Misfit strain-misfit strain phase diagram of (110)-oriented ferroelectric PbTiO3 films: a phase-field study
Abstract
Ferroelectric thin films with high index orientations are found to possess unique structures and properties. In this work, we constructed the misfit strain-misfit strain phase diagram of (110)-oriented PbTiO3 (PTO) thin films by phase-field simulations. The evolutions of ferroelectric phase structures, domain morphologies, volume fractions, and polarization components with the anisotropic strains were analyzed in detail. Large anisotropic strains exist between the orthorhombic scandate substrates and (110)-oriented PTO films, which makes it possible to engineer the structures and properties by anisotropic strain. These results deepen the understanding of ferroelectric domain structures of (110)-oriented PTO films under the anisotropic strain and provide theoretical support for the anisotropic strain engineering of high-index thin films experimentally.
Keywords
INTRODUCTION
Ferroelectric materials have broad applications in the fields of sensors, actuators, and non-volatile memories[1-5] and have attracted abundant attention in recent years. How to regulate the excellent properties of ferroelectric materials is the focus of attention. In this process, researchers have tried many methods, such as strain[6-10], film thickness[11-13], electrical boundary condition[14,15], growth orientation[16-20], etc. Both experiments and theoretical simulations have proved that the ferroelectric thin films with high index orientations, such as (110)- and (111)-orientations, have unique structures and properties different from those with low index orientation, such as the (001)-orientation[21-26]. PbTiO3 (PTO) is a prototypical ferroelectric material, which undergoes a cubic-to-tetragonal ferroelectric transition at about 765 K[27,28]. For (110)-oriented PTO films, the temperature-misfit strain phase diagrams[29] were constructed by the phenomenological theory, which indicates that various low-symmetry phases could emerge at different strain states. However, the phenomenological theory only considers single-domain states and prescribed multi-domain states. In contrast, the phase-field simulations could predict the optimal multi-domain structures under certain external conditions and their evolutions with the external field. In our previous work, the temperature-misfit strain phase diagram of the (110)-oriented PTO film was constructed by phase-field simulations, and the effects of epitaxial strain on the structures and properties were systematically investigated[30].
Due to the anisotropy of the crystal, the (110)-oriented ferroelectric films can exhibit unique properties. Experimentally, there are many orthogonal substrates that exert asymmetric in-plane strain. For example, the orthogonal NdGaO3 substrate applies the asymmetric strain to the (110)-oriented Ba1-xSrxTiO3 film, resulting in strong in-plane dielectric anisotropy[17,31]. For ferroelectric PTO, the anisotropic misfit strain phase diagrams[32] were constructed by the phenomenological theory. However, there are no phase-field studies that could provide predictive anisotropic misfit strain multi-domain phase diagrams for experimentalists.
In this paper, the effect of asymmetric misfit strain on ferroelectric phase (domain) structures of (110)-orientated PTO films is constructed by analyzing the phase-field data via the stereographic projection (SP) method[30,33,34]. Then, the typical phase (domain) structures under asymmetric misfit strain states and their evolution with strain are analyzed in detail. Finally, it is pointed out that a series of orthogonal substrates can apply large asymmetric misfit strains to achieve the regulation of (110)-oriented PTO films. These results help to deepen the understanding of ferroelectric domain structures under high index asymmetric misfit strain and provide theoretical guidance for the design of ferroelectric devices based on asymmetric misfit strain regulation.
METHODS
Phase field model
The phase-field model suitable for the (110)-oriented ferroelectric films was constructed in our previous work[30]. Here, the main formulae were briefly outlined. A new coordinate system (x1, x2, x3) with axes along the [100], [0
where the transformation matrix Tij can be written as follows:
The temporal evolution of Pi is modeled via numerically solving the time-dependent Ginzburg-Landau (TDGL) equation:
where t is the time step, L is the kinetic coefficient related to the domain wall mobility, and the total free energy F consists of the following contributions:
The first term is the bulk energy density,
where
where
where Cijkl is the elastic stiffness tensor involving
where
The thermodynamic parameters of PTO used in this paper refer to the previous literature[30] and are listed in Table 1. The conversion relationship between these coefficients and those under the (001) orientation is shown in Table 2. The coefficients with the superscript ‘*’ denote those in the (110) orientation.
Material parameters of PbTiO3 used in the phase-field simulations
α1 | 3.8(T - 479) × 105 | C-2·m2·N | Q11 | 0.089 | C-2·m4 |
α11 | -7.3 × 107 | C-4·m6·N | Q12 | -0.026 | C-2·m4 |
α12 | 7.5 × 108 | C-4·m6·N | Q44 | 0.0675 | C-2·m4 |
α111 | 2.6 × 108 | C-6·m10·N | G11 | 1.035 × 10-10 | C-2·m4·N |
α112 | 6.1 × 108 | C-6·m10·N | G12 | 0 | C-2·m4·N |
α123 | -3.7 × 109 | C-6·m10·N | G44 | 5.176 × 10-11 | C-2·m4·N |
C11 | 1.746 × 1011 | N·m-2 | εb | 40 | |
C12 | 7.937 × 1010 | N·m-2 | |||
C44 | 1.111 × 1011 | N·m-2 |
The relations of the energy coefficients to transform from (001)-oriented coordinate systems to (110)-oriented coordinate systems
Landau-devonshire coefficients | |
Gradient coefficients | |
Elastic stiffness tensors | |
Electrostrictive coefficients |
To construct the misfit strain-misfit strain phase diagram of (110)-oriented PTO films, we employed
RESULTS AND DISCUSSION
Misfit strain-misfit strain phase diagram
A series of equilibrium structures under different misfit strains ε11 and ε22 are calculated by phase-field simulations. Firstly, the structures of (110)-oriented PTO films are analyzed via the SP method, and the type of phase at each position of misfit strain-misfit strain space is determined, as shown in Figure 1. There are several phases, including the tetragonal phase (Ta: P1≠ 0, P2= P3= 0, Tc: P2= P3≠ 0, P1= 0), the orthogonal phase (Oc: P3 ≠ 0, P1 = P2 = 0), the monoclinic phase (Mc: P2 ≠ P3 ≠ 0, P1 = 0), and the rhombohedral phase
Figure 1. The stereographic projections of ferroelectric phases for (110)-oriented PTO thin films under anisotropic misfit strains at room temperature. (A-H) The stereographic projections of typical ferroelectric phases under different strain states. The schematic diagram in the center gives the ideal locations of different ferroelectric phases for (110)-oriented PTO films. The ranges of tetragonal-like, orthorhombic, and rhombohedral-like phases are marked by green, red, and orange solid circles. The magenta dashed circle indicates the position where the polarization vector deviates from the x3 axis by 45°, and the cyan dashed lines mark the four in-plane <111> directions. The color reflects the intensity of the projection scatters.
When the strain state of the PTO film is the symmetrical strain of ε11 = ε22 = -4%, the peak of the projection point is located at the center of the SPs, as shown in Figure 1A, which indicates that the polarization vectors are parallel to the x3 axis, that is, the Oc phase. Keeping ε11 at a compressive strain of -4% and changing ε22 toward the tensile strain gradually, the peak of the projection point splits into two parts along the x2 axis, corresponding to the two polarized variants of the Mc phase, as shown in Figure 1B. When ε22 continues to increase to 4% of the tensile strain, the peaks of the Mc phase enter the range of the Tc phase, the green circles, as shown in Figure 1C. When ε22 is maintained at 4%, and ε11 is gradually changed toward the tensile strain, it is found that the peak of the Mc phase continues to deflect in the in-plane direction. When ε11 = 1%, the peaks of the Ta phase emerge at both ends of the x1 axis, and the phase structure is Ta/Mc mixed phase, as shown in Figure 1D. Further increasing ε11, the peaks of the R phase emerge. When the strain is ε11 = ε22 = 4%, the peaks of the Mc phase disappear, resulting in the Ta/R phase, as shown in Figure 1E. Keeping ε11 at 4% and decreasing ε22, it is found that the peak of the R phase gradually weakens, and the peak of the Mc phase begins to appear on the x2 axis. When the strain state is ε11 = 4% and ε22 = 1%, the phase structure evolves into the Ta/Mc phase again, as shown in Figure 1F. With the decrease of ε22, the peaks of the Mc phase gradually disappear, and the phase structure transforms into the pure Ta phase, as shown in Figure 1G. Finally, the compressive strain of ε22 is maintained at -4%, after which ε11 is gradually reduced. Under the asymmetric strain of ε11 = 0% and ε22 = -4%, the peak of the Oc phase appears in the central region of the SPs, forming the
Based on the above analysis, the misfit strain-misfit strain phase diagram of the (110)-oriented PTO film with a thickness of 20 nm at room temperature was constructed, as shown in Figure 2. It can be seen from the phase diagram that there are seven kinds of ferroelectric phases at room temperature. Under a large compressive strain (the left-bottom corner), an out-of-plane polarized Oc phase region appears. Under large asymmetric strains (the left-top and right-bottom corners), there are single-phase regions of the Ta phase and the Mc phase. In other regions, there are some mixed-phase regions, including the Ta/Oc, Ta/Mc, and
Typical domain structures under asymmetric strain
Figure 3 gives the typical domain structure in (110)-oriented PTO films under different strain states. Figure 3A shows the orthorhombic Oc phase under the large compressive strain in the x1 and x2 directions, which consists of two polar variants, Oc+(0, 0, +P3) and Oc-(0, 0, -P3), perpendicular to the interface of the film and the substrate. Figure 3B shows the monoclinic Mc phase under the compressive strain in the x1 direction and the tensile strain in the x2 direction, which consists of two polarized anti-parallel polarization variants, Mc2+(0, +P2, -P3) and Mc2-(0, -P2, +P3). Figure 3C is the tetragonal-like Ta phase under the tensile strain in the x1 direction and the compressive strain in the x2 direction, which consists of two polarization variants, Ta+(+P1, 0, 0) and Ta-(-P1, 0, 0), with anti-parallel polarizations along the x1 axis. These three single phases are all featured by 180° domain structures. The domain walls in the Oc phase are perpendicular to the interface, and the domain structures are maze-like. The domain wall in the Mc phase tilts to the interface, and the tilt angle changes with the asymmetric strain of the substrate. In contrast, the domain walls in the Ta phase are straight and perpendicular to the x2 axis.
Figure 3. Typical domain structures of (110)-oriented PTO thin films under different misfit strains. (A) The Oc phase; (B) The Mc phase; (C) The Ta phase; (D) The Ta/Oc phase; (E) The Ta/Mc phase; (F) The Ta/R phase. The polarization variants are denoted by different colors, as labeled on the right.
Figure 3D shows the Ta/Oc mixed phase under zero strain in the x1 direction and large compressive strain in the x2 direction. It contains two tetragonal variants, Ta+(+P1, 0, 0), Ta-(-P1, 0, 0), and two orthogonal variants, Oc+(0, 0, +P3) and Oc-(0, 0, -P3). The 90° ferroelastic domain wall is between the Ta phase and the Oc phase. The Ta/Mc mixed phase under a large tensile strain in the x2 direction shown in Figure 3E contains two tetragonal variants, Ta+(+P1, 0, 0) and Ta-(-P1, 0, 0), and two monoclinic variants, Mc2+(0, +P2, -P3) and
Among the above-mentioned typical domain structures, the Ta/Oc phase is a newly emerged mixed phase under the asymmetric strain. Figure 4 shows the three-dimensional domain structure of the Ta/Oc phase and the cross-sectional view from different directions. From the vertical cross-section in Figure 4B, we can see the vertical and horizontal alternating domain structure and the inclined 90° domain wall, and the angle between the domain wall and the interface is about 50°. The reason that the angle deviates from 45° is that the polarization magnitudes of the Ta and Oc phases are not equal. The horizontal cross-section of Figure 4C reflects the irregular strip domain structure and 90° domain wall from another direction.
Figure 4. Domain structures of the Ta/Oc phase in (110)-oriented PTO thin films under the anisotropy strains of ε11 = 0% and ε22 = -4%. (A) The 3D domain structure; (B) The vertical cross-section; (C) The horizontal cross-section of the white dashed boxes in (A) The bars in
The effect of asymmetric strain on domain structures
In order to further reveal the effect of asymmetric strain on the domain structure of (110)-oriented PTO films, the domain structure and domain morphology of the Ta/Mc phase under different strains are analyzed in this section, as shown in Figure 5. Figure 5A shows the Ta/Mc phase structure under the symmetric strain of ε11 = ε22 = 1%. The strip-like Ta phase and the Mc phase (the volume fraction is about 66.1%) coexist, and the domain wall density is high. When increasing the strain in the x2 direction to ε22 = 4%, as shown in Figure 5B, the volume fraction of the Mc phase increases (about 88.3%) at the expanse of the strip Ta phase. When increasing the strain in the x1 direction to ε11 = 4%, however, the volume fraction of the Ta phase increases while that of the Mc phase decreases (about 9.3%), as shown in Figure 5C.
Figure 5. Domain morphologies of the Ta/Mc phase under different misfit strains. (A) ε11 = ε22 = 1.0%; (B) ε11 = 1.0%, ε22 = 4.0% and (C)
Figure 6 is the evolution of the phase structure with the strain ε11 under fixed ε22. When ε22 = -2%, it can be seen from Figure 6A that with the increase of ε11, the phase structure undergoes the evolution path of
Figure 6. Phase structure evolutions of (110)-oriented PTO films with respective to misfit strain ε11 at various constant strain ε22. (A-C)
Figure 7 is the evolution of the phase structure with the misfit strain ε22 when the misfit strain ε11 is fixed. At ε11 = -2%, with the increase of ε22, the phase structure evolves from the Oc phase to the Mc phase, as shown in Figure 7A. Figure 7B reflects the evolution of the polarization component. When ε22 = -3%, the in-plane Py component emerges and gradually increases with ε22, while the out-of-plane Pz component increases first and then decreases. Figure 7C shows the change of the angle between the polarization of the Mc(Oc) phase and the x3 axis. In the Mc phase region, the angle increases from 0 to 51.5°, indicating that the polarization of the Mc phase gradually rotates to the in-plane direction with the increase of ε22. At ε11 = 0%, it can be seen from the phase volume fraction in Figure 7D that with the increase of ε22, the evolution path is
Figure 7. Phase structure evolutions of (110)-oriented PTO films with respective to misfit strain ε22 at various constant strains ε11.
Asymmetric strain between the orthogonal substrates and the film
In recent years, with the development of a series of commercial orthogonal substrates, it is possible to control the domain structure in (110)-oriented PTO films by the asymmetric strain. The lattice constants of the commonly used orthorhombic scandate and gallate substrates and the mismatch strain between them and the (110)-oriented PTO film are listed in Table 3. In the phase-field simulation, the lattice constants of the (110)-oriented PTO film in the in-plane x1[100] and x2[011] directions are ac = 3.957 Å and
Figure 8. The strain positions of orthorhombic scandate and gallate substrates in the phase diagram.
The lattice constants of commonly used orthorhombic scandate and gallate substrates and the misfit strains between these substrates and (110)-oriented PTO films
Substrate | a/Å | b/Å | c/Å | (100)O substrate | (010)O substrate | ||
[100]//[001]O | [011]//[010]O | [100]//[001]O | [011]//[100]O | ||||
DyScO3 | 5.440 | 5.717 | 7.903 | -0.14% | 2.16% | -0.14% | -2.79% |
TbScO3 | 5.466 | 5.731 | 7.917 | 0.04% | 2.41% | 0.04% | -2.32% |
GdScO3 | 5.480 | 5.746 | 7.932 | 0.23% | 2.68% | 0.23% | -2.07% |
SmScO3 | 5.527 | 5.758 | 7.965 | 0.64% | 2.89% | 0.64% | -1.23% |
NdScO3 | 5.575 | 5.776 | 8.003 | 1.12% | 3.22% | 1.12% | -0.38% |
PrScO3 | 5.608 | 5.780 | 8.025 | 1.40% | 3.29% | 1.40% | 0.21% |
NdGaO3 | 5.433 | 5.504 | 7.716 | -2.50% | -1.64% | -2.50% | -2.91% |
PrGaO3 | 5.459 | 5.493 | 7.732 | -2.30% | -1.84% | -2.30% | -2.45% |
LaGaO3 | 5.494 | 5.527 | 7.778 | -1.72% | -1.23% | -1.72% | -1.82% |
CONCLUSIONS
In this work, the misfit strain-misfit strain multi-domain phase diagram of (110)-oriented PTO thin films at room temperature was constructed by phase-field simulations. Three single phases and four mixed phases were predicted to exist due to the anisotropic strain. The single phases (Oc and Mc) mainly exist within the phase region characterized by compressive ε11, and the phase Ta, which is typically observed only at high temperatures under the isotropic strain conditions, emerges within the phase region marked by the tensile
DECLARATIONS
Authors’ contributions
Data analysis, interpretation, initial draft writing, and manuscript revision: Li HM
Data acquisition: Zhang H
Manuscript revision; funding acquisition: Wang YJ
Funding acquisition: Tang YL, Zhu YL, Ma XL
Availability of data and materials
The data that support the findings of this study are available from the corresponding author upon reasonable request.
Financial support and sponsorship
This work is supported by the National Natural Science Foundation of China (52122101 and 51971223) and Shenyang National Laboratory for Materials Science (L2019R06, L2019R08, L2019F01, L2019F13). Wang YJ and Tang YL acknowledge the Youth Innovation Promotion Association CAS (2021187 and Y202048). Tang YL acknowledges the Scientific Instrument Developing Project of CAS (YJKYYQ20200066). We are grateful to Prof. Li J. Y. at Southern University of Science and Technology and Dr. Lei C. H. at Saint Louis University for the development of the phase-field code.
Conflicts of interest
All authors declared that there are no conflicts of interest.
Ethical approval and consent to participate
Not applicable.
Consent of publication
Not applicable.
Copyright
© The Author(s) 2024.
REFERENCES
1. Muralt P. Ferroelectric thin films for micro-sensors and actuators: a review. J Micromech Microeng 2000;10:136.
2. Setter N, Damjanovic D, Eng L, et al. Ferroelectric thin films: review of materials, properties, and applications. J Appl Phys 2006;100:051606.
4. Hoffman J, Pan X, Reiner JW, et al. Ferroelectric field effect transistors for memory applications. Adv Mater 2010;22:2957-61.
5. Martin LW, Rappe AM. Thin-film ferroelectric materials and their applications. Nat Rev Mater 2016;2:16087.
6. Pertsev NA, Zembilgotov AG, Tagantsev AK. Effect of mechanical boundary conditions on phase diagrams of epitaxial ferroelectric thin films. Phys Rev Lett 1998;80:1988.
7. Li YL, Hu SY, Liu ZK, Chen LQ. Effect of substrate constraint on the stability and evolution of ferroelectric domain structures in thin films. Acta Mater 2002;50:395-411.
8. Schlom DG, Chen LQ, Eom CB, Rabe KM, Streiffer SK, Triscone JM. Strain tuning of ferroelectric thin films. Annu Rev Mater Res 2007;37:589-626.
9. Damodaran AR, Agar JC, Pandya S, et al. New modalities of strain-control of ferroelectric thin films. J Phys Condens Matter 2016;28:263001.
10. Damodaran AR, Pandya S, Agar JC, et al. Three-state ferroelastic switching and large electromechanical responses in PbTiO3 thin films. Adv Mater 2017;29:1702069.
11. Sheng G, Hu JM, Zhang JX, Li YL, Liu ZK, Chen LQ. Phase-field simulations of thickness-dependent domain stability in PbTiO3 thin films. Acta Mater 2012;60:3296-301.
12. Nesterov O, Matzen S, Magen C, Vlooswijk AHG, Catalan G, Noheda B. Thickness scaling of ferroelastic domains in PbTiO3 films on DyScO3. Appl Phys Lett 2013;103:142901.
13. Li S, Zhu YL, Tang YL, et al. Thickness-dependent a1/a2 domain evolution in ferroelectric PbTiO3 films. Acta Mater 2017;131:123-30.
14. Nelson CT, Winchester B, Zhang Y, et al. Spontaneous vortex nanodomain arrays at ferroelectric heterointerfaces. Nano Lett 2011;11:828-34.
15. Wang YJ, Feng YP, Zhu YL, et al. Polar meron lattice in strained oxide ferroelectrics. Nat Mater 2020;19:881-6.
16. Tagantsev AK, Pertsev NA, Muralt P, Setter N. Strain-induced diffuse dielectric anomaly and critical point in perovskite ferroelectric thin films. Phys Rev B 2001;65:012104.
17. Simon WK, Akdogan EK, Safari A, Bellotti JA. In-plane microwave dielectric properties of paraelectric barium strontium titanate thin films with anisotropic epitaxy. Appl Phys Lett 2005;87:082906.
18. Ouyang J, Slusker J, Levin I, et al. Engineering of self-assembled domain architectures with ultra-high piezoelectric response in epitaxial ferroelectric films. Adv Funct Mater 2007;17:2094-100.
19. Cao Y, Sheng G, Zhang JX, et al. Piezoelectric response of single-crystal PbZr1-xTixO3 near morphotropic phase boundary predicted by phase-field simulation. Appl Phys Lett 2010;97:252904.
20. Xu R, Karthik J, Damodaran AR, Martin LW. Stationary domain wall contribution to enhanced ferroelectric susceptibility. Nat Commun 2014;5:3120.
21. Gui Z, Prosandeev S, Bellaiche L. Properties of epitaxial (110) BaTiO3 films from first principles. Phys Rev B 2011;84:214112.
22. Xu R, Zhang J, Chen Z, Martin LW. Orientation-dependent structural phase diagrams and dielectric properties of PbZr1-xTixO3 polydomain thin films. Phys Rev B 2015;91:144106.
23. Lee JH, Chu K, Kim KE, Seidel J, Yang CH. Out-of-plane three-stable-state ferroelectric switching: finding the missing middle states. Phys Rev B 2016;93:115142.
24. Feng YP, Jiang RJ, Zhu YL, et al. Strain coupling of ferroelastic domains and misfit dislocations in [101]-oriented ferroelectric PbTiO3 films. RSC Adv 2022;12:20423-31.
25. Xu R, Gao R, Reyes-Lillo SE, et al. Reducing coercive-field scaling in ferroelectric thin films via orientation control. ACS Nano 2018;12:4736-43.
26. Feng YP, Tang YL, Zhu YL, Zou MJ, Wang YJ, Ma XL. Thickness-dependent evolution of piezoresponses and a/c domains in -oriented PbTiO3 ferroelectric films. J Appl Phys 2020;128:224102.
28. Jung WW, Lee HC, Ahn WS, Ahn SH, Choi SK. Switchable single c-domain formation in a heteroepitaxial PbTiO3 thin film on a (001) Nb-SrTiO3 substrate fabricated by means of hydrothermal epitaxy. Appl Phys Lett 2005;86:252901.
29. Mtebwa M, Tagantsev AK, Yamada T, Gemeiner P, Dkhil B, Setter N. Single-domain (110) PbTiO3 thin films: thermodynamic theory and experiments. Phys Rev B 2016;93:144113.
30. Zhang H, Feng YP, Wang YJ, Tang Y, Zhu Y, Ma X. Strain phase diagram and physical properties of (110)-oriented PbTiO3 thin films by phase-field simulations. Acta Mater 2022;228:117761.
31. Akcay G, Misirlioglu IB, Alpay SP. Dielectric tunability of (110) oriented barium strontium titanate epitaxial films on (100) orthorhombic substrates. Appl Phys Lett 2006;89:042903.
32. Ma W, Wang F. Effect of in-plane strain anisotropy on (011) epitaxial BaTiO3 and PbTiO3 thin films. AIP Adv 2017;7:105120.
33. Guo XW, Wang YJ, Zhang H, Tang YL, Zhu YL, Ma XL. Misfit strain-temperature phase diagram of multi-domain structures in (111)-oriented ferroelectric PbTiO3 films. Acta Mater 2020;196:539-48.
34. Guo XW, Zou MJ, Wang YJ, Tang Y, Zhu YL, Ma X. Effects of anisotropic misfit strains on equilibrium phases and domain structures in (111)-oriented ferroelectric PbTiO3 films. Acta Mater 2021;206:116639.
35. Chen LQ. Phase-field method of phase transitions/domain structures in ferroelectric thin films: a review. J Am Ceram Soc 2008;91:1835-44.
Cite This Article

How to Cite
Download Citation
Export Citation File:
Type of Import
Tips on Downloading Citation
Citation Manager File Format
Type of Import
Direct Import: When the Direct Import option is selected (the default state), a dialogue box will give you the option to Save or Open the downloaded citation data. Choosing Open will either launch your citation manager or give you a choice of applications with which to use the metadata. The Save option saves the file locally for later use.
Indirect Import: When the Indirect Import option is selected, the metadata is displayed and may be copied and pasted as needed.
About This Article
Special Issue
Copyright
Data & Comments
Data
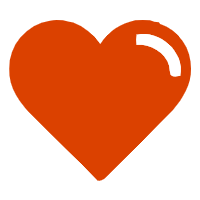
Comments
Comments must be written in English. Spam, offensive content, impersonation, and private information will not be permitted. If any comment is reported and identified as inappropriate content by OAE staff, the comment will be removed without notice. If you have any queries or need any help, please contact us at [email protected].