Characterization of fuel-induced water contamination: chemical composition, odor threshold, and ecotoxicological implications
Abstract
Fuel spills pose significant environmental risks, particularly to drinking water sources and aquatic ecosystems. The composition of fuels has changed over the decades to reduce fossil greenhouse gas emissions. In Sweden, although the number of spill incidents has declined, with around 600 cases reported annually, there remains limited knowledge on the environmental and health impacts of modern fuels. This study aimed to address this gap through comprehensive chemical analysis and ecotoxicological assessments of 31 fuel samples, including petrol, diesel, fuel oil, and marine gas oil. Using gas chromatography-mass spectrometry (GC-MS), we determined 53 substances, including aromatic and aliphatic hydrocarbons, ethers, esters, and 17 polycyclic aromatic hydrocarbons (PAHs). A key focus was on forming a stable water-accommodated fraction (WAF) to isolate non-dissolved fuel elements from water, which is crucial for assessing subsurface aquatic life and drinking water production impacts. Results indicated significant differences in fuel odor profiles, with ethers enhancing odor intensity. Petrol components showed higher water solubility than diesel, partly due to ethanol. Ecotoxicological tests revealed varying toxicity across fuels, with petrol showing the highest toxicity to aquatic organisms, although activated sludge exhibited resilience. Fuels containing water-soluble ethers posed the highest risks to drinking water, while modern diesel was of lower concern due to its low solubility and toxicity. In freshwater ecosystems, petrol and hydrophobic toxins in fuel oil had severe effects during spills. Overall, this study offers critical insights into the environmental impact of common fuels, supporting improved risk assessment and management strategies for spill mitigation and water resource protection.
Keywords
INTRODUCTION
Fuel spills pose a significant threat to environmental integrity and human health worldwide, including in Sweden, where they are the most common occurrence of environmental damage. Although the number of incidents has decreased in recent years thanks to improved practices, techniques, and, for some products, reduced usage, around 600 incidents are still addressed annually by emergency services and salvage teams[1,2]. These spills threaten drinking water sources and aquatic ecosystems, highlighting the need for detailed, up-to-date information about modern fuels and their environmental and human health impacts. The most extensive and transparent summary currently available is from the US and dates from 1999[3], making it less applicable to current conditions.
The chemical composition of petrol and diesel is strictly regulated under the Fuel Act[4] in Sweden. For instance, MK1 petrol (environmental class 1 petrol) is allowed to contain up to 35% volume of aromatic hydrocarbons. Aromatic hydrocarbons are a group of chemicals known for their significant environmental impact. From an environmental assessment perspective, it is not just the total volume of aromatic hydrocarbons that matters; the specific types of aromatics present are equally critical. This is because individual aromatic hydrocarbons vary significantly in toxicity and water solubility. Some can be more harmful and more soluble in water sources than others. Hence, understanding not only the total amount but also the precise composition of these aromatics in fuels is essential[5]. Accurate and up-to-date data on these substances are imperative for effectively assessing and managing environmental risks and ensuring water safety.
Key fuel components are aromatic and aliphatic hydrocarbons, ethers, esters, and polycyclic aromatic hydrocarbons (PAHs). Aromatic hydrocarbons are notable for their strong, sometimes unpleasant odors. They also have some solubility in water and specific health risks; for instance, benzene is well-known for its carcinogenicity[6]. Aliphatic hydrocarbons constitute the bulk of the fuels and have varying properties based on their chain length. Ethers are added to fuels to enhance performance, and at the same time, they are noted for their strong odor[7]. The addition of esters, such as oleic acid methyl ester, as bio-components will influence overall fuel properties, even the odor and potential toxicity of the fuel. In addition, the environmental persistence of PAHs, coupled with their potential to bioaccumulate and induce adverse health effects such as cancer and reproductive issues, underscores the environmental significance of these compounds.
Odors have long been associated with oil and fuel spills, as volatile and semi-volatile compounds create a wide variety of odors that can be easily recognized by humans. Some of the components may be detected by smell at very low detection levels[8]. Many substances in fuels can affect the smell and taste of water at concentrations lower than those that are toxic. For drinking water production, it is essential not only to avoid harmful health effects from pollutants but also to ensure the water is free of taste and odor[9]. While there are known odor thresholds for individual substances like ethers[7], comprehensive studies on the odor properties of fuel products mixed in water are limited. Such studies are necessary to understand how different substances might interact to enhance or reduce each other’s effects in water[10]. In-depth evaluations are needed to comprehend the full impact of fuel spills on drinking water resources.
Fuel-induced water contamination poses significant risks to both human health and wildlife[11]. Contaminants such as benzene, methyl tert-butyl ether (MTBE), and volatile organic compounds (VOCs) can leach into groundwater and surface water sources, jeopardizing drinking water quality and leading to adverse health effects including cancer and neurological disorders[2,12]. Inhalation exposure to volatile compounds from contaminated water sources can cause respiratory problems, while direct skin contact during recreational activities can result in irritation and absorption of harmful chemicals[13]. Consequently, modeling approaches are essential to estimate human exposure to fuel spill-related substances. Fuel contamination can also have detrimental effects on aquatic ecosystems, leading to decreased populations and biodiversity loss due to direct toxicity and bioaccumulation of contaminants in aquatic organisms[14]. Additionally, habitat degradation from fuel spills can disrupt ecosystem functions and lead to increases in harmful algal blooms and pathogenic bacteria[15]. All of this underscores the importance of preventing and mitigating fuel-induced water pollution through effective management practices and regulatory measures[2].
Fuel spills in aquatic environments pose significant risks not only to human health but also to both shoreline (beach zone) and open water (pelagic zone) ecosystems. The recent shift in fuel composition, including the increased addition of ethanol to petrol and biofuels such as hydrogenated vegetable oil (HVO) and rapeseed methyl ester (RME) to diesel, has likely altered the solubility and toxicity of these fuels. However, the extent and nature of these changes remain unclear, particularly regarding their ecotoxicity. Understanding the ecotoxicity of these new fuel products is particularly crucial for diesel fuels used by ships in large quantities on freshwater lakes, which are vulnerable to accidental discharges[16]. To address these concerns, this study aimed to fill key knowledge gaps and provide actionable insights into the environmental and health impacts of fuel spills, especially for surface water sources. The objectives included analyzing the chemical composition of common fuels available at gas stations to inform environmental risk assessments, investigating the transfer of the fuel compounds into the water phase, determining odor thresholds for fuel-water mixtures, and exploring the ecotoxicity of selected fuels on freshwater organisms. Additionally, guidelines were developed to assess the impact of ethers, especially from petrol spills, on surface water sources, supporting effective response planning and risk mitigation. The study also initiated the development of a predictive model to evaluate how individual chemical compounds and mixtures influence the odor characteristics of water. These objectives align with the broader goal of improving our understanding of fuel spills’ environmental and health impacts, thereby supporting evidence-based decision-making to mitigate risks and protect natural resources.
Descriptions of fuels included in the study, based on consumer-available information on the websites of the fuel providers, and the tests performed on each sample
Sample ID | Product name* | Claimed content | Analysis of fuel | PAH analysis of fuel | Analysis of WAF** | Odor test | Ecotoxicity test | Evaporation |
P95-1 | Petrol 95 Octane | E10 petrol, 95 octane, 10% ethanol, 68% bio naphtha | Yes | Yes | Yes | |||
P95-2 | Petrol 95 Octane | E10 petrol, 95 octane, 10% ethanol | Yes | Yes | Yes | |||
P95-3 | Petrol 95 Octane | E10 petrol, 95 octane, 10% ethanol | Yes | Yes | ||||
P95-4 | Petrol 95 Octane | E10 petrol, 95 octane, 10% ethanol | Yes | Yes | ||||
P95-5 | Petrol 95 Octane | E10 petrol, 95 octane, 10% ethanol | Yes | |||||
P95-6 | Petrol 95 Octane | E10 petrol, 95 octane, 10% ethanol | Yes | |||||
P98-1 | Petrol 98 Octane | E05 petrol, 98 octane, 5% ethanol | Yes | Yes | Yes | |||
P98-2 | Petrol 98 Octane | E05 petrol, 98 octane, 5% ethanol | Yes | Yes | Yes | Yes | ||
P98-3 | Petrol 98 Octane | E05 petrol, 98 octane, 5% ethanol | Yes | |||||
P98-4 | Petrol 98 Octane | E05 petrol, 98 octane, 5% ethanol | Yes | Yes | ||||
E85-1 | Ethanol 85 | E85 fuel, 85% ethanol and 15% petrol | Yes | Yes | Yes | |||
E85-2 | Ethanol 85 | E85 fuel, 85% ethanol and 15% petrol | Yes | Yes | Yes | |||
E85-3 | Ethanol 85 | E85 fuel, 85% ethanol and 15% petrol | Yes | |||||
E85-4 | Ethanol 85 | E85 fuel, 85% ethanol and 15% petrol | Yes | |||||
D-SE1 | MK1 Diesel | Diesel, 20% of HVO | Yes | Yes | Yes | |||
D-SE2 | MK1 Diesel | Diesel, 7% of RME | Yes | Yes | Yes | Yes | ||
D-SE3 | MK1 Diesel | Diesel, 6% RME | Yes | Yes | Yes | |||
D-SE4 | MK1 Diesel | Diesel, 7% of RME | Yes | |||||
D-SE5 | MK1 Diesel | Diesel, 7% of RME | Yes | |||||
D-SE6 | MK1 Diesel | Diesel, 7% of RME | Yes | |||||
D-SE7 | MK1 Diesel | Diesel, 7% of RME | Yes | |||||
HVO1 | HVO100 | 100% hydrogenated vegetable oil | Yes | Yes | Yes | Yes | ||
HVO2 | HVO100 | 100% hydrogenated vegetable oil | Yes | |||||
HVO3 | HVO100 | 100% hydrogenated vegetable oil | Yes | |||||
HVO4 | HVO100 | 100% hydrogenated vegetable oil | Yes | |||||
MGO-1 | Marine Gas Oil | Unknown claim | Yes | Yes | ||||
MGO-2 | Marine Gas Oil | Unknown claim | Yes | Yes | Yes | Yes | ||
D-EU1 | EU Diesel | Diesel, 6% RME | Yes | Yes | Yes | Yes | ||
D-EU2 | EU Diesel | Diesel, 6% RME | Yes | Yes | Yes | Yes | ||
EO1-1 | Fuel oil no 1 | Unknown claim | Yes | Yes | Yes | Yes | ||
EO1-2 | Fuel oil no 1 | Unknown claim | Yes | Yes | Yes |
EXPERIMENTAL
Fuels
A total of 31 fuels were analyzed for chemical composition [Table 1]. All fuels were acquired at fuel stations or fuel oil incinerators in Sweden. The EU diesels were acquired in Germany and Italy, and the marine gas oil (MGO) samples were acquired in Sweden, and in Trinidad and Tobago. From the total 31 fuels, specific fuels were selected for further tests. For the odor test, twelve fuels were selected to represent a diverse range of compositions in terms of hydrocarbon origins, additives, bio-components, and ethanol content. This selection aimed to assess how each substance or group of substances contributed to the overall odor, ensuring a comprehensive evaluation across varying fuel types. For the ecotoxicity test, six fuels were selected to represent the major product groups available in the market, providing insights into the ecological impact of different fuel categories. Additionally, two standard fuels and one custom-made were used in evaporation tests, chosen to provide a range of ethanol and ether concentrations. All prior information available on the composition of the fuels was limited to the consumer information provided by fuel producers [Table 1]. More information about the legal framework on fuels in Sweden and Europe can be found in Supplementary Materials [Supplementary Table 1].
Overview of the test execution
The test process began with a chemical analysis of the fuels using gas chromatography-mass spectrometry (GC-MS), focusing on 53 selected substances categorized according to SPIMFAB substance groups
Figure 1. Overview of the execution of tests, including mixing water (1), settling to produce a water/fuel mixture (WAF) (2), which is analysed (3.1), diluted for odor tests (3.2), ecotoxicological tests of crustaceans, algae and bacteria (3.3), and evaporation experiments (3.4), respectively. WAF: Water-accommodated fraction.
The first step involved mixing a significant proportion of fuel with water, chosen to simulate a severe spill scenario. This blending process ensured thorough dispersion of fuel components within the water. Subsequently, the mixture was left to settle, which was crucial for the formation of a stable water-accommodated fraction (WAF), where non-dissolved fuel components separated from the water. The WAF underwent chemical analysis using GC-MS to identify and quantify a diverse range of substances crucial for interpreting odor and ecotoxicity results [Supplementary Section 2]. A portion of the WAF underwent odor testing to determine threshold levels at which odors became noticeable, while ecotoxicological tests on selected fuels targeted crustaceans, algae, and bacteria to provide a comprehensive view of ecotoxicity across different trophic levels. Finally, to understand how volatile fuel components evaporate from the water surface under different temperatures and ethanol concentrations, evaporation experiments were conducted on the WAF to model the fate of fuel spills in aquatic environments under varying conditions of temperature and ethanol concentrations.
Sample preparation and extraction
To prepare the test solution, 500 mL of tap water and 50 mL of each fuel sample were combined in a 1 L Duran bottle fitted with a Teflon-lined screw cap. Mixing was achieved using a Rotier ra20 from Gerhardt, rotating at approximately 20 rpm for approximately 15 h. Subsequently, the mixture was transferred to a separator funnel and allowed to settle for about 4 h. The resulting aqueous phase containing the fuel, known as the WAF, was carefully gathered into a new Duran bottle, and stored at -20 °C before further sample preparation. This method ensured the thorough mixing of the fuel with water and the subsequent separation of the WAF for analysis.
To quantify the concentrations of selected fuel hydrocarbons in the WAF, liquid-liquid extraction was employed. Each WAF sample (4 mL) was extracted with 1 mL of dichloromethane (DCM) inside a pyrolyzed (at 400 °C overnight) glass tube (25 mL) to prevent external contamination. Prior to extraction, 250 ng of surrogate internal standard (IS) consisting of toluene-d8 and hexadecane-d34 were added, followed by 1 min of vortex mixing. The organic phase (1 mL) was then transferred to a pre-cleaned Eppendorf tube, where 200 mg of Na2SO4 was added to eliminate any residual water. After vortexing for 1 min, the tubes underwent centrifugation at 14,000 rpm for 10 min to guarantee recovery of DCM without Na2SO4 residues. Finally, 250 ng of volumetric IS (benzene-d6) was added for recovery calculation before injection. All organic solvents used, sourced from Rathburn Chemicals Ltd (Walkerburn, UK), were of high-performance liquid chromatography (HPLC) grade and pre-tested to ensure quality and purity.
For the quantification of selected hydrocarbons in the fuel samples, a direct dilute and shoot quantitative analysis method was employed[17]. To prevent analytical instrumentation overload, each fuel sample underwent a two-step dilution process, resulting in a 400-fold dilution. This involved diluting each sample to 1 mL of DCM within a pre-pyrolyzed glass vial (1.5 mL) and adding 250 ng of each IS, including toluene-d8, hexadecane-d34, and benzene-d6. Calibration standards of different concentrations (1-5,000 µg/L) in DCM were used for all the compounds [Supplementary Table 2].
Instrumental analysis and quantification
The GC-MS analysis was conducted using an Agilent 5977B GC-MSD system in electron impact ionization mode (EI), specifically configured with an HP-PONA column (length 50 m; inner diameter 0.2 mm; stationary phase thickness 0.5 µm). This setup facilitated the separation of petroleum process products via detailed hydrocarbon analysis, encompassing paraffins, isoparaffins, aromatics, naphthalenes, and olefins. The analysis utilized the PONA and PIANO standard mixes provided by LGC Standards Ltd. The auto-injector (Agilent 7683B) facilitated pulsed splitless mode injection of 1 µL volume samples. MassHunter software version B.04.00 was utilized for quantitative analysis. The quantified substances [Supplementary Table 2] were categorized into groups, including ethers, BTEX compounds, aromatics, aliphatic compounds, and esters. Notably, MTBE, ethyl tert-butyl ether (ETBE), and tertamyl-methyl ether (TAME) were common ethers identified. Due to the project’s focus on odor properties, particular emphasis was placed on achieving optimal resolution for low molecular weight substances such as BTEX and ethers, as these were expected to contribute significantly to odor[7,18]. The BTEX group, comprising benzene, toluene, ethylbenzene, and xylene isomers, along with other aromatic compounds like 3-ethyl-toluene and propyl- and trimethyl-benzene, were of interest. Aliphatic compounds analyzed ranged from hexane (C6) to tetracosane (C24), with challenges noted for shorter alkanes due to solvent peak interference in the GC chromatographic separation caused by water extractions and fuel dilution using DCM. Additionally, cyclohexane and n-hexane evaluations were hindered by minor impurities in the DCM solvent. Quantification of 17 selected PAHs [Supplementary Table 3], including those recommended by the Environmental Protection Agency (EPA)[19] which are frequently found in environmental monitoring samples, was also performed using HPLC with fluorescence detection (HPLC-FL) and diode array detection (DAD)[20]. A mixture of fluorinated PAHs was used as surrogate IS and calibration standards of different concentrations (0.1-1,500 µg/L) in acetonitrile. The reference method utilized was according to the Swedish Standard SS-EN 17503:2022[21].
Odor threshold test
Odor threshold tests were conducted in accordance with the European standard SS-EN 1622:2006[22], utilizing the paired test method (10.2.2) outlined in the comprehensive procedure (10.3.2). The standard and this report adopt the threshold odor number (TON) as the measure of dilution beyond which the sample no longer exhibits a detectable odor. For the assessment of odors, test panels were formed by the four participating drinking water producers (DWP), comprising personnel from production, laboratory, and management, totaling 3-11 members per panel. Each panelist received a series of dilutions of the WAF, with each series containing five different dilutions. Panelists indicated which bottle, if any, emitted an odor compared to a reference water sample. These assessments were conducted on two separate occasions, with DWPs evaluating fuel types based on Table 2. IVL prepared the various dilution series and dispatched them to the drinking water producers (DWP). Following the geometric progression prescribed in the protocol, the dilutions were arranged in a geometric series. Dilution factors were set to 2 in the first and 2.5 in the subsequent rounds (i.e. second and third). This adjustment aimed to widen the concentration disparity between consecutive dilutions, enhancing sensitivity. However, not all results from the odor tests were deemed usable. In many instances, panel members detected an unusual scent in the weakest dilution or failed to perceive any odor throughout the series. Consequently, the conventional definition of odor threshold[22], which necessitates the geometric mean of all panel members’ results, could not be applied. Instead, the threshold was redefined as the dilution at which 50% of panelists detected an odor - a more practically feasible approach employed in odor threshold determinations by various sources[23,24]. This adjustment facilitated the inclusion of more data in the analysis. Concerning the methodology, it is acknowledged that there might be some bias in the odor test outcomes. This bias stems from the inability to fully randomize the odor testing procedure, particularly in the aspect of smelling reference water and samples. Panel members were aware of which bottle served as the reference, rendering complete randomization impractical. Any potential bias would likely manifest as a panel’s early detection of odor, skewing the results.
Fuel types that were sent to each DWP (X: first round; Y: second round; Z: third round)
Fuel | DWP 1 | DWP 2 | DWP 3 | DWP 4 |
HVO1 | (Y) | X | X (Y) | |
D-SE1 | X (Y) | (Y) | X | |
D-SE2 | X (Y) | X (Y) | ||
D-SE3 | X (Y) | (Y) | X | |
E85-1 | X (Y) | X (Y) | ||
P98-1 | X | (Y) | X | (Y) |
P95-1 | X (Y) | X (Y) | ||
P95-2 | X | X (Y) | (Y) | |
P98-2 | Z | Z | ||
MGO-2 | Z | Z | ||
D-EU1 | Z | Z | ||
D-EU2 | Z | Z |
Statistical analysis
The analysis of data on odor thresholds and concentrations in the WAF involved utilizing a linear mixed model, with the TON as the response variable. WAF concentrations served as explanatory variables, while fuel type was considered a random effect to account for correlated observations from the same fuel type. Due to the dataset containing more variables (chemicals) than observations (odor test results), selecting a model with numerous chemical compounds as explanatory variables was infeasible. Therefore, a smaller selection of chemical compounds was chosen by investigating correlations between each chemical and the odor threshold, and between each chemical and every other chemical. Lasso regression, a variable selection technique, was employed to automate the selection process by reducing coefficient estimates of non-important variables to zero. The results obtained from Lasso regression guided the construction of the linear predictor in the mixed model. Notably, in all statistical models, the upper bound concentration was utilized if the concentration fell under the detection limit of the chemical analysis. This approach ensured a robust analysis of the relationship between WAF concentrations of selected chemical compounds and odor thresholds, accounting for the complexity of the dataset and addressing the challenge of variable selection in models with limited observations.
Ecotoxicity to freshwater organisms
Ecotoxicity assessment of freshwater organisms involved evaluating seven fuels, wherein water/fuel solutions were prepared separately from those used for odor threshold tests. A ratio of 100 mg of fuel per
RESULTS AND DISCUSSION
Chemical analysis of fuels
The composition of diesel and petrol fuels was analyzed using GC-MS, focusing on 53 selected substances categorized according to SPIMFAB substance groups [Supplementary Table 2]. These substances were chosen for their significance in interpreting results related to odor and to illustrate the proportion of light and heavy substances in the fuels. While these substances offer insight into the chemical makeup, they do not represent the entirety of the fuels. Results align with European standards and the Swedish Fuel Act, showing lower aromatic compound concentrations in MK1 diesel and HVO fuels compared to EU Diesel, Fuel oil 1, and Marine Gas Oil [Figure 2]. MK1 diesel variants containing biocomponents like HVO or RME exhibit variance in ester concentrations, absent in Fuel oil 1 or MGO. Petrol types display a substantial presence of aromatic compounds across all samples, compliant with the Fuel Act’s allowed limit [Figure 2]. Additionally, blends like E85 and 98 octane petrol contain ethers below permissible levels, impacted by ethanol content regulations. The box and whisker plot presented in Supplementary Figure 3, which includes products with three or more samples per product group, reveals that petrol demonstrates greater heterogeneity in its main constituents compared to diesel. This is evidenced by the wider quartile spans observed within the respective product groups. Consequently, the composition of diesel is considered more homogeneous than gasoline.
Figure 2. Total concentrations of the SPIMFAB substance groups in diesel and diesel-like (A) and petrol and petrol-like (B) fuels. Note that suns of substance groups only contain the quantified substances included in this study.
Moreover, eight fuel samples were chosen for PAH analysis [Supplementary Table 3]. The selection criteria were informed by suspicions of PAH presence in these fuels, intentionally excluding Swedish MK1 diesel and HVO from the analysis in compliance with stringent legislation prohibiting PAHs in these fuel types. The result of the analysis is shown in Figure 3. European diesel samples revealed a moderate presence of these compounds, with medium-molecular-weight PAHs like phenanthrene emerging significantly in one of the samples, indicating a uniformity that might reflect regulations governing European fuel standards. Further insights were gathered from comparing fuel oils produced across different time frames. The EO1-1 sample, a modern fuel oil, contained considerably lower amounts of the PAHs than the EO1-2 sample produced during the 1970s. Another difference was observed in the Marine Gas Oil samples, where the product from Sweden, MGO-1, contained a higher concentration of naphthalene than the Trinidad
Figure 3. Concentrations of PAHs in oeight fuel samples grouped as PAH-L (low molecular weight), PAH-M (medium molecular weight) and PAH-H (high molecular weight). PAH: Polycyclic aromatic hydrocarbon.
Overall, the quantified compounds represented a minor proportion of all the compounds present in the fuels due to the limited number of compounds available in the commercial standard mixtures for spark ignition fuels that were used. These mixtures do not include every chemical found in the fuels but cover a significant portion relevant to the study. As a result, many hydrocarbons were not accounted for in the findings. The goal was to identify which chemicals contributed to odor, allowing for the exclusion of compounds unlikely to affect odor significantly. Additionally, the relationship between the chemicals’ toxicity and their composition was not examined, meaning chemicals that might have harmful environmental effects were not focused on.
The total concentration of quantified components in products D-SE3 and HVO1 appears lower compared to D-SE2 and D-SE1, as evidenced by the span of the bars in Figure 2. Despite this, the selected compounds for quantification portray a broad spectrum of chemicals present in fuels, chosen based on the availability of standards for paraffins, isoparaffins, aromatics, naphthenes, and olefins. While these compounds provide insight into structurally similar compounds, the vast complexity of fuel chemistry cannot be entirely captured. Supplementary Figure 4 demonstrates that the analyzed fuel contains a diverse array of hydrocarbons not included in the calibration mixture, ranging from low boiling point compounds to branched long-chain aliphatics. This complexity likely contributes to the low proportion of quantified compounds in HVO-1. Despite limitations, the analysis still targets representative hydrocarbons in each boiling point fraction, aiming to encompass substances of similar molecular size to those not directly quantified. The observed differences in aromatic and polyaromatic hydrocarbons, as well as ether content among the various fuel types, suggest significant variations in odor and ecotoxicological profiles.
Chemical analysis of fuel-water mixtures
The solubility of petrol-specific substances in water appears higher compared to diesel fuels. This difference is evident from the analysis of total organic carbon (TOC), which shows the difference in total solubility of fuels, where higher concentrations of ethanol in P95-3 (10%) and E85-3 (85%) are reflected in the increased TOC [Supplementary Table 4]. The same trend is indicated by a comparison between Figures 2 and 4. Petrol fuels dissolve more readily in water than diesel, primarily because of the presence of dissolved ether and BTEX components in the fuel. Additionally, specific compounds such as the branched alkane
Figure 4. Total concentrations of the determined substances in the WAF per SPIMFAB substance group. Note that sums of substande groups only contain the quantified substances in this study and not the sum of the entire substance group. WAF: Water-accommodated fraction.
Figure 5 illustrates the concentration levels of specific aromatic compounds in fuels alongside their corresponding levels in the resulting WAF. These compounds are predominantly present in petrol fuels. Notably, less substituted aromatic compounds exhibit a higher solubility in water compared to more substituted compounds, influencing the proportions between fuel and WAF concentrations. Conversely, the levels of selected aliphatic compounds in fuels and WAF, predominantly present in diesel fuels, do not display noticeable differences in the relative proportion during the transfer from fuel to water.
Figure 5. Total concentrations of selected aromatic (A) and aliphattic (B) compounds in the fuels (left) and in the WAF (right). WAF: Water-accommodated fraction.
Ethanol and RME in fuels significantly enhance the dissolution of hydrocarbons in water. The ethanol-containing fuel (E85-1) exhibits the highest concentrations of predominant petrol components (BTEX, aromatic components, linear, branched, and cyclic short alkanes and alkenes) in the WAF, despite only comprising 15% petrol. This probably derives from ethanol's solvent properties, facilitating phase transfer and increased solubility in the receiving WAF. However, it is worth noting that the closed system utilized in testing, with limited fuel and water phase volumes, may potentially exaggerate the solubility of petrol compounds compared to real-world scenarios, yet the observed trend remains relevant in case of a spill event. Moreover, the presence of longer chain aliphatic compounds in the water samples appears to follow with the concentration of the RME component (oleic acid methyl ester) in the fuel, despite the initial concentrations of the aliphatic compounds in the fuel being similar. Hence, the WAF from D-SE2 and
A strong correlation between ether concentration in WAF and fuel content was found [Supplementary Figure 5]. Derived from the chemical analyses of fuels and their corresponding WAF, an ether transfer equation was generated between concentrations in fuel and WAF. The most important variable explaining the ether concentration of the WAF was the ether concentration in the raw fuel. The scatterplot of the total concentration of ether in the WAF vs. the total concentration in fuels on a logarithmic scale shows the linear relation between the concentrations and the regression equation describing ether transfer from fuel to WAF. Given the low number of observations, the equation still gives a good prediction of the solubility of ether from fuel to water.
In conclusion, the most striking observation is that ether is readily soluble in water, independent of the ethanol content in the fuel, indicating its very effective solubility. Additionally, the petrol content in the water phase of E85 is comparable to or even higher than that observed in water mixed with pure petrol fuels. Conversely, diesel fuels demonstrate very low solubility in water, highlighting a significant difference in how these fuel types interact with water.
Determination of odor thresholds and prediction
Fuels E85-1, P98-2, and P98-1 were notably distinguishable by odor. The TON in Table 3, which summarizes the results of the odor tests, is the dilution ratio beyond which a sample has no perceptible odor. If TON = 10, the WAF and reference water mixture consisted of 9-part reference water and 1-part WAF (i.e. dilution ratio of 10%). A high TON implies that odor was detected at a low proportion of WAF in the sample; therefore, the odor was potent. These three fuels stood out with notably high TON values, thus minimal odor thresholds. These are the 85% ethanol petrol and the 98-octane petrol, respectively. Considerable variability in odor thresholds was observed among panel members evaluating the same fuel type [Figure 6]. In the initial round, several panelists detected an odor in the most diluted sample within the series, particularly for E85-1 and P98-1 fuels. Remarkably, during the first round, a dilution factor of 2 was employed, while a dilution factor of 2.5 was utilized in subsequent rounds. This adjustment resulted in more significant steps between each dilution, leading to a wider range of concentrations and larger discrepancies in odor threshold among panel members. TON values [Table 3] were calculated by summarizing the data for each fuel type.
Figure 6. The threshold odor number, on a logarithmic scale, indicates whcre an odor first was noticed by cach panel member. Triangles indicate a anel member noticing an odor in the most diluted sample, and diamonds indicate no odor in the least diluted sample. Dots indicate a panel member noticing an odor in any other bottle. In theIst round, a dilution factor of 2 was used, and in the 2nd and the 3rd round, a dilution factor of 2.5 was used. One cur per fuel type for better visual separation. WAF: Water-accommodated fraction.
TON averaged over fuel types, the dilution ratio where 50% of the panellists did not sense an odor and the MTBE concentration at that dilution
Fuel | TON | Dilution ratio (%)* | Concentration MTBE (µg/L) |
HVO1 | 62 | 1.6 | 13 |
P95-1 | 250 | 0.40 | 8.5 |
D-SE3 | 260 | 0.39 | 0.22 |
D-SE1 | 300 | 0.33 | 1.7 |
D-SE2 | 300 | 0.33 | 5.5 |
P95-2 | 490 | 0.21 | 8.1 |
MGO-2 | 1,500 | 0.066 | 0.0031 |
D-EU2 | 1,500 | 0.066 | - |
D-EU1 | 9,500 | 0.011 | 0.011 |
E85-1 | 24,000 | 0.0042 | 4.0 |
P98-2 | 24,000 | 0.0042 | 3.4 |
P98-1 | 60,000 | 0.0017 | 1.4 |
When predicting odor, it was found that only a few of the quantified substances in the fuels had a strong correlation with the TON value. The substances with correlations above 0.6 are depicted in a correlation matrix [Supplementary Figure 6]. Initial analysis suggested that the relationship between TON and concentrations is best modeled on a logarithmic scale. Consequently, the natural logarithm was applied to both TON and the concentrations of chemicals. The primary observation reveals that the substances correlated with the odor threshold are also positively associated. However, there are exceptions, such as the correlations between 1-heptene and ETBE, as well as 1,2,4,5-tetramethylbenzene and ETBE, which exhibit lower correlations. In instances where 50% of the odor panel identified an odor, the MTBE concentration in the samples was measured at 1.4 µg/L in P98-1 and 4.1 µg/L in E85-1. These concentrations fall below the odor threshold reported by WHO[7], which stands at 15 µg/L. The concentrations of other ethers in the WAF are too minimal to account for the variance. Consequently, the results suggest that the low threshold could be a result of MTBE being part of a mixture of other substances that either add to or enhance the odor. However, statistical evaluation could not be performed due to the confined number of samples.
MTBE levels demonstrate a significant correlation with TON, indicated by an R2 value of 0.7
The results from the odor tests, combined with the solubility experiments, lead to the conclusion that the total volume of ether in a spill primarily determines the risk posed to the water source. This is because odor intensity is proportional to ether concentration, and ether concentration in water is directly proportional to ether concentration in the fuel. In cases of surface water contamination, existing technologies are equipped to handle hydrophobic substances on the water surface. However, soluble compounds like ethers present a significant challenge, as there are no efficient methods to remove them from open water bodies. This necessitates reliance on preventive measures or advanced treatment processes, such as sorption filters, by drinking water producers to ensure water safety and quality.
Ecotoxicological test results
All ecotoxicological tests were performed on WAF attained from mixing fuel and water and extracting the water phase. In some tests, the concentration is expressed as the loading rate, which is the amount of WAF per liter of water, and in some tests, it is noted as a percentage of WAF in the solution. All tests focus on the effective toxicity of the fuel product, which means that the effects of potential solvents in the product, such as ethanol, are desired outcomes. The results from the analysis of TOC show the difference in total solubility of fuels, where higher concentrations of ethanol in P95-3 (10%) and E85-3 (85%) are reflected in the increased TOC [Supplementary Table 4].
The first test aimed to evaluate the impact of various fuels on the growth of freshwater microalgae. Exponentially growing algae cultures were subjected to WAF of the test substances for a duration of 72 h. Despite the relatively short test period, effects spanning several generations may be evaluated using this test. The primary response measured was the reduction in growth observed across a series of algal cultures (test units) exposed to varying concentrations (loading rates) of the test substances. This response is evaluated by comparing the exposure concentration to the average growth of an unexposed control culture. Growth and growth inhibition are determined based on measurements of algal biomass, typically represented as dry weight per unit volume, recorded over time. Cell counts (cells/mL) served as a proxy for dry weight per OECD Test Guideline No. 201. The test endpoint was the inhibition of growth, expressed as the logarithmic increase in biomass, or the average specific growth rate, during the exposure period. On the contrary, fuels HVO1, D-SE1, P95-3, and D-EU1 exhibited no notable contrast in comparison to the control (P > 0.05), leading to the establishment of their NOEC at 100 mg/L, which represents the highest concentration tested and indicates no observed toxic effect. Figure 7 displays the growth of algae over 72 h for the most concentrated solutions utilized in the tests.
Figure 7. The biomass (cells/mL) in the highest test concentration used (100 mL loading rate) after 0, 48 and 72 h of exposure to the fuel/water solutions of P95-3, P98-2, E85-2, D-EU1, Eol-1, HVO1 and D-SE1.
The microtox test aimed to assess general toxicity by measuring the inhibition of bacterial activity through the suppression of luminescence in genetically modified bacterial cells. Luminescence inhibition serves as an indicator of the cells’ toxic response. Results from the microtox tests revealed significant luminescence inhibition in bacteria when exposed to petrol fuels [Figure 8]. For a solution comprising 50% WAF, absolute inhibition of luminescence was observed for E85 and P95 samples, while P98 exhibited nearly complete inhibition. EU-diesel and fuel oil also displayed luminescence inhibition at 50% WAF, albeit not when diluted to 4.5%. Conversely, HVO and MK1 diesel (D-SE1) showed minimal to no inhibition when used as a 90% WAF solution, except for a minor inhibition observed for day 28 after 5 min. Toxicity to Daphnia magna, indicated by reproduction inhibition, was observed across all fuel samples [Supplementary Figure 8]. In the case of HVO-1, the EC10 (the concentration at which 10% of the organisms are affected) was attained at 11 Vol% WAF, while the EC50 was reached at 69 Vol%, suggesting mild inhibitory effects of the HVO fuel. Conversely, the EU-diesel D-EU1 reached the EC50 at just 1% WAF, indicating very strong toxic properties. The uptake of toxic substances in crustaceans such as Daphnia magna can occur through direct absorption via gills and body surfaces, or through ingestion of contaminated food. The D-EU1 sample contained hydrophobic aromatic substances, which may have adsorbed to the food, potentially increasing the exposure of the crustaceans compared to other fuels.
Figure 8. Inhibition of luminescence in a mictotox test for seven fuel sams. HVO-1 and D-SEI were tested using 90% WAF solution, while the others were tested using 50 % and 4.5% WAF solutions. Each solution was tested for 0 and 28 days,and 5, 10 and 15 min. The incubation conditions are controlled tomaintain the bacterial culture’s viability, providing a nutrient-rich medium, ensuring proper aeration, and regular subculturing. Accurate and consistent bacterial cultures are essentiaI for reliable microtox test results. WAF: Water-accommodated fraction.
The microbial degradation test utilized wastewater sludge inoculated in WAF solutions and was intended to evaluate how well the sludge could degrade organic material in the presence of fuels. However, due to insufficient levels of DOC in the test solutions, the results were not reliable and, therefore, could not be used for assessment. None of the samples exhibited acceptable levels of DOC, and no interference with the degradation process of DOC was observed [Supplementary Figure 9] for the HVO-1 and D-SE1 samples; the water solubility and, consequently, the DOC levels in the WAF were under the permissible range for the analytical methodology. These levels were also near the lower limit for D-EU1 and EO1-1 samples. The method recommended a DOC range of 10-40 mg/L, yet the concentration of the test material fell below
The ISO 8192 method[30] was employed to evaluate the inhibitory effects of WAF on the respiration rate of activated sludge microorganisms. This involved exposing the sludge to WAF concentrations of 0.5%, 5%, and 50% and monitoring changes in oxygen consumption, serving as an indicator of the fuels’ potential toxicity to the microbial community. Results indicated that at a 50% WAF concentration, all samples, except EO1-1, exhibited inhibitory effects on the activated sludge. In contrast, EO1-1 demonstrated negative inhibition, suggesting it acted as a growth substrate for the activated sludge community [Figure 9]. Overall, petrol fuels exhibited the highest toxicity to the activated sludge among the tested samples, indicating that petrol was the most harmful. Since activated sludge is a mixture of microorganisms and organic matter often used in wastewater treatment to break down pollutants[32], if a substance is toxic to activated sludge, it can impair the sludge’s ability to treat wastewater effectively, leading to environmental and public health risks. In that sense, spill events involving petrol fuels that risk entering the sewage system through storm water or intrusion from contaminated surroundings are particularly dangerous because they can disrupt the wastewater treatment process by damaging the microbial communities in activated sludge.
Figure 9. The inhibition of respiration of active sludge from different doses of WAF (Vol %) attained from five different fuels. WAF: Water-accommodated fraction.
Finally, this study started exploring the evaporation rates of ethers in water-fuel mixtures and their impact on odor properties. Our research involved bench-scale aeriation tests across various temperatures and ethanol concentrations, revealing significant findings on the evaporation patterns of MTBE and ETBE in different fuel blends. This was the first step to introduce a linear regression model to predict ether evaporation, offering valuable insights for managing fuel spills, particularly in cold environments. However, the model requires further research before being applicable to real-world scenarios of fuel spills, providing valuable insights for practical applications in fuel handling and environmental management.
CONCLUSION
A method to quantify many of the chemicals present in fuels has been successfully developed and applied, enabling a more accurate assessment of their environmental and health impacts. This is vital for complying with regulations, improving fuel compositions, reducing pollution, and supporting informed decision making to develop cleaner and safer energy sources. Thus, the evaluation of the solubility of the components in modern fuels in water was further applied in studies determining correlations between fuel content and odor, toxicity and the evaporation of the components at different temperatures. As fuel compositions have been changed in recent decades to reduce the emissions of fossil greenhouse gasses, investigations of how these changes can affect risks for humans and the environment are much needed. Petrol-specific substances were found to exhibit higher solubility in water compared to those in diesel, with ethanol in petrol and rapeseed methyl ester in diesel aiding hydrocarbon dissolution into water. However, ethanol did not significantly increase ether solubility, and a strong correlation between ether concentration in water and fuel content was observed, allowing for the prediction of ether concentration in WAF solely from fuel ether concentration. Only a limited number of substances were strongly associated with odor, with the concentration of dissolved ethers, especially MTBE, shown to be a reliable indicator of fuel odor in water. This raises concerns when using odor as a proxy for the presence of fuel components in drinking water, as fuels with low or no addition of MTBE or other ethers may go undetected. Evaporation experiments revealed that ether evaporation can be predicted based on WAF ether concentration, water temperature, and ethanol content. Cold water conditions lead to a reduction in ether evaporation rates, resulting in a decrease in ether concentration over time, depending mainly on diffusion, with consequent risks of more widespread pollution. European diesel samples revealed a moderate presence of PAHs, but 95 and 98-octane fuels displayed considerable amounts of naphthalene, which may have a toxic effect on aquatic organisms. Ecotoxicological tests indicated reproduction inhibitions in crustaceans across all fuel samples, with petrol fuels exhibiting greater algae growth inhibition and bacterial luminescence decrease compared to diesel. Despite the indication that petrol was the most harmful, none of the fuels significantly inhibited activated sludge respiration, suggesting its resilience due to diverse bacterial flora. The increased water solubility of fuels due to changes to the fuel composition necessitates a review of risk management concerning fuel spills. For drinking water producers, fuels containing water-soluble ethers pose the most significant risk, while modern diesel poses a marginal concern for surface water-based raw water sources due to low solubility and reduced toxic substance levels. In freshwater ecosystems, water-soluble petrol-associated substances and hydrophobic toxic substances in fuel oil or EU diesel have the most severe effects during a spill, although MK1 and HVO diesel minimally affected test organisms compared to the control.
DECLARATIONS
Acknowledgments
We would like to extend our sincere thanks to Alexis Marcou, the talented graphic designer who skillfully created the graphical abstract for this work.
Authors’ contributions
Conceptualization, methodology, formal analysis, investigation, writing - original draft preparation, review and editing, visualization, funding acquisition: Strandberg J
Methodology, formal analysis, investigation, writing - original draft preparation, review and editing, visualization: Waldetoft H
Methodology, formal analysis, investigation: Egelrud L
Methodology, formal analysis, investigation, writing - original draft preparation, review and editing: Backlund A
Investigation, writing - original draft preparation, review and editing: Cascone C
Methodology, formal analysis, investigation, writing - original draft preparation, review and editing, visualization: Thorsén G
Methodology, formal analysis, investigation: Potter A
Methodology, formal analysis, investigation, writing - original draft preparation, review and editing, visualization: Giovanoulis G
Availability of data and materials
Additional data and information about this study are presented in the Supplementary Materials. The raw data are summarized in Supplementary Tables 5-7.
Financial support and sponsorship
The research described was generously funded by a consortium of organizations involved in environmental management, including the Swedish Coastal Guard, Norrvatten, Göteborg Kretslopp & Avfall, Sandviken Energi, Stockholm Vatten och Avfall, Svenskt Vatten, and the Stiftelsen Institutet för Vatten- och Luftvårdsforskning (SIVL).
Conflicts of interest
Giovanoulis G is an Editorial Board member of Journal of Environmental Exposure Assessment, while the other authors have declared that there are no conflicts of interest.
Ethical approval and consent to participate
Not applicable.
Consent for publication
Not applicable.
Copyright
© The Author(s) 2024.
Supplementary Materials
REFERENCES
1. Annual report 2023. (in Swedish) Available from: https://www.brandskyddsforeningen.se/om-oss/verksamhetsberattelse/. [Last accessed on 10 Sep 2024].
2. Strandberg J, Waldetoft H, Giovanoulis G, Egelrud L, Thorsén G, Potter A. Odour and ecotoxicity in water from fuels of varying content of non-fossil components: odour threshold values, predictive modelling and ecotox data. 2022. Available from: https://www.ivl.se/english/ivl/publications/publications/odour-and-ecotoxicity-in-water-from-fuels-of-varying-content-of-non-fossil-components-odour-threshold-values-predictive-modelling-and-ecotox-data.html. [Last accessed on 7 Sep 2024].
3. ATSDR. Toxicological profile for total petroleum hydrocarbons (TPH). 1999. Available from: https://www.atsdr.cdc.gov/toxprofiles/tp123.pdf. [Last accessed on 7 Sep 2024].
4. Drivmedelslag (2011:319). (in Swedish) Available from: https://www.riksdagen.se/sv/dokument-och-lagar/dokument/svensk-forfattningssamling/drivmedelslag-2011319_sfs-2011-319/. [Last accessed on 7 Sep 2024].
5. Pires A, Han Y, Kramlich J, Garcia-Perez M. Chemical composition and fuel properties of alternative jet fuels. BioResources 2018;13:2632-57. Available from: https://bioresources.cnr.ncsu.edu/resources/chemical-composition-and-fuel-properties-of-alternative-jet-fuels/. [Last accessed on 7 Sep 2024]
6. IARC. Benzene - IARC Monographs on the Evaluation of Carcinogenic Risks to Humans. Volume 120. 2018. Available from: https://publications.iarc.fr/Book-And-Report-Series/Iarc-Monographs-On-The-Identification-Of-Carcinogenic-Hazards-To-Humans/Benzene-2018. [Last accessed on 7 Sep 2024].
7. WHO. Methyl tertiary-butyl ether (MTBE) in drinking-water. 2005. Available from: https://cdn.who.int/media/docs/default-source/wash-documents/wash-chemicals/mtbe200605.pdf?sfvrsn=a4435114_4. [Last accessed on 7 Sep 2024].
8. U.S. Environmental Protection Agency. Government response to the BP oil spill - odors from the BP Spill. 2010. Available from: https://archive.epa.gov/emergency/bpspill/web/pdf/odorfactsheet.pdf. [Last accessed on 7 Sep 2024].
9. Livsmedelsverket. LIVSFS 2022:12. Available from: https://www.livsmedelsverket.se/om-oss/lagstiftning1/gallande-lagstiftning/livsfs-202212. [Last accessed on 7 Sep 2024].
10. SEPA. Odour guidance 2010. Available from: https://www.sepa.org.uk/media/154129/odour_guidance.pdf. [Last accessed on 7 Sep 2024].
11. Laffon B, Pásaro E, Valdiglesias V. Effects of exposure to oil spills on human health: updated review. J Toxicol Environ Health B Crit Rev 2016;19:105-28.
12. Govindarajan SK, Mishra A, Kumar A. Oil spill in a marine environment: requirements following an offshore oil spill. Rud Geol Naft Zb 2021;36:1-9.
13. Gorman Ng M, Cherrie JW, Sleeuwenhoek A, et al. GuLF DREAM: a model to estimate dermal exposure among oil spill response and clean-up workers. Ann Work Expo Health 2022;66:i218-33.
14. Ehigbor MJ, Iwegbue CMA, Eguavoen OI, Tesi GO, Martincigh BS. Occurrence, sources and ecological and human health risks of polycyclic aromatic hydrocarbons in soils from some functional areas of the Nigerian megacity, Lagos. Environ Geochem Health 2020;42:2895-923.
15. Eklund RL, Knapp LC, Sandifer PA, Colwell RC. Oil spills and human health: contributions of the gulf of Mexico research initiative. Geohealth 2019;3:391-406.
16. Drivmedel 2020. ISBN978-91-7993-039-4. Eskilstuna, Sweden. (in Swedish) Available from: https://energimyndigheten.a-w2m.se/System/TemplateView.aspx?p=Arkitektkopia&id=ab21d64a1cd74d0f8dec7bbcd96aaf2e&view=693&q=drivmedel. [Last accessed on 10 Sep 2024].
17. Johnson KJ, Rose-Pehrsson SL, Morris RE. Characterization of fuel blends by GC-MS and multi-way chemometric tools. Petrol Sci Technol 2006;24:1175-86. Available from: https://www.tandfonline.com/doi/citedby/10.1081/LFT-200048192?scroll=top&needAccess=true. [Last accessed on 18 Sep 2024]
18. WHO. Guidelines for drinking-water quality. 2008. Available from: https://sswm.info/sites/default/files/reference_attachments/WHO%202008.%20Guidelines%20for%20drinking%20water%20quality.pdf. [Last accessed on 7 Sep 2024].
19. Keith LH. The source of U.S. EPA’s sixteen PAH priority pollutants. Polycycl Aromat Compd 2015;35:147-60.
20. Świt P, Orzeł J, Maślanka S. Monitoring of PAHs in simulated natural and artificial fires by HPLC-DAD-FLD with the application of Multi-Component Integrated calibration method to improve quality of analytical results. Measurement 2022;196:111242.
21. Swedish Institute for Standards (SIS). Soil, sludge, treated biowaste and waste - determination of polycyclic aromatic hydrocarbons (PAH) by gas chromatography (GC) and high performance liquid chromatography (HPLC). 2022. Available from: https://www.se.byggsystem.kemi.sis.se/en/produkter/chemical-technology/analytical-chemistry/physicochemical-methods-of-analysis/ss-en-175032022/. [Last accessed on 7 Sep 2024].
22. Swedish Institute for Standards (SIS). Water quality - Determination of the threshold odour number (TON) and threshold flavour number (TFN). Available from: https://www.sis.se/en/produkter/environment-health-protection-safety/water-quality/drinking-water/ssen16222006/. [Last accessed on 7 Sep 2024].
23. Alberta Health. Odour thresholds in emergency management. 2020. Available from: https://open.alberta.ca/dataset/e08a5b0d-1e4d-41d3-88de-a0808d08f501/resource/707256d0-6681-4cc2-b798-cd1775f12330/download/health-odour-thresholds-in-emergency-management-jurisdictional-review.pdf. [Last accessed on 7 Sep 2024].
24. Danish Environmental Protection Agency. Industrial odour control. Environmental Guidelines No. 9, 2002. Available from: https://mma.gob.cl/wp-content/uploads/2017/06/Environmental-Guidelines-9-2002-Industrial-Odour-control-ONUK2651.pdf. [Last accessed on 7 Sep 2024].
25. OECD guidelines for the testing of chemicals, Section 2. Test No. 201: Freshwater alga and cyanobacteria, growth inhibition test. Available from: https://www.oecd-ilibrary.org/environment/test-no-201-alga-growth-inhibition-test_9789264069923-en. [Last accessed on 7 Sep 2024].
26. ISO 11348-3:2007. Water quality - Determination of the inhibitory effect of water samples on the light emission of Vibrio fischeri (Luminescent bacteria test). Available from: https://www.iso.org/standard/40518.html. [Last accessed on 7 Sep 2024].
27. EPA. Methods for measuring the acute toxicity of effluents and receiving waters to freshwater and marine organisms. Fifth edition, 2002. Available from: https://www.epa.gov/sites/default/files/2015-08/documents/acute-freshwater-and-marine-wet-manual_2002.pdf. [Last accessed on 7 Sep 2024].
28. Slob W, Setzer RW. Shape and steepness of toxicological dose-response relationships of continuous endpoints. Crit Rev Toxicol 2014;44:270-97.
29. ISO 7827:2010. Water quality - Evaluation of the “ready”, “ultimate” aerobic biodegradability of organic compounds in an aqueous medium - Method by analysis of dissolved organic carbon (DOC). Available from: https://www.iso.org/standard/46248.html. [Last accessed on 7 Sep 2024].
30. ISO 8192:2007. Water quality - Test for inhibition of oxygen consumption by activated sludge for carbonaceous and ammonium oxidation. Available from: https://www.iso.org/standard/37369.html. [Last accessed on 7 Sep 2024].
31. Harrell FE Jr. Regression modeling strategies - With applications to linear models, logistic and ordinal regression, and survival analysis. 2015. Available from: https://link.springer.com/book/10.1007/978-3-319-19425-7. [Last accessed on 7 Sep 2024].
Cite This Article
How to Cite
Download Citation
Export Citation File:
Type of Import
Tips on Downloading Citation
Citation Manager File Format
Type of Import
Direct Import: When the Direct Import option is selected (the default state), a dialogue box will give you the option to Save or Open the downloaded citation data. Choosing Open will either launch your citation manager or give you a choice of applications with which to use the metadata. The Save option saves the file locally for later use.
Indirect Import: When the Indirect Import option is selected, the metadata is displayed and may be copied and pasted as needed.
About This Article
Copyright
Data & Comments
Data
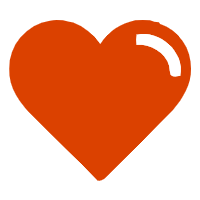
Comments
Comments must be written in English. Spam, offensive content, impersonation, and private information will not be permitted. If any comment is reported and identified as inappropriate content by OAE staff, the comment will be removed without notice. If you have any queries or need any help, please contact us at [email protected].