Control of exposed crystal planes of CeO2 enhances electrocatalytic nitrate reduction
Abstract
Cerium dioxide (CeO2) has emerged as a promising electrocatalyst for electrocatalytic nitrate reduction to produce ammonia (NRA). However, the NRA performance of CeO2 still needs to be improved and the interface-related NRA electrocatalytic activity of CeO2 is unclear. Herein, CeO2 with exposed (111) or (200)/(220) planes is prepared by adjusting the amount of added surfactant simply. The CeO2 with exposed (220)/(200) planes presents higher NRA performance than that of CeO2 with the exposed (111) plane. Based on density functional theory, the enhanced mechanism is revealed. The exposed (111) plane of CeO2 repels
Keywords
INTRODUCTION
Nitrate pollution poses a major threat to water resources and ecosystems, leading to adverse health effects and ecological imbalances[1,2]. By utilizing electrocatalytic reduction, nitrate can be converted into ammonia (NH3) efficiently, which is widely used as a fertilizer and precursor for various industrial processes[3,4]. This approach offers a promising alternative to traditional methods of NH3 production, such as the energy-intensive Haber-Bosch process which consumes large amounts of fossil fuels and emits greenhouse gases[5-7]. The electrocatalytic and thermal catalytic nitrate reduction to produce NH3 (NRA) provides a greener and sustainable pathway for NH3 production but also presents from nitrate pollution[8-10]. In contrast, thermal catalytic NRA requires a hydrogen source, leading to high complexity of the catalytic device. The electrocatalytic NRA operates with a simple experimental device driven by green electric energy, and it looks more promising. Nevertheless, due to the involvement of an eight-electron transfer, the electrocatalytic reaction exhibits complex reaction pathways and intermediates, and the competitive hydrogen evolution reaction (HER) weakens the electrocatalytic efficiency[11-13]. As a result, there is an urgent need to design electrocatalysts with high efficiency and selectivity for NH3 synthesis.
Cerium dioxide (CeO2), as a rare earth metal oxide, possesses a distinctive crystal structure, such as the flexible conversion between Ce4+ and Ce3+[14-16]. These unique characteristics contribute to its excellent redox properties and high oxygen mobility and make it highly valuable in various electrochemical fields[17-22]. Electrocatalytic procedures are interface reactions conducted on the exposed planes of electrocatalysts. Many works have found that catalytic performance is strongly dependent on the exposed crystal planes, because the exposed planes would interact with the reactants/intermediates resulting in different adsorption energy, Gibbs free energy and activity of competitive/side reactions[23,24]. Therefore, the NRA performance can be enhanced by controlling the exposed crystal planes. Moreover, the effect mechanism of different exposed crystal planes of CeO2 on the NRA processes remains ambiguous.
Herein, the exposed crystal planes of CeO2 are controlled by adjusting the quantity of cetyltrimethylammonium bromide (CTAB), and CeO2 with exposed (111) plane or (220)/(200) planes is prepared. The CeO2 with exposed (220)/(200) planes presents higher NRA performance than that of CeO2 with the (111) plane. Compared with the CeO2 with exposed (111) plane, the NH3 yield rate (YieldNH3) and Faraday efficiency (FE) of CeO2 with exposed (200)/(220) planes increase by 11.9% and 37.7%, respectively. Density functional theory (DFT) is used to reveal the underlying mechanism. This work paves a new pathway for designing high-performance NRA electrocatalysts.
EXPERIMENTAL DETAILS
Preparation of CeO2
First, 0.87 g of Ce(NO3)3·6H2O was dissolved in 60 mL of ethanol to obtain solution A. In a separate container, a specific amount of CTAB (0.5, 1.0, or 2.0 g), formic acid (575 uL), and dimethylamine
Characterization
X-ray diffraction (XRD) patterns were obtained using a D8 Advance diffractometer (Bruker, Germany) equipped with Cu Kα radiation. Scanning electron microscopy (SEM) images were acquired with a ZEISS MERLIN Compact microscope (Zeiss, Germany). The Brunauer-Emmett-Teller (BET) tests were performed with an ASAP2460 instrument (Micromeritics, USA). Transmission electron microscopy (TEM) images were captured using a FEI Tecnai G2 F20 microscope (FEI, USA). The thermogravimetric analysis (TGA) and differential scanning calorimetry (DSC) curves were obtained through testing on the TGA5500 instrument (TA, USA).
Electrochemical tests
Electrochemical tests were conducted using an Interface 1010E electrochemical workstation (Gamry, USA) with an H-type electrolytic cell. The three-electrode system comprised a sample as the working electrode, a platinum sheet as the counter electrode, and the Hg/HgO as the reference electrode. All measured potentials were converted to the reversible hydrogen electrode (RHE) scale, determined by E(RHE) = E(Hg/HgO) + 0.059 × pH + 0.098. To prepare the working electrode, a dispersion was created by combining 5 mg of the samples with 50 μL Nafion solution (5 wt%) in 450 μL of ethanol. Then, 100 μL droplets of the dispersion were carefully transferred onto 1 × 1 cm2 carbon paper. The loading mass of the working electrode was around 0.2 mg. For electrochemical testing, 50 mL electrolyte (0.1 M Na2SO4) was added to the anodic electrolytic cell, and 50 mL electrolyte (0.1 M Na2SO4 and NaNO3) was added to the cathodic electrolytic cell. The electrolyte type, pH and concentration setting is the same as the related work[25]. Before testing, argon gas was bubbled through the electrolyte to remove any traces of nitrogen. Linear sweep voltammetry (LSV) was performed with a scan rate of 10 mV/s, and potentiostatic testing was conducted at a constant potential for an hour. Subsequently, the electrolyte after the tests was subjected to UV-Vis light analysis to calculate the YieldNH3 and FE. The stirring rate remained constant for all tests.
RESULTS AND DISCUSSION
Figure 1A-C presents the SEM images of the samples; it is evident that the samples predominantly consist of nearly spherical agglomerates. These agglomerates exhibit a wide size distribution, ranging from around 100 to 400 nm. Additionally, with increasing amounts of CTAB, no significant change in the morphology and size of agglomerates indicates that the amount of CTAB has little effect on its morphology and size. The XRD patterns show that the precursor material is cerium formate, which transforms into CeO2 upon calcination [Figure 1D]. The peaks at 28.6°, 33.2°, 47.6°, 56.5°, 59.2°, 69.4°, 76.9°, 79.3° and 88.6° correspond to (111), (200), (220) (311), (222), (400), (331), (420) and (422) planes of CeO2 (PDF 34#0394), respectively. Figure 1E and F demonstrates the N2 adsorption/desorption isotherm curves and pore size distribution curves of samples. Based on the classification of International Union of Pure and Applied Chemistry (IUPAC) adsorption isotherms, those curves correspond to the type III adsorption model indicating the porous nature of samples[26]. BET specific surface areas of CeO2-CTAB0.5, CeO2-CTAB1.0, and CeO2-CTAB2.0 are 83, 97, and 87 m²/g, respectively, and the average pore diameters calculated by the Barrett-Joyner-Halenda (BJH) method are 16.3, 16.0 and 13.8 nm, respectively. Therefore, adding CTAB does not significantly influence the morphology, size and specific surface area of CeO2, and its effect on the NRA performance of CeO2 can be disregarded.
Figure 1. SEM image of (A) CeO2-CTAB0.5, (B) CeO2-CTAB1.0 and (C) CeO2-CTAB2.0. (D) XRD patterns of precursor and calcined product of CeO2-CTAB 1.0. (E) The N2 adsorption and desorption isotherm curves. (F) Pore size distribution curves of CeO2.
To further investigate the microstructure of CeO2 and assess its influence on performance, TEM testing was conducted [Figure 2]. Figure 2A-C further reveals the aggregation of smaller structures. Interestingly, the ratio of rod-shaped CeO2 increases significantly with the rising amount of CTAB. For the small granular CeO2, the observed interplanar spacing is 0.312 nm [Figure 2D], which corresponds to its (111) crystal plane. This crystal plane is considered the most stable low-index plane for CeO2 and is exposed typically on granular CeO2 surfaces[27,28]. For the rod-shaped CeO2, the observed interplanar spacing is primarily
Figure 2. TEM morphology of (A) CeO2-CTAB0.5, (B) CeO2-CTAB1.0 and (C) CeO2-CTAB2.0. HR-TEM of (D) granular CeO2 and (E and F) rod-shaped CeO2 in CeO2-CTAB1.0.
To determine whether the rod-shaped CeO2 structures are formed during the precipitation or calcination process, TEM testing was also conducted on the cerium formate precursor [Figure 3A]. However, no rod-shaped morphology is observed in the precursor, suggesting that the formation of rod-shaped CeO2 structures occurs during subsequent calcination rather than inherited by the precipitation, and the presence of a mushy substance on the surface indicates the presence of CTAB. The DSC/TGA curves of the precursor [Figure 3B] reveal that the main decomposition temperature of Ce(HCOO)3 is approximately 320 °C, and previous weight loss is primarily due to dehydration and CTAB decomposition[32]. Based on these findings, the calcination process was adjusted (two-hour calcination at 300 °C followed by one-hour calcination at 500 °C). As a result, all the rod-shaped CeO2 structures disappear [Figure 3C], and the exposed crystal faces of small particles are (111) plane [Figure 3D]. This outcome can be attributed to the complete decomposition of CTAB at 300 °C, which effectively halts its contribution to the subsequent growth of CeO2. When the CTAB is removed ahead, the growth of CeO2 would be along the (111) crystal plane with the lowest energy[33]. With the adsorption of CTAB, the growth of the (111) crystal plane is obstructed, and its growth would follow other directions to form the rod shape.
Figure 3. (A) TEM morphology and (B) TGA/DSC curves of cerium formate precursor. (C) TEM and (D) HRTEM morphology of
The NRA performance of prepared catalysts is evaluated. LSV results [Figure 4A] reveal a significant increase in current density at the same potential after the addition of
Figure 4. (A) LSV curves of CeO2 in a 0.5 M Na2SO4 electrolyte with and without
DFT calculations are employed to investigate the effect of exposed crystal planes on NRA. The calculated model of CeO2 is shown in Supplementary Figure 10. Based on TEM analysis results, 2 × 2 surfaces of CeO2 (111), (200), and (220) planes are considered for the calculation processes [Supplementary Figure 11]. The adsorption energies of H and
Figure 5. (A) Adsorption energy of
The change of Gibbs free energy throughout the entire NRA reactions is calculated for (200) and (220) planes [Figure 5B] and the configurations are shown in Supplementary Figures 12 and 13. For the CeO2 with (220) plane, the rate-determining step (RDS) is *NO to *N step with an energy barrier of 3.5 eV. For the CeO2 with (200) plane, the RDS is *NH to *NH2 step with an energy barrier of 2.1 eV. For the aspect of free energy, the NRA tends to occur on the CeO2 (200) plane. The energy barrier (2.1 eV) is close to many reported high-performance NRA electrocatalysts, such as TiO2[37], Rh[38], and NiCo2O4[39]. The improved NRA performance observed in experiments is attributed to the high affinity for
CONCLUSIONS
In this work, the exposed crystal planes of CeO2 are controlled by adjusting the amount of added CTAB. With the increase of CTAB, the exposed crystal planes of CeO2 are transferred from (111) to (200)/(220) planes. This transition occurred due to the obstruction of the growth of the (111) crystal plane by the adsorbed CTAB.
The CeO2 with exposed (220)/(200) planes presents higher NRA performance than that of CeO2 with the (111) plane. Compared with the CeO2 with exposed (111) plane, the YieldNH3 and FE of CeO2 with exposed (200)/(220) planes increase by 11.9% and 37.7%, respectively. DFT is used to reveal the underlying mechanism. The exposed (111) plane of CeO2 is hard to adsorb
DECLARATIONS
Acknowledgments
The authors thank the Analytical & Testing Center of Sichuan University (CASTEP).
Authors’ contributions
Conceived and designed the study: Mao J, Li D, Wang F
Prepared the samples and collected the data: Li D
Performed data analysis and wrote the main draft of the paper: Li D, Wang F
All authors discussed the results and commented on the manuscript.
Availability of data and materials
Not applicable.
Financial support and sponsorship
This work was supported by the “Natural Science Foundation of Sichuan Province” [24NSFSC3150], the “2021 Strategic Cooperation Project between Sichuan University and The People's Government of Luzhou” [2021CDLZ-1], and “Special Funding for Postdoctoral Research Project of Sichuan Province” [TB2023053].
Conflicts of interest
All authors declared that there are no conflicts of interest.
Ethical approval and consent to participate
Not applicable.
Consent for publication
Not applicable.
Copyright
© The Author(s) 2024.
Supplementary Materials
REFERENCES
1. Huang Y, Liu S, Pei M, Li J, Xu H, Chen Y. Unveiling H2O2-optimized NOx adsorption-selective catalytic reduction (AdSCR) performance of WO3/CeZrO2 catalyst. Rare Met 2023;42:3755-65.
2. Ju L, Tang X, Kou L. Polarization boosted catalysis: progress and outlook. Microstructures 2022;2:2022008.
3. Li X, Shen P, Li X, Ma D, Chu K. Sub-nm RuOx clusters on Pd metallene for synergistically enhanced nitrate electroreduction to ammonia. ACS Nano 2023;17:1081-90.
4. Wu Y, Yao K, Zhao Z, et al. Efficient electrocatalytic reduction of nitrate to ammonia over Mo2CTx micro-foam with rich edge sites. Chem Eng J 2024;479:147602.
5. Soloveichik G. Electrochemical synthesis of ammonia as a potential alternative to the Haber-Bosch process. Nat Catal 2019;2:377-80.
6. Luo H, Li S, Wu Z, et al. Modulating the active hydrogen adsorption on Fe-N interface for boosted electrocatalytic nitrate reduction with ultra-long stability. Adv Mater 2023;35:e2304695.
7. Wu Z, Song Y, Guo H, et al. Tandem catalysis in electrocatalytic nitrate reduction: unlocking efficiency and mechanism. Interdiscip Mater 2024;3:245-69.
8. Sun L, Liu B. Mesoporous PdN alloy nanocubes for efficient electrochemical nitrate reduction to ammonia. Adv Mater 2023;35:e2207305.
9. Yang G, Zhou P, Liang J, Li H, Wang F. Opportunities and challenges in aqueous nitrate and nitrite reduction beyond electrocatalysis. Inorg Chem Front 2023;10:4610-31.
10. Liang X, Zhu H, Yang X, et al. Recent advances in designing efficient electrocatalysts for electrochemical nitrate reduction to ammonia. Small Struct 2023;4:2200202.
11. Zhou J, Wen M, Huang R, et al. Regulating active hydrogen adsorbed on grain boundary defects of nano-nickel for boosting ammonia electrosynthesis from nitrate. Energy Environ Sci 2023;16:2611-20.
12. Peng O, Hu Q, Zhou X, et al. Swinging hydrogen evolution to nitrate reduction activity in molybdenum carbide by ruthenium doping. ACS Catal 2022;12:15045-55.
13. Yang R, Li H, Long J, Jing H, Fu X, Xiao J. Potential dependence of ammonia selectivity of electrochemical nitrate reduction on copper oxide. ACS Sustain Chem Eng 2022;10:14343-50.
14. Masuda T, Fukumitsu H, Fugane K, et al. Role of cerium oxide in the enhancement of activity for the oxygen reduction reaction at
15. Zhu Y, Liu S, Jin C, Bie S, Yang R, Wu J. MnOx decorated CeO2 nanorods as cathode catalyst for rechargeable lithium-air batteries. J Mater Chem A 2015;3:13563-7.
16. Lu G, Zheng H, Lv J, Wang G, Huang X. Review of recent research work on CeO2-based electrocatalysts in liquid-phase electrolytes. J Power Sources 2020;480:229091.
17. Zhou A, Wang D, Li Y. Hollow microstructural regulation of single-atom catalysts for optimized electrocatalytic performance. Microstructures 2021;2:2022005.
18. Bi H, Yin X, He J, et al. Conjugated organic component-functionalized hourglass-type phosphomolybdates for visible-light photocatalytic Cr(VI) reduction in wide pH range. Rare Met 2023;42:3638-50.
19. Xie H, Wang H, Geng Q, et al. Oxygen vacancies of Cr-Doped CeO2 nanorods that efficiently enhance the performance of electrocatalytic N2 fixation to NH3 under ambient conditions. Inorg Chem 2019;58:5423-7.
20. Liu Y, Li C, Guan L, Li K, Lin Y. Oxygen vacancy regulation strategy promotes electrocatalytic nitrogen fixation by doping Bi into Ce-MOF-Derived CeO2 nanorods. J Phys Chem C 2020;124:18003-9.
21. Wang X, Sun C, He F, et al. Enhanced hydrogen evolution reaction performance of NiCo2P by filling oxygen vacancies by phosphorus in thin-coating CeO2. ACS Appl Mater Interfaces 2019;11:32460-8.
22. Shi Y, Fu J, Hui K, et al. Promoting the electrochemical properties of yolk-shell-structured CeO2 composites for lithium-ion batteries. Microstructures 2021;1:2021005.
23. Zhang W, Shen Y, Pang F, et al. Facet-dependent catalytic performance of Au nanocrystals for electrochemical nitrogen reduction. ACS Appl Mater Interfaces 2020;12:41613-9.
24. Wu T, Stone ML, Shearer MJ, et al. Crystallographic facet dependence of the hydrogen evolution reaction on CoPS: theory and experiments. ACS Catal 2018;8:1143-52.
25. Gong Z, Zhong W, He Z, et al. Regulating surface oxygen species on copper (I) oxides via plasma treatment for effective reduction of nitrate to ammonia. Appl Catal B Environ 2022;305:121021.
26. Rahman MM, Muttakin M, Pal A, Shafiullah AZ, Saha BB. A statistical approach to determine optimal models for IUPAC-classified adsorption isotherms. Energies 2019;12:4565.
27. Sayle DC, Maicaneanu SA, Watson GW. Atomistic models for CeO2 (111), (110), and (100) nanoparticles, supported on yttrium-stabilized zirconia. J Am Chem Soc 2002;124:11429-39.
28. Lundberg M, Skårman B, Reine Wallenberg L. Crystallography and porosity effects of CO conversion on mesoporous CeO2. Microporous Mesoporous Mater 2004;69:187-95.
29. Qu J, Wang Y, Mu X, et al. Determination of crystallographic orientation and exposed facets of titanium oxide nanocrystals. Adv Mater 2022;34:e2203320.
30. Mai HX, Sun LD, Zhang YW, et al. Shape-selective synthesis and oxygen storage behavior of ceria nanopolyhedra, nanorods, and nanocubes. J Phys Chem B 2005;109:24380-5.
31. Zhou K, Wang X, Sun X, Peng Q, Li Y. Enhanced catalytic activity of ceria nanorods from well-defined reactive crystal planes. J Catal 2005;229:206-12.
32. Sui Z, Chen X, Wang L, et al. Capping effect of CTAB on positively charged Ag nanoparticles. Physica E Low Dimens Syst Nanostruct 2006;33:308-14.
33. Bakshi MS. How surfactants control crystal growth of nanomaterials. Cryst Growth Des 2016;16:1104-33.
34. Li T, Li J, Zhang L, et al. Work-function-induced interfacial electron redistribution of MoO2/WO2 heterostructures for high-efficiency electrocatalytic hydrogen evolution reaction. Rare Met 2024;43:489-99.
35. Guo Z, Yu Y, Li C, et al. Deciphering structure-activity relationship towards CO2 electroreduction over SnO2 by a standard research paradigm. Angew Chem Int Ed 2024;63:e202319913.
36. Ling C, Zhang Y, Li Q, Bai X, Shi L, Wang J. New mechanism for N2 reduction: the essential role of surface hydrogenation. J Am Chem Soc 2019;141:18264-70.
37. García-mota M, Vojvodic A, Metiu H, et al. Tailoring the activity for oxygen evolution electrocatalysis on rutile TiO2 (110) by transition-metal substitution. ChemCatChem 2011;3:1607-11.
38. Li T, Han S, Cheng C, et al. Sulfate-enabled nitrate synthesis from nitrogen electrooxidation on a rhodium electrocatalyst. Angew Chem Int Ed 2022;134:e202204541.
Cite This Article
How to Cite
Download Citation
Export Citation File:
Type of Import
Tips on Downloading Citation
Citation Manager File Format
Type of Import
Direct Import: When the Direct Import option is selected (the default state), a dialogue box will give you the option to Save or Open the downloaded citation data. Choosing Open will either launch your citation manager or give you a choice of applications with which to use the metadata. The Save option saves the file locally for later use.
Indirect Import: When the Indirect Import option is selected, the metadata is displayed and may be copied and pasted as needed.
About This Article
Copyright
Data & Comments
Data
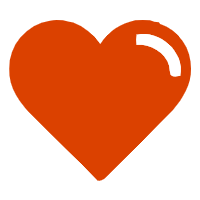
Comments
Comments must be written in English. Spam, offensive content, impersonation, and private information will not be permitted. If any comment is reported and identified as inappropriate content by OAE staff, the comment will be removed without notice. If you have any queries or need any help, please contact us at [email protected].