-411.
43. Bahrami B, Child MW, Macfarlane S, Macfarlane GT. Adherence and cytokine induction in Caco-2 cells by bacterial populations from a three-stage continuous-culture model of the large intestine. Appl Environ Microbiol 2011;77:2934-42.
44. Maccaferri S, Klinder A, Brigidi P, Cavina P, Costabile A. Potential probiotic Kluyveromyces marxianus B0399 modulates the immune response in Caco-2 cells and peripheral blood mononuclear cells and impacts the human gut microbiota in an in vitro colonic model system. Appl Environ Microbiol 2012;78:956-64.
45. Castellarin M, Warren RL, Freeman JD, et al. Fusobacterium nucleatum infection is prevalent in human colorectal carcinoma. Genome Res 2012;22:299-306.
46. Dharmani P, Strauss J, Ambrose C, Allen-Vercoe E, Chadee K. Fusobacterium nucleatum infection of colonic cells stimulates MUC2 mucin and tumor necrosis factor alpha. Infect Immun 2011;79:2597-607.
47. Kleiveland CR. Co-culture Caco-2/immune cells. In: Verhoeckx K, Cotter P, López-Expósito I, et al., editors. The impact of food bioactives on health. Cham: Springer; 2015.
48. Lozoya-Agullo I, Araújo F, González-Álvarez I, et al. Usefulness of Caco-2/HT29-MTX and Caco-2/HT29-MTX/Raji B coculture models to predict intestinal and colonic permeability compared to Caco-2 monoculture. Mol Pharm 2017;14:1264-70.
49. Singh D, Mathur A, Arora S, Roy S, Mahindroo N. Journey of organ on a chip technology and its role in future healthcare scenario. Appl Surf Sci Adv 2022;9:100246.
50. Caccia S, Casartelli M, Tettamanti G. The amazing complexity of insect midgut cells: types, peculiarities, and functions. Cell Tissue Res 2019;377:505-25.
51. Dubreuil RR. Copper cells and stomach acid secretion in the Drosophila midgut. Int J Biochem Cell Biol 2004;36:745-52.
52. Wright WE, Shay JW. The two-stage mechanism controlling cellular senescence and immortalization. Exp Gerontol 1992;27:383-9.
53. Namba M, Nishitani K, Hyodoh F, Fukushima F, Kimoto T. Neoplastic transformation of human diploid fibroblasts (KMST-6) by treatment with 60Co gamma rays. Int J Cancer 1985;35:275-80.
54. Kitagishi Y, Okumura N, Yoshida H, Nishimura Y, Takahashi J, Matsuda S. Long-term cultivation of in vitro Apis mellifera cells by gene transfer of human c-myc proto-oncogene. In Vitro Cell Dev Biol Anim 2011;47:451-3.
56. Zhao Z, Fowle H, Valentine H, et al. Immortalization of human primary prostate epithelial cells via CRISPR inactivation of the CDKN2A locus and expression of telomerase. Prostate Cancer Prostatic Dis 2021;24:233-43.
57. Pringle FM, Johnson KN, Goodman CL, McIntosh AH, Ball LA. Providence virus: a new member of the tetraviridae that infects cultured insect cells. Virology 2003;306:359-70.
58. Kharat KR, Sawant MV, Peter S, Hardikar BP. Development and characterization of new cell line BPH22 from midgut epithelial cells of Poekilocerus pictus (Fabricius, 1775). In Vitro Cell Dev Biol Anim 2010;46:824-7.
59. Granados RR. Cell line isolated from larval midgut tissue of Trichoplusia ni. 1994. Available from: https://patents.google.com/patent/US5298418A/en. [Last accessed on 26 Feb 2024].
60. Zhou K, Goodman CL, Ringbauer J Jr, Song Q, Beerntsen B, Stanley D. Establishment of two midgut cell lines from the fall armyworm, Spodoptera frugiperda (Lepidoptera: Noctuidae). In Vitro Cell Dev Biol Anim 2020;56:10-4.
61. Abstracts. In vitro cell dev biol anim 1993;29A:30-125A.
62. Li J, He F, Yang Y, et al. Establishment and characterization of a novel cell line from midgut tissue of Helicoverpa armigera (Lepidoptera: Noctuidae). In Vitro Cell Dev Biol Anim 2015;51:562-71.
63. Marhoul Z, Pudney M. A mosquito cell line (mos. 55) from Anopheles gambiae larva. Trans R Soc Trop Med Hyg 1972;66:183-4.
64. Aljabr AM, Rizwan-ul-Haq M, Hussain A, Al-Mubarak AI, Al-Ayied HY. Establishing midgut cell culture from Rhynchophorus ferrugineus (Olivier) and toxicity assessment against ten different insecticides. In Vitro Cell Dev Biol Anim 2014;50:296-303.
65. Harvey DM, Levine AJ. p53 alteration is a common event in the spontaneous immortalization of primary BALB/c murine embryo fibroblasts. Genes Dev 1991;5:2375-85.
66. Martorell Ò, Merlos-Suárez A, Campbell K, et al. Conserved mechanisms of tumorigenesis in the Drosophila adult midgut. PLoS One 2014;9:e88413.
67. Pastor-Pareja JC, Wu M, Xu T. An innate immune response of blood cells to tumors and tissue damage in Drosophila. Dis Model Mech 2008;1:144-54.
68. Scharrer B. Insect tumors induced by nerve severance: incidence and mortality. Cancer Res 1953;13:73-6.
69. Villegas SN. One hundred years of Drosophila cancer research: no longer in solitude. Dis Model Mech 2019;12:dmm039032.
70. Proto-oncogenes to oncogenes to cancer. Scitable. Available from: https://www.nature.com/scitable/topicpage/proto-oncogenes-to-oncogenes-to-cancer-883/. [Last accessed on 26 Feb 2024].
71. Frühauf MI, Barcelos LDS, Botton NY, et al. Alternatives for obtaining a continuous cell line from Apis mellifera. Cienc Rural 2021;51:e20201111.
72. Felgner PL, Gadek TR, Holm M, et al. Lipofection: a highly efficient, lipid-mediated DNA-transfection procedure. Proc Natl Acad Sci U S A 1987;84:7413-7.
73. Poole CJ, van Riggelen J. MYC - master regulator of the cancer epigenome and transcriptome. Genes 2017;8:142.
74. Dequéant ML, Fagegaltier D, Hu Y, et al. Discovery of progenitor cell signatures by time-series synexpression analysis during Drosophila embryonic cell immortalization. Proc Natl Acad Sci U S A 2015;112:12974-9.
76. Kurze C, Le Conte Y, Dussaubat C, et al. Nosema tolerant honeybees (Apis mellifera) escape parasitic manipulation of apoptosis. PLoS One 2015;10:e0140174.
77. Insect cell lines. Department of Entomology at the University of Kentucky. Available from: https://entomology.ca.uky.edu/aginsectcellsdatabase?combine=&field_cell_order_tid=303&field_cell_species_tid=All&field_cell_stage_tid=All&field_cell_status_tid=All&field_cell_tissue_tid=All. [Last accessed on 26 Feb 2024].
78. Sari-Ak D, Alomari O, Shomali RA, Lim J, Thimiri Govinda Raj DB. Advances in CRISPR-Cas9 for the baculovirus vector system: a systematic review. Viruses 2022;15:54.
79. Nweke EE, Thimiri Govinda Raj DB. Chapter one - development of insect cell line using CRISPR technology. In: Singh V, editor. Progress in molecular biology and translational science. Prog Mol Biol Transl Sci 2021;180:1-20.
80. Carlos-Reyes A, Muñiz-Lino MA, Romero-Garcia S, López-Camarillo C, Hernández-de la Cruz ON. Biological adaptations of tumor cells to radiation therapy. Front Oncol 2021;11:718636.
81. Vaiserman A, Cuttler JM, Socol Y. Low-dose ionizing radiation as a hormetin: experimental observations and therapeutic perspective for age-related disorders. Biogerontology 2021;22:145-64.
82. Iebba V, Totino V, Gagliardi A, et al. Eubiosis and dysbiosis: the two sides of the microbiota. New Microbiol 2016;39:1-12.
83. Daisley BA, Koenig D, Engelbrecht K, et al. Emerging connections between gut microbiome bioenergetics and chronic metabolic diseases. Cell Rep 2021;37:110087.
84. Marasco R, Fusi M, Callegari M, et al. Destabilization of the bacterial interactome identifies nutrient restriction-induced dysbiosis in insect guts. Microbiol Spectr 2022;10:e0158021.
85. Tolstoy L. Anna Karenina. The Russian Messenger; 1878.
86. Sabree ZL, Huang CY, Arakawa G, et al. Genome shrinkage and loss of nutrient-providing potential in the obligate symbiont of the primitive termite Mastotermes darwiniensis. Appl Environ Microbiol 2012;78:204-10.
87. Su Q, Wang Q, Mu X, et al. Strain-level analysis reveals the vertical microbial transmission during the life cycle of bumblebee. Microbiome 2021;9:216.
88. De Landa GF, Alberoni D, Baffoni L, et al. The gut microbiome of solitary bees is mainly affected by pathogen assemblage and partially by land use. Environ Microbiome 2023;18:38.
89. Sender R, Fuchs S, Milo R. Revised estimates for the number of human and bacteria cells in the body. PLoS Biol 2016;14:e1002533.
90. Gianetto-Hill CM, Vancuren SJ, Daisley B, et al. The Robogut: a bioreactor model of the human colon for evaluation of gut microbial community ecology and function. Curr Protoc 2023;3:e737.
91. Chevignon G, Boyd BM, Brandt JW, Oliver KM, Strand MR. Culture-facilitated comparative genomics of the facultative symbiont Hamiltonella defensa. Genome Biol Evol 2018;10:786-802.
92. Zhang Y, Cai T, Yuan M, et al. Microbiome variation correlates with the insecticide susceptibility in different geographic strains of a significant agricultural pest, Nilaparvata lugens. NPJ Biofilms Microbiomes 2023;9:2.
93. Trinder M, McDowell TW, Daisley BA, et al. Probiotic Lactobacillus rhamnosus reduces organophosphate pesticide absorption and toxicity to Drosophila melanogaster. Appl Environ Microbiol 2016;82:6204-13.
94. Leska A, Nowak A, Miśkiewicz K, Rosicka-Kaczmarek J. Binding and detoxification of insecticides by potentially probiotic lactic acid bacteria isolated from honeybee (Apis mellifera L.) environment - an in vitro study. Cells 2022;11:3743.
95. De Weirdt R, Crabbé A, Roos S, et al. Glycerol supplementation enhances L. reuteri’s protective effect against S. typhimurium colonization in a 3-D model of colonic epithelium. PLoS One 2012;7:e37116.
96. Daisley BA, Pitek AP, Mallory E, et al. Disentangling the microbial ecological factors impacting honey bee susceptibility to Paenibacillus larvae infection. Trends Microbiol 2023;31:521-34.
Cite This Article
How to Cite
Download Citation
Export Citation File:
Type of Import
Tips on Downloading Citation
Citation Manager File Format
Type of Import
Direct Import: When the Direct Import option is selected (the default state), a dialogue box will give you the option to Save or Open the downloaded citation data. Choosing Open will either launch your citation manager or give you a choice of applications with which to use the metadata. The Save option saves the file locally for later use.
Indirect Import: When the Indirect Import option is selected, the metadata is displayed and may be copied and pasted as needed.
About This Article
Special Issue
Copyright
Data & Comments
Data
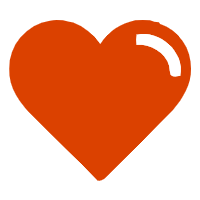
Comments
Comments must be written in English. Spam, offensive content, impersonation, and private information will not be permitted. If any comment is reported and identified as inappropriate content by OAE staff, the comment will be removed without notice. If you have any queries or need any help, please contact us at [email protected].