Dependence of microwave dielectric properties on ceramic connectivity in ultralow-εr Al2O3 porous ceramics
Abstract
Introducing pores is an effective approach for fabricating ultralow-εr ceramics; however, it is still unclear how the microstructures affect the microwave dielectric properties. In the present work, Al2O3-A and Al2O3-B porous ceramics were prepared by incomplete sintering at 1,000-1,650 °C and sintering at 1,650 °C with a porogen, respectively, so that the effects of porosity and ceramic connectivity can be clarified. The introduction of pores led to a significant decrease in εr, Qf, and |τf|, and Al2O3-B ceramic exhibits a slightly higher εr, a larger |τf|, and a much higher Qf value compared to the Al2O3-A counterpart with a similar relative density. For Al2O3-A, increasing the sintering temperature and relative density notably enhanced ceramic connectivity, while Al2O3-B's ceramic connectivity remained relatively insensitive to density variations. The improved ceramic connectivity in Al2O3-B enhanced the contribution of the ceramic phase to εr and τf, while also increased the Qf value by reducing ceramic-pore interfaces. Notably, Al2O3-B, with a low relative density of 48.31%, demonstrated a good combination of microwave dielectric properties, with εr = 4.16, Qf = 38,400 GHz, and τf = -44.3 ppm/°C. These findings reveal the strong dependence of microwave dielectric properties on ceramic connectivity in porous ceramics, and also inspire the development of ultralow-εr materials by regulating both the porosity and ceramic connectivity.
Keywords
INTRODUCTION
In recent years, the low-dielectric-constant (εr < 10) and ultralow-εr (εr < 5) microwave dielectric ceramics have attracted more and more attention mainly due to the rapid development of microwave communication technology toward higher working frequencies, especially millimeter-wave frequency band[1-4]. On one hand, for the electromagnetic wave propagating in a dielectric substrate with the length of L, the phase delay is
In the past decades, many low-εr microwave dielectric ceramics have been developed, including borates[6-8], silicates[9-12], aluminates[13-15], phosphates[16-18], molybdates[19,20], fluorides[21], and etc. However, the εr is higher than 5 for most of them, and very limited dense inorganic materials exhibit the εr lower than 5, such as SiO2 polymorphs[22,23], borosilicate glass[24], and cordierite[25,26]. Till now, amorphous SiO2 with the εr of around 3.8 is still the most important and irreplaceable ultralow-εr inorganic material[23]. The embarrassing situation for the research and application of ultralow-εr inorganic materials is attributed to the higher ionic polarization and higher density in comparison with organic materials, both of which tend to increase the εr[4]. Therefore, developing dense inorganic materials with ultralow εr is a big challenge. An effective approach to reducing the εr of ceramics is to introduce pores, forming porous ceramics[27-32], which can be regarded as “ceramic-air” composites. Since air has a very low εr of around 1 and is nearly lossless, porous ceramics offer a means to widely regulate the three key parameters of microwave dielectric properties: εr, Qf value, and temperature coefficient of resonant frequency (τf)[2,3]. Moreover, introducing air into microwave dielectric ceramics can lighten microwave devices, lower costs, and improve portability[33,34].
Although extensive research has been conducted on porous microwave dielectric ceramics, most studies have primarily focused on controlling porosity and its effects on microwave dielectric properties[27,31,35], while other microstructural factors have often been overlooked. Among these factors, phase connectivity is an important microstructural factor, as it significantly influences the properties of composites and porous materials, such as mechanical properties[36], electrical and thermal conductivities[37], and piezoelectric properties[38]. Our recent work revealed that the ceramic connectivity is also very important for the dielectric properties of BaTiO3-epoxy composites[39] and CaTiO3 porous ceramics[32] with high εr, since it dominates the electric field distributed in the constituting phases with the εr differing much from each other. For the low-εr and ultralow-εr porous ceramics, the difference between the εr of constituting phases (ceramic and air) becomes much less, so the effects of ceramic connectivity may be quite different. Furthermore, previous studies on porous ceramics have mainly addressed dielectric constant and dielectric loss, while little attention has been given to τf, a key parameter that determines the temperature stability of microwave dielectric ceramics. Therefore, it is not only of scientific significance but also important for practical application to systematically investigate how porosity and ceramic connectivity affect the εr, Qf value, and τf of low-εr and ultralow-εr porous ceramics.
Al2O3 ceramic is an important low-εr microwave dielectric ceramic, which exhibits a low εr around 10, a high Qf value of 330,000-450,000 GHz, and a relatively large negative τf of about -60 ppm/°C[27,40-42].
MATERIALS AND METHODS
Fine α-Al2O3 powder with an average particle size of 200 nm (> 99.9%, Sinopharm Chemical Reagent Co., Ltd.) was used for preparing Al2O3 porous ceramics. The raw powder was dried and mixed with 5 wt% polyvinyl alcohol solution as the binder. The powder was uniaxially pressed into compacts with 12 mm in diameter under 100 MPa for 1 min. The compacts were then sintered at different temperatures of 1,000-1,650 °C for 3 h in air with a heating rate of 5 °C/min and a cooling rate of 2 °C/min, and the as-prepared ceramics were denoted by Al2O3-A. For Al2O3-B porous ceramics, Al2O3 powder was mixed with 20-60 vol% NH4HCO3 powder (AR, Sinopharm Chemical Reagent Co., Ltd.) as the porogen because NH4HCO3 can be decomposed completely at low temperatures without any residual or reaction with Al2O3, and thus does not affect the sintering characteristics. The mixed powder was also pressed into compacts, which were sintered at the optimum temperature of 1,650 °C for 3 h after heating at 70 °C for 3 h to decompose NH4HCO3 and form large pores.
The bulk density and total porosity of the samples were measured using the geometric method. The open porosity was measured by the Archimedes drainage method, and the closed porosity was calculated from the total porosity and open porosity. The phase constitution of the Al2O3 raw powder and porous ceramics was identified by X-ray diffraction (XRD) using Cu Kα Radiation (Rigaku 2550/PC, Rigaku, Tokyo, Japan). The microstructure on the fractured surfaces of Al2O3-A and Al2O3-B ceramics was observed by field emission scanning electron microscopy (SEM, GeminiSEM 300, Carl Zeiss Microscopy GmbH, Germany). The Vickers hardness was measured by a Vickers indentation tester (MHVD-5IS, Shanghai Jujing Precision Instrument Manufacturing), and the measurement for Al2O3-B porous ceramics was conducted in the regions far from the large pores. The cylindrical samples with 9.5-12 mm in diameter and 3.8-6 mm in thickness were used to measure the microwave dielectric properties using a vector network analyzer (Agilent E8363B, Agilent Technologies Inc., Santa Clara, CA). The εr and Qf were measured at
RESULTS AND DISCUSSION
Figure 1 shows the relative density and porosities of Al2O3-A ceramics as functions of sintering temperature (Ts). With increasing Ts from 1,000 to 1,300 °C, the relative density of Al2O3-A ceramics increases slowly from 41.25% to 46.15%, and the trend speeds up for higher sintering temperatures. The closed porosity is within 5% for all the Al2O3-A ceramics, so the total porosity is dominated by the open one. The highest relative density of 91.73% is obtained for the sintering temperature of 1,650 °C. Therefore, the Al2O3-B ceramics are sintered at the optimum temperature of 1,650 °C, and Figure 1B shows the relative density and porosities of Al2O3-B ceramics as functions of NH4HCO3 volume fraction in the green compacts (VNH4HCO3). The relative density of Al2O3-B ceramics decreases with VNH4HCO3, and it can be tuned significantly from 78.76% to 48.31% by increasing VNH4HCO3 from 20% to 60%. The decomposition of NH4HCO3 particles produces large pores, which tend to be separated from one another and, therefore, increase the closed porosity for the low VNH4HCO3. When VNH4HCO3 is high, however, the NH4HCO3 particles may contact the neighbors, and the decomposition of contacting particles results in the interconnected network of the formed large pores. Therefore, the closed porosity first increases and then decreases with VNH4HCO3, although the total porosity always increases for Al2O3-B ceramics, as shown in Figure 1B. The XRD patterns of Al2O3 raw powder and some typical Al2O3-A and Al2O3-B ceramics are shown in Supplementary Figure 1. These XRD patterns are almost the same, and all the peaks can be indexed by α-Al2O3 (PDF card: 97-003-3639), indicating that the sintering temperature and porosity have no effect on the phase constitution and crystal structure.
The SEM images of the fractured surfaces of Al2O3-A [Figure 2] and Al2O3-B [Figure 3] ceramics clearly reveal the significant difference between the microstructures of the two groups of Al2O3 ceramics. When the sintering temperature is as low as 1,000 °C, the small and rounded particles with a size of around 200 nm and their aggregations are observed in Al2O3-A, which inherits the microstructure of Al2O3 raw powder. The microstructure does not change much at a sintering temperature of 1,200 °C, except that the aggregations become more clear, which is consistent with the slowly increasing relative density shown in Figure 1A and indicates the initial stage of sintering. A significant microstructural evolution can be observed at a sintering temperature of 1,400 °C, at which the original particles with round shapes disappear. Instead, the particles become a little larger and their edges are somewhat sharp, indicating the appearance of initial grains. Furthermore, the initial grains are bonded to some extent and the sintering necks between grains appear, although a lot of small pores seem to hinder the bonding of grains. With further increase in the sintering temperature up to 1,600 °C, the grains with sharp edges and the bonding between neighbored grains become more distinct, together with significant grain growth, clear grain boundaries, and exclusion of pores. Here, connectivity is employed to represent the phase continuity or degree of interconnection within a phase. For the present Al2O3 porous ceramics, the ceramic connectivity refers to the extent of bonding between Al2O3 particles or grains. Therefore, the above discussion reveals that the connectivity of ceramic phase is enhanced with relative density when the sintering temperature increases from 1,000 to 1,600 °C. The microstructure changes little at a higher sintering temperature of 1,650 °C, except that the pores continue to decrease, indicating the final stage of sintering. Additionally, the average grain size increases with sintering temperature from 0.44 to 4.23 um, as shown in Figure 4, and the grain size distribution is illustrated in Supplementary Figure 2. In comparison, the microstructure changes very differently with relative density for Al2O3-B ceramics, as shown in Figure 3. The large pores with the size of several to tens of micrometers are observed in Al2O3-B ceramics, which are formed by decomposing the NH4HCO3 large particles as the porogen. As discussed above, the relative density of Al2O3-B ceramics decreases significantly from 78.76% to 48.31% by adjusting the NH4HCO3 volume fraction from 20% to 60%. Interestingly, the microstructure far from the large pores in Al2O3-B ceramics depends little on relative density and is similar to that of Al2O3-A ceramic sintered at 1,650 °C. Since the porogen of NH4HCO3 is decomposed completely at only 70 °C without any residual or reaction with Al2O3, it is expected to have a limited effect on the sintering characteristics and microstructure of Al2O3-B ceramics except the produced large pores. Therefore, the ceramic connectivity is dominated by the sintering temperature rather than the porogen volume fraction, and the ceramic connectivity is similar for all the Al2O3-B ceramics. Moreover, the ceramic connectivity in Al2O3-B porous ceramic is significantly improved compared to that in Al2O3-A counterpart with a similar density. For example, the ceramic connectivity in Figure 3C is much better than that in Figure 2C, although the corresponding relative density of Al2O3-B (48.31%) is a little lower than that of
Figure 2. SEM images of fractured surfaces of Al2O3-A ceramics sintered at (A) 1,000 °C, (B) 1,200 °C, (C) 1,400 °C, (D) 1,450 °C, (E) 1,500 °C, (F) 1,550 °C, (G) 1,600 °C, and (H) 1,650 °C.
Figure 3. SEM images of fractured surfaces of Al2O3-B ceramics with relative densities of (A) 78.76%, (B) 65.29%, and (C) 48.31%. The insets show the fractured surfaces with higher magnification.
Figure 6A shows the εr of Al2O3-A ceramics sintered at different temperatures. With increasing Ts from 1,000 to 1,300 °C, the εr increases slightly from 3.00 to 3.49, and it increases more rapidly to 8.99 with further increasing Ts up to 1,650 °C, which is consistent with the relative density shown in Figure 1. The relationship between εr and relative density for Al2O3-A ceramics can be observed more clearly in Figure 6B, where the εr increases monotonously and smoothly with relative density. Figure 6B also shows that the εr of Al2O3-B ceramics exhibits similar dependence on relative density to Al2O3-A counterparts, while the εr of the former is always slightly higher than that of the latter with a similar relative density, and the difference is larger for the lower relative density. As discussed above, the Al2O3-B ceramic exhibits a larger grain size and improved ceramic connectivity than the Al2O3-A counterpart with a similar density because of the higher sintering temperature. Since the low-loss microwave dielectric ceramics are generally non-polar materials, their εr is determined by the electric and ionic polarizations and, hence, insensitive to grain size. Therefore, the larger εr of Al2O3-B ceramics is attributed to better ceramic connectivity. Such results are consistent with those in CaTiO3 porous ceramic[32], although the difference in εr between the two groups of Al2O3 ceramics is not so dramatic in the present work. Since CaTiO3 has a high εr of 183[32] and the difference between the εr of the ceramic phase and air is much larger for the CaTiO3 porous ceramics, the electric field distribution in the constituting phases is more sensitive to the continuity of ceramic phase, leading to the much stronger dependence of εr on the ceramic connectivity. Moreover, the ultralow εr (< 5) can be obtained in both Al2O3-A and Al2O3-B ceramics when the relative density is lower than 60%, proving the feasibility of achieving ultralow εr by introducing pores into low-εr ceramics.
Figure 6. (A) εr of Al2O3-A ceramics sintered at different Ts. (B) εr of Al2O3-A and Al2O3-B ceramics with different relative densities and the predicted εr by several dielectric mixing rules.
It is well known that the εr of dielectric composites and porous materials can be predicted by many dielectric mixing rules, including parallel model, serial model, logarithmic model[45], effective medium approximation (EMA)[46], Heidinger’s approximation[47], etc. Additionally, Bosman and Havinga’s correction[48] described by
is often used for predicting the dielectric constant of porous materials, where p is the porosity, εr,theo is the theoretical dielectric constant of the fully densified material, and εr is the measured dielectric constant of the material with the porosity p. Bosman and Havinga’s correction is usually reliable when the porosity is not high, and the theoretical dielectric constant of Al2O3 is calculated to be 10.12 from the measured εr (8.99) and porosity (8.27%) of Al2O3-A ceramic sintered at 1,650 °C, which is used for the following prediction. Figure 6B shows the predicted εr of Al2O3 porous ceramics with different relative densities from these dielectric mixing rules. The predicted results by the parallel model, serial model, logarithmic model, and Bosman and Havinga’s correction differ much from the experimental ones, especially for the low relative density. Although Heidinger’s approximation and effective medium approximation provide much better prediction, they still fail to explain the difference between Al2O3-A and Al2O3-B porous ceramics. Such a failure is not surprising, since all the above mixing rules are empirical or based on some assumptions in microstructure, and only the relative density acts as the independent variable. However, the above discussion on microstructure reveals the significant difference in microstructure and ceramic connectivity between Al2O3-A and Al2O3-B ceramics, which should be considered for a more reliable prediction.
Our previous work shows that the parameter α in the exponential model
can be used to describe the connectivity of constituting phases in dielectric composites and porous materials[32], where V is the volume fraction, and the numeric subscripts mean phases 1 and 2. The volume fraction of ceramic phase (phase 1) is just the relative density for porous ceramics, and phase 2 is air with εr,2 = 1 and V2 = 1 - V1, so Eq. (2) can be rewritten as
The fitted parameter α is calculated for the present Al2O3-A and Al2O3-B ceramics from the measured εr and relative density, and the calculated α as a function of relative density is shown in Figure 7. With increasing
Since Al2O3 has a negative τf, all the Al2O3-A and Al2O3-B ceramics in the present work exhibit negative τf, as shown in Figure 8A and B. With increasing Ts from 1,000 to 1,650 °C and relative density from 41.25% to 91.73% for Al2O3-A, τf decreases significantly from -15.8 to -58.5 ppm/°C. In comparison, τf decreases more slightly with relative density for Al2O3-B, and the difference between the τf values for the relative densities of 48.31% and 91.73% is only 12.7 ppm/°C. Similarly, the parallel model, serial model, and logarithmic model fail in predicting the τf of Al2O3 porous ceramics. Since εr is independent of grain size for low-loss microwave dielectric ceramics, as discussed above, its temperature coefficient (τε) and, hence, the τf are also insensitive to grain size. Therefore, it can be inferred that τf is also strongly dependent on ceramic connectivity. The expression for the τε of a dielectric composite can be obtained by derivating Equation 2 with respect to temperature as[32]
Figure 8. (A) τf of Al2O3-A ceramics sintered at different Ts. (B) τf of Al2O3-A and Al2O3-B ceramics with different relative densities, and the predicted τf by Equation 6 and other dielectric mixing rules.
In the present work, phase 2 is air with V2 = 1 - V1, εr,2 = 1, and τε,2= 0 for Al2O3 porous ceramics. Combining with
for both the porous and fully dense Al2O3 ceramics, the τf of the Al2O3 porous ceramics can be obtained as
By using τf,1 = -60.2 ppm/°C and αL,1 = 8.1 ppm/°C for fully dense Al2O3 ceramic and assuming that the αL of porous ceramics is proportional to relative density, the τf of Al2O3-A and Al2O3-B porous ceramics can be predicted from the relative density and α, and the results are also shown in Figure 8B. The predicted τf decreases with relative density for both Al2O3-A and Al2O3-B ceramics, and Al2O3-A exhibits a higher predicted τf than Al2O3-B with similar density, which is consistent with the experimental results and attributed to the poorer ceramic connectivity and lower α for the former. The predicted τf values fit the experiments well for all the Al2O3-B porous ceramics and the Al2O3-A counterparts with high relative density. However, when the relative density of Al2O3-A ceramics is lower than 60%, the difference between the predicted and experimental results becomes large, indicating that the exponential model is more suitable for predicting the τf of Al2O3 porous ceramics with relatively good ceramic connectivity. Similarly to εr, the effect of ceramic connectivity on the τf of Al2O3 porous ceramics is not so dramatic as that for CaTiO3[32] because of the much lower εr of Al2O3 and less sensitive electric field distribution to the continuity of ceramic phase. Additionally, it is well known that the low-εr microwave dielectric ceramics usually exhibit large negative τf values that deteriorate the temperature stability for practical application. The above discussion shows that the pores introduced in microwave dielectric ceramics can not only decrease the dielectric constant, but also improve the temperature stability.
As discussed above, the εr and τf of Al2O3 porous ceramics are directly determined by the relative density and ceramic connectivity. In comparison, the Qf value of porous ceramics is more complex since many microstructural factors may result in extrinsic dielectric loss and thus decrease the Qf value. Without considering the effects of microstructural factors, the Qf value of a composite can be expressed as
from the exponential rule[32]. For the porous ceramics, phase 2 is air with V2 = 1 - V1, εr,2 = 1, and tanδ2 = 0, so the theoretical Qf value of porous ceramics is
By using εr,1 = 10.12 and Qf1 = 402,000 GHz, one can find in Figure 9A that the predicted Qf value of Al2O3 porous ceramics increases as the relative density decreases, since the decreased relative density means the decreased content of lossy Al2O3 and increased content of lossless air. However, the measured Qf values of Al2O3 porous ceramics are always much lower than that of the dense Al2O3 ceramic and the predicted Qf values. Such a contradiction is not surprising since the Qf value of Al2O3 itself is very high, and the extrinsic dielectric loss caused by microstructural factors may greatly exceed the ultra-low dielectric loss of Al2O3 and hence dominate the dielectric loss and Qf value of Al2O3 porous ceramics. Moreover, the Al2O3-B ceramics exhibit similar Qf values of around 40,000 GHz, which are insensitive to the varying relative density from 48.31% to 78.76%, and 1.5-4 times higher than those of the Al2O3-A ceramics with the similar relative densities. Differently from Al2O3-B, the Qf value is strongly dependent on the relative density for Al2O3-A porous ceramics. Such results further verify the dominant role of the microstructural factors. Supplementary Figure 4 also shows the calculated dielectric loss of Al2O3-A porous ceramics at 13 GHz as a function of relative density. Since the Qf value is defined as the product of frequency and reciprocal dielectric loss, the dielectric loss shows a reverse trend to the Qf value. As analyzed from the SEM images, the grain size and ceramic connectivity are the main microstructural factors for the Al2O3-A and Al2O3-B porous ceramics. For Al2O3-A, both the grain size and ceramic connectivity increase with higher sintering temperatures and relative densities. In contrast, for Al2O3-B, these factors remain largely unaffected by changes in relative density. The increase in grain size generally increases the dielectric loss and decreases the Qf value for the dense Al2O3 ceramics[27], which may be responsible for the decreasing Qf value of Al2O3-A ceramics with increasing sintering temperature from 1,000 to 1,300 °C and relative density from 41.25% to 46.15%, as shown in Figure 9B. However, the negative effect of grain size on Qf value is inconsistent with the experimental results for the Al2O3-A ceramics with higher relative densities and Al2O3-B ceramics, which are much more important in the present work. It can be inferred that the Qf value of these ceramics is predominantly determined by the ceramic connectivity, as the ceramic-pore interfaces introduce extrinsic dielectric loss through microwave scattering, and these interfaces can be greatly decreased by enhancing the ceramic connectivity.
Figure 9. (A) Experimental and predicted Qf values of Al2O3-A and Al2O3-B ceramics with different relative densities. (B)Qf value of Al2O3-A ceramics sintered at different Ts.
By integrating the above discussion on the εr, τf, and Qf value, the optimum microwave dielectric properties with εr = 4.16, Qf = 38,400 GHz, and τf = -44.3 ppm/°C are obtained in Al2O3-B ceramic with a low relative density of 48.31%, indicating that the microwave dielectric properties of ultralow-εr porous ceramics can be optimized by regulating both the porosity and ceramic connectivity. Furthermore, the microstructural effects revealed in the present work provide valuable guidance for the future development of ultralow-εr porous ceramics. Considering the substantial impact of ceramic connectivity on the Qf value of porous ceramics, good ceramic connectivity is essential for achieving high Qf values. However, the enhanced ceramic connectivity also leads to the increase in both εr and |τf|, so it is crucial to select the ceramics with both low εr and small τf in the dense state for obtaining the ultra-low εr and improving the temperature stability.
CONCLUSIONS
In conclusion, two groups of Al2O3 porous ceramics are prepared by incomplete sintering at low temperatures and sintering at the optimal temperature with the introduction of NH4HCO3 porogen, respectively. The introduction of pores leads to a significant decrease in εr, Qf, and |τf|, and Al2O3-B ceramic exhibits a slightly higher εr, a larger |τf|, and a much higher Qf value than Al2O3-A with similar density. The microstructural analysis clearly reveals that the ceramic connectivity and relative density of Al2O3-A porous ceramics are improved significantly with increasing sintering temperature, while the excellent ceramic connectivity of Al2O3-B changes little with the porogen content and relative density. The ceramic connectivity is dominated by sintering temperature rather than relative density, and can be described by the parameter α in the exponential rule, which increases significantly with relative density for Al2O3-A but changes little for Al2O3-B. The significantly improved ceramic connectivity in Al2O3-B porous ceramics enhances the contribution of ceramic phase to the εr and τf of porous ceramics, although such effects are not so dramatic as those in CaTiO3 porous ceramics because of the much lower εr of Al2O3. Moreover, the improved ceramic connectivity increases the Qf value by decreasing the ceramic-pore interfaces that cause extrinsic dielectric loss. The good combination of microwave dielectric properties with εr = 4.16,
DECLARATIONS
Authors’ contributions
Made substantial contributions to the conception and design of the study: Li, L.; Wu, S. Y.
Performed data analysis and interpretation: Yan, X. J.; Cao, M.; Li, L.
Performed data acquisition: Yan, X. J.
Provided administrative, technical, and material support: Li, L.; Chen, X. M.
Availability of data and materials
The data that support the findings of this study are available from the corresponding author upon reasonable request.
Financial support and sponsorship
This work was supported by the National Natural Science Foundation of China under Grant Nos. 52272128 and U20A20243.
Conflicts of interest
All authors declared that there are no conflicts of interest.
Ethical approval and consent to participate
Not applicable.
Consent for publication
Not applicable.
Copyright
© The Author(s) 2025.
Supplementary Materials
REFERENCES
1. Rappaport, T. S.; Sun, S.; Mayzus, R.; et al. Millimeter wave mobile communications for 5G cellular: It will work! IEEE. Access. 2013, 1, 335-49.
2. Sebastian, M. T.; Ubic, R.; Jantunen, H. Low-loss dielectric ceramic materials and their properties. Int. Mater. Rev. 2015, 60, 392-412.
3. Raveendran, A.; Sebastian, M. T.; Raman, S. Applications of microwave materials: a review. J. Electron. Mater. 2019, 48, 2601-34.
4. Li, L.; Zhu, X. L.; Chen, X. M. Where can the low dielectric constant go in dense inorganic materials? J. Materiomics. 2023, 9, 980-3.
5. Reaney, I. M.; Iddles, D. Microwave dielectric ceramics for resonators and filters in mobile phone networks. J. Am. Ceram. Soc. Soc. 2006, 89, 2063-72.
6. Došler, U.; Kržmanc, M. M.; Suvorov, D. The synthesis and microwave dielectric properties of Mg3B2O6 and Mg2B2O5 ceramics. J. Eur. Ceram. Soc. 2010, 30, 413-8.
7. Chang, S.; Pai, H.; Tseng, C.; Tsai, C. Microwave dielectric properties of ultra-low temperature fired Li3BO3 ceramics. J. Alloy. Compd. 2017, 698, 814-8.
8. Zhou, D.; Pang, L.; Wang, D.; Qi, Z.; Reaney, I. M. High quality factor, ultralow sintering temperature Li6B4O9 microwave dielectric ceramics with ultralow density for antenna substrates. ACS. Sustain. Chem. Eng. 2018, 6, 11138-43.
9. Sun, H.; Zhang, Q.; Yang, H.; Zou, J. (Ca1-xMgx)SiO3: a low-permittivity microwave dielectric ceramic system. Mater. Sci. Eng. B. 2007, 138, 46-50.
10. Bian, J.; Xie, Y. Sintering behavior and dielectric properties of SiO2-BPO4 glass-fluxed ceramics. J. Eur. Ceram. Soc. 2018, 38, 2747-52.
11. Kamutzki, F.; Schneider, S.; Barowski, J.; Gurlo, A.; Hanaor, D. A. Silicate dielectric ceramics for millimetre wave applications. J. Eur. Ceram. Soc. 2021, 41, 3879-94.
12. Hou, H.; Zhang, A.; Yang, H.; et al. LiGaSiO4: an ultra-low permittivity dielectric material enabling application in patch antenna. Ceram. Int. 2024, 50, 7758-66.
13. Surendran, K.; Bijumon, P.; Mohanan, P.; Sebastian, M. (1-x)MgAl2O4-xTiO2 dielectrics for microwave and millimeter wave applications. Appl. Phys. A. 2005, 81, 823-6.
14. Wan Jalal, W. N.; Abdullah, H.; Zulfakar, M. S.; Bais, B.; Shaari, S.; Islam, M. T. ZnAl2O4-based microwave dielectric ceramics as GPS patch antenna: a review. Trans. Indian. Ceram. Soc. 2013, 72, 215-24.
15. Xie, M.; Li, X.; Lai, Y.; et al. Phase evolution and microware dielectric properties of high-entropy spinel-type
16. Bian, J. J.; Kim, D. W.; Hong, K. S. Microwave dielectric properties of A2P2O7 (A = Ca, Sr, Ba; Mg, Zn, Mn). Jpn. J. Appl. Phys. 2004, 43, 3521.
17. Thomas, D.; Abhilash, P.; Sebastian, M. T. Casting and characterization of LiMgPO4 glass free LTCC tape for microwave applications. J. Eur. Ceram. Soc. 2013, 33, 87-93.
18. Bian, J.; Sun, X.; Xie, Y. Structural evolution, sintering behavior and microwave dielectric properties of Al(1-x)(Si0.5Zn0.5)xPO4 ceramics. J. Eur. Ceram. Soc. 2019, 39, 4139-43.
19. Zhou, D.; Randall, C. A.; Wang, H.; Pang, L.; Yao, X. Microwave dielectric ceramics in Li2O-Bi2O3-MoO3 system with ultra-low sintering temperatures. J. Am. Ceram. Soc. 2010, 93, 1096-100.
20. Varghese, J.; Siponkoski, T.; Nelo, M.; Sebastian, M. T.; Jantunen, H. Microwave dielectric properties of low-temperature sinterable
21. Song, X.; Du, K.; Li, J.; et al. Low-fired fluoride microwave dielectric ceramics with low dielectric loss. Ceram. Int. 2019, 45, 279-86.
22. Krupka, J.; Derzakowski, K.; Tobar, M.; Hartnett, J.; Geyer, R. G. Complex permittivity of some ultralow loss dielectric crystals at cryogenic temperatures. Meas. Sci. Technol. 1999, 10, 387-92.
23. Li, L.; Fang, Y.; Xiao, Q.; Wu, Y. J.; Wang, N.; Chen, X. M. Microwave dielectric properties of fused silica prepared by different approaches. Int. J. Appl. Ceram. Technol. 2014, 11, 193-9.
24. Akkasoglu, U.; Sengul, S.; Arslan, İ.; Ozturk, B.; Cicek, B. Structural, thermal and dielectric properties of low-alkali borosilicate glasses for electronic applications. J. Mater. Sci. Mater. Electron. 2021, 32, 22629-36.
25. Wu, S.; Song, K.; Liu, P.; et al. Effect of TiO2 doping on the structure and microwave dielectric properties of cordierite ceramics. J. Am. Ceram. Soc. 2015, 98, 1842-7.
26. Lou, W.; Mao, M.; Song, K.; et al. Low permittivity cordierite-based microwave dielectric ceramics for 5G/6G telecommunications. J. Eur. Ceram. Soc. 2022, 42, 2820-6.
27. Penn, S. J.; Alford, N. M.; Templeton, A.; et al. Effect of porosity and grain size on the microwave dielectric properties of sintered alumina. J. Am. Ceram. Soc. 1997, 80, 1885-8.
28. Jin, F.; Tong, H.; Shen, L.; Wang, K.; Chu, P. K. Micro-structural and dielectric properties of porous TiO2 films synthesized on titanium alloys by micro-arc discharge oxidization. Mater. Chem. Phys. 2006, 100, 31-3.
29. Xia, Y.; Zeng, Y.; Jiang, D. Dielectric and mechanical properties of porous Si3N4 ceramics prepared via low temperature sintering. Ceram. Int. 2009, 35, 1699-703.
30. Hou, Z.; Ye, F.; Liu, L. Effects of pore shape and porosity on the dielectric constant of porous β-SiAlON ceramics. J. Eur. Ceram. Soc. 2015, 35, 4115-20.
31. Chen, Y.; Guo, W.; Luo, Y.; Ma, Z.; Zhang, L.; Yue, Z. Microwave and terahertz properties of porous Ba4(Sm,Nd,Bi)28/3Ti18O54 ceramics obtained by sacrificial template method. J. Am. Ceram. Soc. 2021, 104, 5679-88.
32. Cao, M.; Li, L.; Wu, S. Y.; Chen, X. M. Dominant role of ceramic connectivity in microwave dielectric properties of porous ceramics. Acta. Mater. 2023, 258, 119207.
33. Xu, Y.; Tsai, Y.; Tu, K. N.; et al. Dielectric property and microstructure of a porous polymer material with ultralow dielectric constant. Appl. Phys. Lett. 1999, 75, 853-5.
34. Dong, X.; Hu, Y.; Zhao, J.; Wu, Y. Method to predict effective dielectric constant of porous silica low dielectric constant materials. Phys. B. 2010, 405, 3551-4.
35. Luo, W.; Guo, J.; Randall, C.; Lanagan, M. Effect of porosity and microstructure on the microwave dielectric properties of rutile. Mater. Lett. 2017, 200, 101-4.
36. Meng, F.; Fu, Z.; Zhang, J.; et al. Study on the structure and properties of fine-grained alumina fast sintered with high heating rate. Mater. Res. Bull. 2008, 43, 3521-8.
37. Li, L.; Deng, Y.; Chen, G. Status and prospect of garnet/polymer solid composite electrolytes for all-solid-state lithium batteries. J. Energy. Chem. 2020, 50, 154-77.
38. Levassort, F.; Lethiecq, M.; Desmare, R.; Hue, T. H. Effective electroelastic moduli of 3-3(0-3) piezocomposites. IEEE. Trans. Ultrason. Ferroelectr. Freq. Control. 1999, 46, 1028-34.
39. Cao, M.; Yan, X. J.; Li, L.; Wu, S. Y.; Chen, X. M. Obtaining greatly improved dielectric constant in BaTiO3-epoxy composites with low ceramic volume fraction by enhancing the connectivity of ceramic phase. ACS. Appl. Mater. Interfaces. 2022, 14, 7039-51.
40. Alford, N. M.; Penn, S. J. Sintered alumina with low dielectric loss. J. Appl. Phys. 1996, 80, 5895-8.
41. Penn, S.; Poole, M.; Breeze, J.; Alford, N. Layered Al2O3-TiO2 composite dielectric resonators with tuneable temperature coefficient for microwave applications. IEE. Proc. Sci. Meas. Technol. 2000, 147, 269-73.
42. Kolodiazhnyi, T.; Annino, G.; Spreitzer, M.; et al. Development of Al2O3-TiO2Al2O3-TiO2 composite ceramics for high-power millimeter-wave applications. Acta. Mater. 2009, 57, 3402-9.
43. Mollá, J.; González, M.; Vila, R.; Ibarra, A. Effect of humidity on microwave dielectric losses of porous alumina. J. Appl. Phys. 1999, 85, 1727-30.
44. Kajfez, D.; Gundavajhala, A. Measurement of material properties with a tunable resonant cavity. Electron. Lett. 1993, 29, 1936-7.
45. Zakri, T.; Laurent, J.; Vauclin, M. Theoretical evidence for `Lichtenecker's mixture formulae' based on the effective medium theory. J. Phys. D. Appl. Phys. 1998, 31, 1589-94.
46. Stroud, D. Generalized effective-medium approach to the conductivity of an inhomogeneous material. Phys. Rev. B. 1975, 12, 3368-73.
47. Heidinger, R.; Nazare, S. Influence of porosity on the dielectric properties of AlN in the range of 30. 40 GHz. Powder. Metall. Int. 1988, 20, 30-2. Available from: https://www.osti.gov/etdeweb/biblio/6526366 [Last accessed on 6 May 2025]
Cite This Article

How to Cite
Download Citation
Export Citation File:
Type of Import
Tips on Downloading Citation
Citation Manager File Format
Type of Import
Direct Import: When the Direct Import option is selected (the default state), a dialogue box will give you the option to Save or Open the downloaded citation data. Choosing Open will either launch your citation manager or give you a choice of applications with which to use the metadata. The Save option saves the file locally for later use.
Indirect Import: When the Indirect Import option is selected, the metadata is displayed and may be copied and pasted as needed.
About This Article
Special Issue
Copyright
Data & Comments
Data
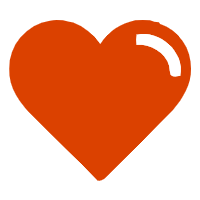
Comments
Comments must be written in English. Spam, offensive content, impersonation, and private information will not be permitted. If any comment is reported and identified as inappropriate content by OAE staff, the comment will be removed without notice. If you have any queries or need any help, please contact us at [email protected].