Recent developments in nanoparticle-based treatment for atrial fibrillation and other human cardiac arrhythmias
Abstract
This review aims to summarize the current landscape of cardiac arrhythmia therapeutics and highlight recent advances in nanoparticle-based strategies for the treatment of various human cardiac arrhythmias. A comprehensive literature review was conducted to curate and synthesize recent preclinical developments in nanoparticle-mediated therapies targeting cardiac arrhythmias. Cardiac arrhythmias represent one of the most prevalent and challenging forms of cardiovascular disease worldwide. Conventional treatments, including antiarrhythmic drugs, are often limited by suboptimal efficacy, high recurrence rates, and significant off-target toxicities. While catheter-based ablation techniques have emerged as alternative interventions, their long-term success remains inconsistent, as highlighted by outcomes from large trials such as the CABANA trial. In response to these limitations, nanoparticle-based interventions have emerged as a promising class of therapeutics. These strategies offer potential advantages, including site-specific drug delivery, reduced systemic toxicity, and novel approaches to both pharmacologic and ablative therapy. This review presents an overview of the emerging nanoparticle-based strategies for the treatment of atrial fibrillation and other cardiac arrhythmias. It also discusses recent proof-of-concept studies, evaluates the benefits and limitations of various nanoparticle formulations, and outlines key challenges and future directions for translating these technologies into clinical practice.
Keywords
INTRODUCTION
Cardiac arrhythmias are a spectrum of cardiovascular diseases (CVDs) characterized by disruptions in cardiac excitation patterns and rhythm abnormalities[1]. These disorders are among the most prevalent types of CVDs, affecting an estimated 1%-4% of the global population[2]. Atrial fibrillation (AF), the most common rhythm disorder, impacts over 37 million individuals worldwide[3]. In addition to atrial fibrillation, other types of cardiac arrhythmias include supraventricular tachycardias, bradyarrhythmias, ventricular tachycardia/ventricular fibrillation, and atrioventricular block[4,5]. The prevalence of cardiac arrhythmias increases with age, making age a significant risk factor for the development of these conditions. Specifically, the prevalence of AF rises from less than 0.5% in individuals under 40 years to more than 5% in those over 60 and approximately 10%-17% in those aged 80 and older[6]. Older adults are particularly vulnerable to arrhythmias due to age-related structural and electrical changes in the heart, including fibrosis, diminished pacemaker cell function, and slower conduction, all of which contribute to the increased incidence of arrhythmias in this population[7]. The presence of cardiac arrhythmias can significantly reduce quality of life due to the heightened risk of disability and cardiovascular complications, including sudden death[8]. Notably, about 80% of sudden cardiac deaths were linked to ventricular arrhythmias[2]. As the global population ages, the incidence of cardiac arrhythmias is expected to increase significantly, rising from 8.8 million in 2010 to an estimated 17.9 million by 2060[9]. This rise will place additional strain on healthcare systems and economic resources, driven by frequent emergency room visits for acute episodes, thus positioning cardiac arrhythmias as a major public health challenge[10].
Antiarrhythmic drug therapy (e.g., amiodarone, beta-blockers, and calcium channel blockers) remains one of the cornerstone management strategies for cardiac arrhythmias[11]. However, prolonged use of these medications is associated with toxic side effects. For example, prolonged amiodarone treatment for AF has off-target effects on the liver, lungs, and thyroid[12,13]. In addition to these adverse effects, antiarrhythmic drugs exhibit limited efficacy and high rates of recurrence[14]. The CABANA trial, the largest study to compare catheter ablation with drug therapy for AF, revealed that 36.4% and 18.4% of patients undergoing catheter ablation experienced AF recurrence within 12 months and developed symptomatic AF during follow-up, respectively[15]. These suboptimal outcomes highlight the urgent need to further explore alternative therapies to traditional antiarrhythmic drug treatments. Nanoparticle-based therapeutics offer a promising solution to mitigate the toxic effects of conventional pharmacotherapies, given their unique physicochemical properties that enhance bioavailability and enable targeted delivery[16]. The novel characteristics of nanoparticle-based treatments have spurred significant research into their application in cardiovascular diseases, particularly in cardiac arrhythmias [Figure 1][17].
Figure 1. Schematic overview of nanoparticle-based therapeutic strategies for cardiac arrhythmias. Figure created with Microsoft PowerPoint and BioRender. Created in BioRender. https://biorender.com/463e7en
This review aims to present preclinical studies on nanoparticle-based interventions for various cardiac arrhythmias, emphasizing their advantages and limitations [Tables 1 and 2]. Additionally, we include proof-of-concept studies on innovative drug and ablation therapies. Finally, we discuss future research directions and key challenges associated with the translation and clinical application of nanoparticle-based interventions for cardiac arrhythmias, with particular attention to their applicability in an aging population.
In-vitro studies on nanoparticle-based therapies for cardiac arrhythmia treatment
Nanoparticle | Drug (mechanism of action) | Disorder | In-vitro experiments | Advantages | Limitations | References |
Lipid nanocapsules (LNCs) | Amiodarone (potassium channel blocker) | Cardiac arrhythmias (Atrial Fibrillation) | Particle size | Mono-disperse | Dependence on acceptor phase interfaces | [30] |
Zeta potential | ||||||
Drug release kinetics | Submicron-size | |||||
Solubility | ||||||
Partitioning | Biocompatible excipients | |||||
Differential scanning calorimetry (DSC) | ||||||
TAT-conjugated polymeric liposomes | Lidocaine(sodium channel blocker) | Cardiac ventricular arrhythmias | Particle profiling | Facile uptake across cell membrane No considerable toxicity | Low solubility | [85] |
Short half-life | ||||||
Skin permeation | Leakage | |||||
Oxidation and hydrolysis of phospholipids | ||||||
Niosomes (with Gellan gum) | Dronedarone (potassium channel blocker) | Cardiac arrhythmias (Atrial Fibrillation) | Particle size | Nano- and narrow size | Aggregation | [38] |
Size distribution | High zeta potential | |||||
Zeta potential | High drug encapsulation | Sedimentation | ||||
Entrapment efficiency | Fusion | |||||
Drug release kinetics | Enhanced drug release | Drug leakage | ||||
DSC | ||||||
Niosomes | Diltiazem (calcium channel blocker) | Cardiac arrhythmias | Particle size | Controlled and prolonged drug release | Aggregation | [39] |
Entrapment efficiency | Sedimentation | |||||
Drug release kinetics | Fusion | |||||
DSC | Drug leakage | |||||
Niosomes (Bile salt-enriched; Bilosomes) | Carvedilol (beta blocker) | Cardiac arrhythmias | Particle size | Prevents instability, insolubility and rapid drug degradation | Aggregation | [41] |
Zeta potential | Fusion | |||||
Entrapment efficiency | ||||||
Drug release kinetics | Hydrolysis | |||||
Stability | ||||||
Exosomes (derived from adipose tissue-derived mesenchymal stem cells) | microRNA-320d | Atrial Fibrillation | MTT [3-(4,5-Dimethylthiazol-2-yl)-2,5-Diphenyltetrazolium Bromide] assay | Widely applied to deliver functional molecules, e.g., microRNAs | Difficulty in purification and drug loading | [43] |
TUNEL assay | ||||||
Exosomes (derived from bone marrow mesenchymal stem cells) | microRNA-148a | Atrial Fibrillation | Cell viability assay | Widely applied to deliver functional molecules, e.g., microRNAs | Difficulty in purification and drug loading | [44] |
Cell apoptosis assay | ||||||
TUNEL assay | ||||||
Solid lipid nanoparticles (composed of lecithin, Compritol, poloxamer & modified with PEG stearate and chitosan oligosaccharide lactate) | Nebivolol (beta blocker) | Hypertension-related cardiac arrhythmias | Drug release kinetics | Slower drug release in intestinal media | Relatively high water content | [92] |
Inadequate loading capacity | ||||||
Calcium carbonate-cells permeation ability | Drug leakage | |||||
Solid lipid nanoparticles | Carvedilol (beta blocker) | Cardiac arrhythmias | Calcium carbonate-cells permeation ability | Avoidance of the first-pass metabolism | Unwanted burst release (from particle growth and agglomeration) | [48] |
Higher drug bioavailability | ||||||
Absorption test | Prolonged drug release Protection from gastric acid | |||||
Chitosan-based polymeric nanoparticles (modified with cyclodextrin) | Amiodarone (potassium channel blocker) | Cardiac arrhythmias | Particle size | Slow and controlled drug release (long-term therapy) | High degradability rate | [64] |
Size distribution | ||||||
Zeta potential | ||||||
Entrapment efficiency | ||||||
Drug release kinetics | ||||||
DSC | ||||||
X-ray diffraction |
In-vivo studies on nanoparticle-based therapies for cardiac arrhythmia treatment
Nanoparticle | Drug (mechanism of action) | Mode of administration | Disorder | In-vivo experiments (animal model) | Advantages | Limitations | References |
Liposomes | Amiodarone (potassium channel blocker) | Intravenous | Ablation-related cardiac arrhythmias | Cardiac radiofrequency ablation rat model | Sustained, stable drug release Increased circulation time | Instability High production cost Unwanted oxidation and hydrolysis | [35] |
Liposomes | Amiodarone (potassium channel blocker) | Intravenous | Lethal cardiac arrhythmias | Ischemia-reperfusion (I/R) injury rat model | Reduced mortality | Instability | [34] |
High production cost | |||||||
Unwanted oxidation and hydrolysis | |||||||
Liposomes | Phenytoin (sodium channel blocker) | Intravenous | Cardiac arrhythmias | I/R injury rat model | Shorter drug distribution and elimination half-life | Instability | [88] |
High production cost | |||||||
Unwanted oxidation and hydrolysis | |||||||
TAT-conjugated polymeric liposomes | Lidocaine (sodium channel blocker) | Transdermal | Cardiac ventricular arrhythmias | Kunming mice | Facile uptake across the cell membrane | Low solubility | [85] |
Short half-life | |||||||
No considerable toxicity | Drug Leakage | ||||||
Unwanted oxidation and hydrolysis | |||||||
Bile salt-enriched niosomes (Bilosomes) | Carvedilol (beta blocker) | Oral | Cardiac arrhythmias | Pharmacokinetics on Wistar rats | Improved intestinal absorption | Aggregation | [41] |
Chylomicron-blocked rat model | Increased peak plasma concentration | Fusion | |||||
Hydrolysis | |||||||
Niosomes | Diltiazem (calcium channel blocker) | Nasal delivery | Cardiac arrhythmias | Pharmacokinetics on Wistar rats | Rapid delivery | Aggregation | [39] |
Sedimentation | |||||||
Avoidance of first-pass metabolism | Fusion | ||||||
Drug leakage | |||||||
Niosomes (with Gellan gum) | Dronedarone (potassium channel blocker) | Nasal delivery | Cardiac arrhythmias (Atrial Fibrillation) | Pharmacokinetics on male New Zealand white rabbits | Nano- and narrow size | Aggregation | [38] |
High zeta potential | Sedimentation | ||||||
High drug encapsulation | Fusion | ||||||
Enhanced drug release | Drug leakage | ||||||
Solid lipid nanoparticles (composed of lecithin, Compritol, poloxamer and modified with Polyethylene glycol (PEG) stearate and chitosan oligosaccharide lactate) | Nebivolol (beta blocker) | Oral | Hypertension-related cardiac arrhythmias | Pharmacokinetics on Wistar rats | Slower drug release in intestinal media | Relatively high water content | [92] |
Inadequate loading capacity | |||||||
Drug leakage | |||||||
Solid lipid nanoparticles | Carvedilol (beta blocker) | Intranasal | Cardiac arrhythmias | Pharmacokinetics on rabbits | Mucous membrane adhesion | Unwanted burst release (from particle growth and agglomeration) | [49] |
Permeation of large quantities of drug to nasal mucosa | |||||||
Cyclodextrin nanoparticles | Amiodarone (potassium channel blocker) | Intravenous | Atrial fibrillation | MerTKGFP/+ reporter mouse | Decreased effect on off-target tissues (e.g., lung, liver, and thyroid) | No evidence of anti-Afib activity (only AVN-specific targeting) | [55] |
Tannic acid and cyclodextrin-derived polyphenol nanoparticles (TPTN) | TPTN (ROS scavenging ability) | Intravenous injection | Ventricular fibrillation | Male Sprague-Dawley rats | Protection from hypoxic-ischemic injury | Long-term effects and toxicological profile not evaluated | [90] |
Good safety profile on i. v. route | |||||||
Polymeric nanoparticles | Amiodarone (potassium channel blocker) | Oral | Atrial Fibrillation | Male Sprague-Dawley rats | Controlled release | Limited biodistribution and toxicological profile | [61] |
Slow biodegradation | |||||||
Low energy fabrication | |||||||
Magnetic nanoparticles | N-isopropyl acrylamide monomer (NIPA-M) | Circumflex coronary artery infusion | Atrial fibrillation | Mongrel dogs | Targeted and controlled therapy | Mobilization kinetics to epicardium not measured | [74] |
Minimal systemic side-effects | In vivo payload release kinetics not measured | ||||||
Magnetic nanoparticles | CaCl2 payload (suppression of ganglionic plexi) | Microinjection into ganglionic plexi | Atrial fibrillation | Mongrel dogs | Endogenous components | Minimal targeting time not measured | [76] |
Performed during cardiac catheterization | |||||||
N-isopropyl acrylamide-coated Magnetic Nanoparticles (NIPA-co-MN) | N-isopropyl acrylamide | Intracardiac injection | Atrial fibrillation | Beagle dogs | Minimally-invasive and low-cost therapy | Small sample size | [75] |
Stimulus factors not considered | |||||||
Short medication time | |||||||
Magnetic nanoparticles (with L-glutamate) | CaCl2 payload (suppression of ganglionic plexi) | Microinjection into ganglionic plexi | Postoperative atrial fibrillation | Mongrel dogs | Short burst and sustained drug release | Long-term effects not evaluated | [66] |
Target site concentration and stabilization | |||||||
Connexin 43 (Cx43)-loaded magnetic nanoparticles | Cx43-expressing cardiac fibroblasts (cellular cardiomyoplasty) | Intramyocardial transplantation | Post-infarct ventricular tachycardia | CD-1® wild-type mice | Significant reduction of postinfraction arrhythmias | No improvement of cardiac pump function | [83] |
PEG-coated gold nanorods (PEG-AuNRs) | PEG-AuNRs and near-infrared (NIR) light (left stellate ganglion inhibition) | Intraganglionic injection and irradiation | Acute ischemia-induced ventricular arrhythmias | Canines | Ameliorates acute ischemia-induced VAs | High production cost | [82] |
Off-target organ toxicity | |||||||
Near-infrared sensitive (NIRS) gold nanoshells (GNS) | NIRS GNS (cardiac ablation) | Photothermal ablation | Medically refractory ventricular tachycardia | Porcine cardiac tissue | NIR light can penetrate tissue depths > 1 cm | Wavelength close to maximal absorption of hemoglobin | [89] |
Biocompatibility and oxidation resistant | |||||||
Botulinum toxin-chitosan nanoparticles | Botulinum toxin (autonomic block) | Subepicardial injection | Atrial fibrillation | Wistar rats | Prolongs botulinum effect | High degradability rate | [80] |
Decreases toxicity | |||||||
Tetanus toxin C (TTC)-conjugated nanoparticles | TTC and N-isopropylacrylamide (left cardiac sympathetic denervation) | Intravenous injection | Ventricular arrhythmia | Canines | Improved cardiac function | Biodistribution, long-term effects and toxicological profile not evaluated | [81] |
Reduced ventricular arrhythmia inducibility | |||||||
Nanoparticle-encapsulated superoxide dismutase (NP-SOD) | Superoxide dismutase (antioxidant) | Intramyocardial injection | Myocardial ischemia-reperfusion injury | Wistar rats | Improves retention | Biodistribution, long-term effects and toxicological profile not evaluated | [84] |
Protects against I/R injury | Electrophysiological effects not assessed | ||||||
Attenuates ventricular remodeling |
NANOPARTICLE-BASED TREATMENTS FOR ATRIAL FIBRILLATION
AF is the most common cardiac arrhythmia, accounting for nearly one-third of rhythm-related hospitalizations[18]. It significantly increases the risk of stroke, heart failure, and sudden cardiac death[19]. Management typically involves antiarrhythmic drugs or catheter ablation[20].
Nanoparticle-based antiarrhythmic drug therapy for atrial fibrillation
Amiodarone, a Class III antiarrhythmic, is the most effective and widely recommended drug for managing atrial fibrillation (AF), particularly in patients with heart failure[21-23]. However, its use is limited by significant side effects (i.e., pulmonary, hepatic, and thyroid toxicity) due to poor solubility, long half-life, and nonspecific tissue accumulation, as reported from clinical experience and real-world data. To address these challenges, novel drug delivery systems [Figure 1] are being developed to improve its safety and therapeutic efficacy[24-26].
(Top Panel) Nanoparticle-mediated drug delivery platforms - including lipid nanocapsules, liposomes, niosomes, exosomes, solid lipid nanoparticles, cyclic nanoparticles, and polymeric nanoparticles - facilitate the targeted and controlled release of antiarrhythmic agents such as amiodarone, enhancing therapeutic efficacy while minimizing systemic toxicity. (Middle Panel) Magnetic nanoparticle-based ablation strategies utilize externally applied magnetic fields to guide particles to sympathetic ganglia (e.g., left stellate ganglion), where localized neuromodulation or photothermal ablation may be achieved. (Bottom Panel) Non-magnetic nanoparticle-based ablation involves the direct injection of bioactive particles (e.g., those carrying botulinum or tetanus toxin) to modulate autonomic input to the heart.
Lipid nanocapsules
One of the earliest novel delivery systems for amiodarone in preclinical studies was the lipid nanocapsules (LNCs). LNCs are nanoscale carriers, typically ranging from 20 to 100 nm in diameter, composed of three primary components: (1) a nonionic surfactant forming the capsule membrane; (2) an aqueous phase of sodium chloride solution; and (3) an oily core consisting of medium-chain triglycerides, such as capric and caprylic acid derivatives[27-29]. In preclinical formulations, amiodarone was successfully encapsulated within LNCs utilizing polyethylene glycol-660 hydroxystearate as the surfactant, with soybean lecithin added to enhance capsule stability[30].
These amiodarone-loaded LNCs demonstrated a low polydispersity index and pH-sensitive drug release, thereby addressing the significant burst release commonly observed in earlier nanoparticle systems designed for low molecular weight compounds[31,32]. The use of biocompatible excipients and the physicochemical properties of the formulation suggest potential suitability for intravenous or intraperitoneal administration[30]. However, these findings are currently limited to preclinical evaluations and require further investigation in clinical settings to confirm their safety and therapeutic efficacy in humans.
Liposomes
Liposomes have also been explored in preclinical studies as a novel delivery system for amiodarone. These spherical vesicles, ranging in size from approximately 30 nm to several micrometers, are composed of one or more phospholipid bilayers formed from natural, non-toxic phospholipids and cholesterol[33]. The lipophilic character of the bilayer facilitates the encapsulation and solubilization of amphiphilic and lipophilic drugs, such as amiodarone. Additionally, liposomes are generally considered non-immunogenic following both systemic and non-systemic administration[16,33]. In animal models, amiodarone-loaded liposomes have demonstrated improved therapeutic profiles compared to free amiodarone. In a rat model of ischemia/reperfusion (I/R) injury, intravenous administration of liposomal amiodarone at 3 mg/kg in Wistar rats significantly reduced the duration of ventricular tachycardia and ventricular fibrillation (VT/VF), as well as mortality from these arrhythmias, compared to a higher dose (10 mg/kg) of free amiodarone[34]. Liposomal amiodarone was also associated with a reduced incidence of bradycardia and hypotension in the same I/R model[34]. Furthermore, in Sprague-Dawley rats subjected to cardiac radiofrequency (RF) ablation, liposomal amiodarone exhibited more sustained and stable drug release compared to its free form[35]. These properties contributed to enhanced antiarrhythmic efficacy at lower doses, potentially reducing dose-dependent side effects such as bradycardia and hypotension.
Biodistribution studies further revealed that liposomal amiodarone preferentially accumulates in the myocardium of rats following cardiac I/R injury and RF ablation, likely due to increased vascular permeability in inflamed cardiac tissues[34,35]. This targeted accumulation underscores the potential utility of liposomal amiodarone in the treatment of arrhythmias associated with ischemic heart disease or post-catheter ablation interventions. However, despite reduced drug concentrations in the lungs and kidneys, potentially minimizing pulmonary and renal toxicity, liposomal delivery was associated with increased amiodarone accumulation in the liver and spleen. This effect is likely due to passive uptake by these macrophage-rich organs (i.e., organs with a high concentration of macrophages), raising concerns about potential hepatic and splenic toxicity with long-term use[35].
Collectively, these findings from preclinical studies suggest that liposomal delivery of amiodarone holds promise for targeted cardiac therapy, but further investigation is required to evaluate its safety and efficacy in clinical settings.
Niosomes
Niosomes have emerged in preclinical research as a promising alternative to liposomes for drug delivery. Structurally like liposomes, niosomes are spherical vesicles comprising at least one bilayer but are formed using nonionic surfactants rather than phospholipids. This substitution imparts greater stability, resulting in improved shelf life and resistance to photodegradation compared to liposomal formulations[36]. Moreover, the simpler preparation methods make niosomes more cost-effective for large-scale production[37].
In preclinical studies, niosomes have been employed to enhance the bioavailability of antiarrhythmic agents. Dronedarone - a non-iodinated benzofuran analog of amiodarone - was successfully encapsulated in niosomes composed of Span® 80 and cholesterol (1:1), with Gellan gum used as a mucoadhesive agent (i.e., able to stick to mucosal surfaces). When administered intranasally to male New Zealand rabbits, this formulation resulted in significantly increased maximum plasma concentration (C_max), the area under the concentration-time curve from 0 to 72 h (AUC0-72), and total drug exposure (AUC0-∞) compared to an orally administered dronedarone suspension[38].
Similarly, in a preclinical model involving male Wistar rats, the calcium channel blocker diltiazem - another drug used in atrial fibrillation management - was encapsulated in niosomes formulated with Span® 60 and cholesterol (1:1). Intranasal administration of this formulation led to enhanced pharmacokinetic parameters, including increased mean residence time (MRT), elimination half-life (t1/2), and AUC, along with a reduced elimination rate constant (K_e), compared to an aqueous diltiazem solution in phosphate-buffered saline[39].
Niosomes have also shown potential for improving the oral bioavailability of drugs. Although the precise mechanisms remain under investigation, previous studies have demonstrated that incorporating bile salts into vesicular systems can increase their resistance to bile salt-induced disruption in the gastrointestinal (GI) tract[40]. This approach has been applied in a preclinical context to enhance the oral delivery of carvedilol, a third-generation β-blocker used in the prevention and treatment of atrial fibrillation. In male Wistar rats, oral administration of carvedilol-loaded niosomes with bile salts significantly increased intestinal absorption and systemic bioavailability - as indicated by higher AUC0-∞ - compared to carvedilol suspended in 0.5% sodium carboxymethyl cellulose[41].
These preclinical findings underscore the versatility and promise of niosomal drug delivery systems for improving the pharmacokinetics of antiarrhythmic agents. However, further studies, including clinical trials, are necessary to validate their translational potential.
Exosomes
Exosomes are another class of nanoscale vesicles currently being investigated in preclinical studies for drug delivery applications. These endogenous, spherical vesicles range from approximately 40 to 200 nm in diameter and can transport a diverse array of signaling biomolecules, including proteins, nucleic acids, and non-coding RNAs such as microRNAs (miR)[42].
In preclinical experiments, exosomes derived from adipose tissue-derived mesenchymal stem cells (AMSCs) and loaded with miR-320d demonstrated anti-apoptotic effects when applied to isolated cardiomyocytes obtained from a murine model of AF[43]. Similarly, bone marrow mesenchymal stem cell (BMSC)-derived exosomes loaded with miR-148a showed protective effects in HL-1 mouse cardiomyocyte cells subjected to electrical stimulation to induce an AF-like phenotype. Treatment with these engineered exosomes significantly inhibited apoptosis in the stimulated cardiomyocytes[44].
While these findings highlight the potential of exosome-based therapies for AF, it is important to note that all current evidence is limited to preclinical models. Significant challenges remain before clinical translation can be realized, including the development of reproducible, high-purity isolation techniques and efficient, scalable methods for exosome loading[45].
Solid lipid nanoparticles
In contrast to the aqueous or semi-aqueous core vesicles discussed so far, solid lipid nanoparticles (SLNs) represent a distinct class of drug delivery systems characterized by a solid lipid core surrounded by a monolayer of surfactant[46]. This rigid lipid matrix enhances the encapsulation efficiency and stability of hydrophobic drugs relative to liposomes and polymeric nanoparticles[47].
Carvedilol, a third-generation β-blocker commonly used in the management of atrial fibrillation, exhibits poor oral bioavailability (~ 25%) due to extensive first-pass hepatic metabolism[48]. In preclinical studies, encapsulating carvedilol in SLNs significantly improved its cellular uptake. Specifically, SLN-encapsulated carvedilol demonstrated superior absorption in Caco-2 cells (a human colorectal adenocarcinoma cell line commonly used to model intestinal epithelium) compared to carvedilol physically mixed with SLNs or carvedilol alone, suggesting the potential for overcoming its limited oral bioavailability[48].
Additional preclinical investigations have evaluated the intranasal delivery of carvedilol-loaded SLNs. In an ex vivo study using sheep nasal mucosa, SLN formulations achieved enhanced mucosal penetration compared to carvedilol solution alone. Furthermore, in vivo administration of carvedilol-loaded SLNs incorporated into an in situ gelling system resulted in significantly higher absolute bioavailability following intranasal delivery in rabbits compared to conventional oral administration[49].
These preclinical findings underscore the potential of SLNs as a promising platform for improving the bioavailability of poorly absorbed cardiovascular drugs. However, further studies are needed to optimize formulation parameters and assess safety and efficacy in clinical contexts.
Cyclic nanoparticles
Cyclodextrins are cyclic oligosaccharide-based nanoparticles composed of α-1,4-linked α-D-glucopyranose units[50]. These molecules possess a hydrophobic, bucket-shaped cavity capable of forming supramolecular host-guest inclusion complexes with lipophilic compounds, such as amiodarone[51]. In drug delivery applications, cyclodextrins offer several advantages, including enhanced solubility of hydrophobic drugs, improved encapsulation efficiency, protection from chemical degradation, and superior drug stability compared to liposomal systems[52].
Recent preclinical studies have explored the use of cyclodextrin nanoparticles (CDNPs) for targeted antiarrhythmic drug delivery. Macrophages, which exhibit high phagocytic activity toward CDNPs, are abundant in the atrioventricular nodal (AVN) region of the heart, making this cell population an attractive target for localized drug delivery within the cardiac conduction system[53,54]. In a murine model, intravenous administration of amiodarone-loaded CDNPs in C57BL/6 mice resulted in selective accumulation of nanoparticles in the myocardium, particularly within the AVN, 24 h post-injection[55]. Additional accumulation was observed in macrophage-rich organs such as the liver and spleen, whereas a relatively lower distribution was detected in the lungs and skeletal muscle[55]. Correspondingly, tissue analysis confirmed elevated amiodarone concentrations in the heart, liver, and spleen, suggesting the potential for reduced pulmonary toxicity but raising the possibility of hepatic and splenic adverse effects.
Although these findings underscore the potential of CDNPs for site-specific delivery of amiodarone and reduction of off-target effects, it is important to note that the current evidence remains limited to preclinical models. Demonstration of therapeutic efficacy - specifically, arrhythmia prevention or suppression such as in atrial fibrillation - has yet to be established. Rigorous evaluation in disease-relevant models and eventual clinical studies will be essential to determine the translational applicability of this approach.
Polymeric nanoparticles
Polymeric nanoparticles represent a versatile class of nanocarriers for drug delivery, typically classified as nanocapsules - comprising a polymeric shell surrounding an oily core - or nanospheres, in which the drug is uniformly dispersed within a polymeric matrix[56]. These systems can either adsorb therapeutic agents onto their surface or encapsulate them within the core or matrix, offering several pharmacokinetic advantages, including enhanced bioavailability, sustained release, and protection from premature degradation[57,58].
Polymeric nanoparticles may be synthesized from either synthetic or natural biodegradable polymers. Synthetic polymers can be chemically modified to tailor drug release profiles, improve targeting specificity, and reduce systemic toxicity[59,60]. Among these, poly(lactic-co-glycolic acid) (PLGA) is a well-characterized biodegradable polymer with demonstrated biocompatibility and controlled degradation properties. In preclinical studies, oral administration of PLGA-encapsulated amiodarone in Sprague-Dawley rats resulted in significantly reduced drug-related toxicities. Animals receiving the PLGA formulation exhibited lower serum levels of hepatic enzymes (alanine aminotransferase and aspartate aminotransferase), attenuated pulmonary capillary congestion and inflammatory infiltration, and preserved thyroid function (normal T4 and thyroid stimulating hormone levels) compared to those administered with free amiodarone[61].
Natural polymers offer distinct advantages due to their inherent biocompatibility, low immunogenicity, and additional properties such as antimicrobial activity[62]. Chitosan, a cationic natural polymer, has garnered significant attention for drug delivery applications due to its mucoadhesive properties, capacity to enhance epithelial permeability, and affinity for negatively charged cellular membranes[63]. In a preclinical formulation, amiodarone was first modified with cyclodextrin to enhance its solubility and then encapsulated into chitosan-based nanoparticles. This system demonstrated sustained release of amiodarone over 14 days, suggesting strong potential for use in controlled-release antiarrhythmic therapy[64].
Although these findings are currently limited to preclinical models, they collectively underscore the potential of polymeric nanoparticle systems to improve the safety and efficacy of amiodarone and other antiarrhythmic drugs. Further investigation is needed to evaluate their clinical translatability and therapeutic benefit in human subjects.
Nanoparticle-based ablative treatment for atrial fibrillation
Refractory AF is often treated with radiofrequency (RF) catheter ablation via circumferential pulmonary vein isolation (CPVI), but its non-selective nature can cause collateral tissue damage and new arrhythmogenic substrates, limiting long-term success rates to below 50% at five years[65,66]. Targeted ganglionated plexi (GP) ablation within the cardiac autonomic nervous system has emerged as a more precise alternative, modulating autonomic input while minimizing myocardial injury. Building on this, nanoparticle-based systems are being explored for precise, non-destructive neuromodulation of autonomic pathways[66-70] [Figure 1]. Although still in preclinical stages, early studies suggest these platforms could offer reversible, site-specific alternatives to conventional ablation for AF treatment.
Magnetic nanoparticles
Magnetic nanoparticles (MNPs) have garnered significant interest in preclinical research for their capacity to enable the targeted delivery of therapeutic agents under the guidance of an external magnetic field[71]. While MNP-based strategies have been explored in a variety of disease models, including oncology and neurodegenerative disorders, their application in the context of GP ablation for AF remains entirely preclinical at this stage[72,73].
One of the earliest MNP-based formulations for GP modulation featured a magnetite core surrounded by a thermoresponsive hydrogel matrix capable of delivering a neurotoxic payload[74]. In a preclinical canine study, MNPs loaded with the neurotoxin N-isopropyl acrylamide monomer (NIPA-M) were infused into the circumflex coronary artery of adult mongrel dogs. Within two hours, electrophysiological measurements indicated functional suppression of the inferior right GP (IRGP), with histological analysis confirming the accumulation of the nanoparticles within the IRGP[74]. In addition to serving as a neurotoxic payload, N-isopropyl acrylamide (NIPA) can be integrated into the hydrogel matrix itself to exploit its thermoresponsive properties. In another preclinical study, MNPs coated with NIPA were administered via the circumflex coronary artery in beagle dogs and similarly suppressed IRGP activity[75].
A distinct MNP formulation employed a PLGA matrix shell with a magnetite core and calcium chloride as the active agent[76]. Although calcium is a physiological ion, excessive intracellular calcium in neurons can induce neuroapoptosis, thereby achieving a form of chemical GP ablation[77]. In a preclinical canine model, calcium chloride-loaded MNPs were infused into the left circumflex artery (LCX) under an externally applied magnetic gradient, leading to marked suppression of both IRGP and superior left GP (SLGP) activity[76].
To further enhance neurotoxicity, L-glutamate was added to a similar MNP formulation to potentiate calcium influx through NMDA receptor activation. This enhanced formulation was tested in a preclinical model of postoperative atrial fibrillation (POAF), created via sequential survival thoracotomies in mongrel dogs[66]. Targeted injection of the MNPs into the left atrial GPs suppressed AF inducibility and reversed POAF-associated shortening of the atrial effective refractory period. Notably, this treatment preserved circadian heart rate variability and exercise-induced chronotropic response, suggesting modulation rather than complete ablation of cardiac autonomic function[66].
These findings underscore the preclinical promise of MNP-based strategies for selective neuromodulation of cardiac autonomic inputs in AF. However, translation to clinical application will require substantial validation, including long-term safety profiling, precise magnetic targeting techniques, and robust demonstration of efficacy in larger and more heterogeneous animal models.
Non-magnetic nanoparticles
Non-magnetic nanoparticle formulations have also been investigated in preclinical studies as a potential strategy for GP ablation. Botulinum toxin has previously demonstrated efficacy in suppressing AF inducibility and delaying its progression when directly injected into atrial GPs, although these findings remain limited to animal models[78,79]. Building on this concept, a preclinical study recently evaluated a chitosan-based nanoparticle formulation encapsulating botulinum toxin for neuromodulation. In this study, epicardial administration of the botulinum toxin-loaded chitosan nanoparticles significantly reduced the incidence of cardiac arrhythmias - specifically ventricular fibrillation - in rat models[80].
While these findings are promising, particularly about localized and sustained neurotoxin delivery, further preclinical investigations using validated animal models of AF are essential to determine whether this formulation can effectively modulate atrial GPs to treat or prevent atrial fibrillation specifically. Translation to clinical application will also require a comprehensive evaluation of safety, specificity of GP targeting, and long-term outcomes.
NANOPARTICLE-BASED TREATMENTS FOR OTHER CARDIAC ARRHYTHMIAS
Ventricular arrhythmias
Current research into nanoparticle-based therapies for ventricular arrhythmias (VAs) is primarily focused on the prevention of post-infarction VA and the preservation or improvement of ventricular function, all within preclinical settings. One strategy to prevent post-infarction VA involves left cardiac sympathetic denervation (LCSD), which has been shown to modulate arrhythmic risk. In a preclinical canine model of heart failure, LCSD combined with tetanus toxin C (TTC)-conjugated nanoparticles significantly improved cardiac function and reduced the inducibility of VAs[81]. Similarly, in an acute ischemia-induced canine model, polyethylene glycol-coated gold nanorods (PEG-AuNRs) were used in combination with near-infrared (NIR) light to achieve photothermal inhibition of left stellate ganglion (LSG) activity. This preclinical intervention resulted in a marked decrease in VA occurrence[82].
Other preclinical approaches involve reparative strategies following myocardial infarction. For instance, connexin 43 (Cx43)-loaded magnetic nanoparticles have been used in murine models of cellular cardiomyoplasty to enhance intercellular coupling and reduce post-infarction arrhythmias[83]. In addition, superoxide dismutase (SOD), a potent antioxidant with poor myocardial retention, has been encapsulated into nanoparticles (NP-SOD) for preclinical evaluation in rat models. Intramyocardial administration of NP-SOD before reperfusion was found to reduce reperfusion injury and prevent adverse ventricular remodeling, suggesting a protective role against ischemia-reperfusion injury[84].
In contrast, some nanoparticle formulations for VA remain at an even earlier in vitro stage of investigation. Lidocaine, a class IB antiarrhythmic agent, was previously incorporated into polymeric liposomes conjugated with a transcriptional transactivator peptide (TAT) to facilitate transdermal delivery[85]. However, this formulation has only been evaluated in preclinical in vitro studies and, to date, has not been tested in any animal model of cardiac arrhythmia.
Ventricular tachycardia
VT can be pharmacologically managed using class IB antiarrhythmic agents such as phenytoin[86]. Phenytoin acts by reducing calcium-dependent depolarization and prolonging the cardiac action potential refractory period, thereby inhibiting early afterdepolarizations (EADs) and suppressing ventricular tachyarrhythmias[87]. In a preclinical study using a rat model of myocardial ischemia/reperfusion (I/R) injury, administration of liposome-encapsulated phenytoin significantly improved left ventricular function and attenuated adverse post-infarction remodeling - evidenced by reduced infarct size and decreased fibrotic area - compared to treatment with empty liposomes[88]. These beneficial effects were partially attributed to the preferential uptake of liposome-encapsulated phenytoin by CD43+inflammatory monocytes, which play a critical role in post-injury inflammation[88]. While these results highlight the potential of nanoparticle-mediated phenytoin delivery in post-infarction cardiac repair, the specific antiarrhythmic efficacy of this formulation remains to be demonstrated in preclinical animal models of cardiac arrhythmia.
Nanoparticle-based systems are also being explored to enhance the precision and efficacy of ablative therapies for VT. Radiofrequency (RF) ablation remains the clinical standard for treating medically refractory VT; however, it is limited by shallow lesion depth and the need for consistent tissue contact. To address these limitations, near-infrared-sensitive (NIRS) nanoparticles have been developed to facilitate site-specific, photothermal ablation through externally applied NIR light. In a preclinical ex vivo porcine model, NIRS nanoparticle-mediated ablation was shown to create deeper, transmural lesions compared to RF, offering the potential for improved therapeutic outcomes and reduced procedural complications[89]. This approach also enables lesion formation using conventional catheters equipped with fiber optic guidance, bypassing the need for continuous tissue contact inherent to RF systems. While promising, these findings remain at the preclinical stage, and further in vivo studies are needed to evaluate the safety, specificity, and functional outcomes of NIRS-based VT ablation.
Ventricular fibrillation
Polyphenol-functionalized nanoparticles (NPs) have emerged as a promising platform for targeted therapy in cardiovascular diseases due to their high affinity for various cells and tissues. Among these, tannic acid (TA)-derived functional nanoparticles demonstrate notable accumulation in injured cardiac tissue following intravenous administration. By integrating TA with a bioactive cyclodextrin-based material (TPCD), a nanoparticle formulation known as TPTN was developed, characterized by favorable physicochemical properties and robust reactive oxygen species (ROS)-scavenging activity. In a preclinical study using a murine model of VF, intravenous administration of TPTN significantly improved survival rates, particularly when used in conjunction with therapeutic hypothermia, a standard adjunctive therapy following cardiac arrest[90]. TPTN was found to localize effectively to the site of myocardial injury in resuscitated rats and contributed to improvements in myocardial function, reduction of oxidative stress, and attenuation of cardiac tissue damage - all under preclinical conditions[90].
In another preclinical development, nebivolol - a third-generation β-blocker known to reduce the incidence of ventricular arrhythmias by increasing the ventricular fibrillation threshold[91] - was incorporated into SLNs to improve its pharmacokinetic profile. These SLNs were surface-modified with chitosan oligosaccharide lactate to enhance permeability across Caco-2 cell monolayers and with polyethylene glycol (PEG) to increase systemic bioavailability[92]. In a rat model, oral administration of PEG-modified, nebivolol-loaded SLNs resulted in a higher peak plasma concentration compared to conventional nebivolol formulations[92]. However, it is important to note that while these delivery systems enhanced drug absorption in preclinical pharmacokinetic studies, they have not yet been tested in any animal models of cardiac arrhythmia, and their antiarrhythmic potential remains to be established.
LIMITATIONS AND CHALLENGES OF NANOPARTICLE-BASED TREATMENTS FOR CARDIAC ARRHYTHMIAS
Nanoparticle-based therapeutics represent a novel and promising approach to the treatment of cardiac arrhythmias. However, as presented in the preceding sections, nearly all investigations into their application remain in the preclinical stage, encompassing in vitro experiments and animal models. While early findings are encouraging, several critical challenges must be addressed before these novel therapeutics can advance to clinical translation.
One of the primary limitations is the incomplete understanding of cardiovascular toxicity associated with nanoparticle-based therapies, particularly concerning their impact on the cardiac conduction system[93]. Although limited in number, existing preclinical studies examining the cardiovascular toxicities of various nanomaterials suggest that they may induce pro-thrombotic states, inflammation, apoptosis, and oxidative stress in cardiac tissues[93,94]. Among these, inflammation and oxidative stress are believed to be the predominant contributors to nanoparticle-induced cardiovascular damage, which could potentially precipitate hypertension, myocardial infarction, or myocarditis[93,95]. Furthermore, the off-target accumulation of nanoparticles, particularly in the liver and spleen, raises concerns regarding long-term toxicity with repeated or chronic use. Comprehensive safety evaluations - including dose-response, biodistribution, and toxicity studies - are therefore essential before human application.
Another major area of focus is the enhancement of targeting precision, particularly for MNP-mediated ablation techniques. Improved control over lesion localization is essential to avoid off-target effects that could result in inadvertent damage to critical cardiac structures such as valvular tissue, coronary vasculature, or components of the conduction system, all of which could lead to serious complications. In addition, the long-term biocompatibility of MNPs must be carefully investigated, with particular attention to the potential for iron accumulation from the magnetite core and its implications for myocardial and systemic health.
Beyond biological safety, another major hurdle is the manufacturing challenges associated with nanoparticle-based formulations. To be viable for clinical use, nanoparticles must be produced at a large scale with high throughput, reproducibility, and batch-to-batch consistency. Currently, many nanoparticle synthesis protocols yield small quantities of products with variable chemical composition, inconsistent physical properties, and limited stability[93]. For instance, the scalable production of exosomes remains hampered by the lack of robust high-purity isolation and purification techniques[45]. In contrast, synthetic vesicles such as niosomes offer a more straightforward and cost-effective production process compared to liposomes and represent a step in the right direction toward scalable manufacturing[37].
Another underexplored area is the application of nanoparticle-based therapeutics in aging populations. Although no studies have directly evaluated nanoparticle toxicity in the aging heart, age-related physiological changes are known to alter the pharmacokinetics of drugs and may similarly affect nanoparticles[96]. Aging is associated with reduced mesenteric blood flow, which may impair oral drug and nanoparticle absorption[97]. Alterations in body composition - specifically increased fat mass and decreased total body water - can affect the distribution of lipophilic and hydrophilic nanoparticles[98]. Furthermore, age-related declines in hepatic and renal clearance may lead to increased bioavailability of nanoparticles, necessitating careful dose adjustments in older populations[99]. These factors underscore the importance of age-specific design and dosing strategies for nanoparticle-based therapies targeting older adults with cardiac arrhythmias.
Collectively, these scientific and technical barriers must be addressed to enable the safe and effective clinical translation of nanoparticle-based antiarrhythmic therapies. Future research should focus on comprehensive toxicological evaluation, refinement of large-scale synthesis techniques, and standardization of characterization and quality control protocols. These efforts will be instrumental in ensuring the safety, efficacy, and regulatory compliance necessary for clinical translation.
CONCLUSION
Nanoparticle-based therapies are emerging as innovative tools to advance the treatment landscape of cardiac arrhythmias, offering the potential to improve efficacy while minimizing the side effects of conventional pharmacologic and interventional approaches. However, their clinical translation hinges on overcoming several key challenges through ongoing preclinical and translational efforts.
A critical priority is enhancing targeting precision, particularly for MNP-mediated ablation. Precise lesion localization is essential to avoid collateral damage to vital cardiac structures. Additionally, the long-term biocompatibility of MNPs must be evaluated, including risks of iron accumulation from magnetite cores and associated systemic effects. For other platforms, such as liposomal, polymeric, and cyclic nanoparticles, improving targeting efficiency is equally important. Reducing off-target accumulation, especially in the liver and spleen, remains a major obstacle. Enhancing nanoparticle delivery to arrhythmogenic sites while sparing healthy tissue will be key to improving safety and therapeutic efficacy.
Future studies using large animal models will help test nanoparticle-based treatments for cardiac arrhythmias. Large animal studies, including canines (atrial fibrillation, ventricular arrhythmias), swine (ventricular tachycardia, atrial fibrillation), ovine (chronic AF), and non-human primates, offer more clinically relevant physiology than rodent models and are essential for assessing dosing, safety, and efficacy before human trials.
Finally, scalable and reproducible manufacturing remains a significant barrier. Clinical and commercial viability will depend on the ability to produce nanoparticles with consistent physicochemical properties, stability, and batch-to-batch uniformity at an industrial scale.
DECLARATIONS
Acknowledgment
Figures were made using the licensed version of BioRender.com.
Authors’ contributions
Conceived and designed research, synthesized and interpreted data, drafted, edited, revised and approved the final manuscript: Dela Rosa JGL, Bantayan NRN, Tantengco OAG
Availability of data and materials
Not applicable.
Financial support and sponsorship
None.
Conflicts of interest
Not applicable.
Ethical approval and consent to participate
Not applicable.
Consent for publication
Not applicable.
Copyright
© The Author(s) 2025.
REFERENCES
1. Antzelevitch C, Burashnikov A. Overview of basic mechanisms of cardiac arrhythmia. Card Electrophysiol Clin. 2011;3:23-45.
2. Rahman F, Kwan GF, Benjamin EJ. Global epidemiology of atrial fibrillation. Nat Rev Cardiol. 2014;11:639-54.
3. Lippi G, Sanchis-Gomar F, Cervellin G. Global epidemiology of atrial fibrillation: an increasing epidemic and public health challenge. Int J Stroke. 2021;16:217-21.
4. Yuyun MF, Bonny A, Ng GA, et al. A systematic review of the spectrum of cardiac arrhythmias in sub-saharan africa. Glob Heart. 2020;15:37.
5. Dai H, Zhang Q, Much AA, et al. Global, regional, and national prevalence, incidence, mortality, and risk factors for atrial fibrillation, 1990-2017: results from the global burden of disease study 2017. Eur Heart J Qual Care Clin Outcomes. 2021;7:574-82.
6. Chen Q, Yi Z, Cheng J. Atrial fibrillation in aging population. Aging Med (Milton). 2018;1:67-74.
7. Tellez JO, Mczewski M, Yanni J, et al. Ageing-dependent remodelling of ion channel and Ca2+ clock genes underlying sino-atrial node pacemaking. Exp Physiol. 2011;96:1163-78.
8. Murakoshi N, Aonuma K. Epidemiology of arrhythmias and sudden cardiac death in Asia. Circ J. 2013;77:2419-31.
9. Krijthe BP, Kunst A, Benjamin EJ, et al. Projections on the number of individuals with atrial fibrillation in the European Union, from 2000 to 2060. Eur Heart J. 2013;34:2746-51.
10. Murphy A, Banerjee A, Breithardt G, et al. The world heart federation roadmap for nonvalvular atrial fibrillation. Glob Heart. 2017;12:273-84.
11. Wolfes J, Ellermann C, Frommeyer G, Eckardt L. Evidence-based treatment of atrial fibrillation around the globe: comparison of the latest ESC, AHA/ACC/HRS, and CCS guidelines on the management of atrial fibrillation. Rev Cardiovasc Med. 2022;23:56.
12. Singh BN, Nademanee K. Amiodarone and thyroid function: clinical implications during antiarrhythmic therapy. Am Heart J. 1983;106:857-69.
13. Herendael H, Dorian P. Amiodarone for the treatment and prevention of ventricular fibrillation and ventricular tachycardia. Vasc Health Risk Manag. 2010;6:465-72.
14. Wang Z, Tong Q, Li T, Qian Y. Nano drugs delivery system: a novel promise for the treatment of atrial fibrillation. Front Cardiovasc Med. 2022;9:906350.
15. Poole JE, Bahnson TD, Monahan KH, et al; CABANA Investigators and ECG Rhythm Core Lab. Recurrence of atrial fibrillation after catheter ablation or antiarrhythmic drug therapy in the CABANA trial. J Am Coll Cardiol. 2020;75:3105-18.
16. Nadimi AE, Ebrahimipour SY, Afshar EG, et al. Nano-scale drug delivery systems for antiarrhythmic agents. Eur J Med Chem. 2018;157:1153-63.
17. Smith BR, Edelman ER. Nanomedicines for cardiovascular disease. Nat Cardiovasc Res. 2023;2:351-67.
18. Li T, Liang W, Xiao X, Qian Y. Nanotechnology, an alternative with promising prospects and advantages for the treatment of cardiovascular diseases. Int J Nanomedicine. 2018;13:7349-62.
19. Kirchhof P, Benussi S, Kotecha D, et al. 2016 ESC guidelines for the management of atrial fibrillation developed in collaboration with EACTS. Eur J Cardiothorac Surg. 2016;50:e1-e88.
20. Barra S, Primo J, Gonçalves H, Boveda S, Providência R, Grace A. Is amiodarone still a reasonable therapeutic option for rhythm control in atrial fibrillation? Rev Port Cardiol. 2022;41:783-9.
21. Hayward C, Patel HC, Patel K, et al. The evolving landscape of oral anti-arrhythmic prescriptions for atrial fibrillation in England: 1998-2014. Eur Heart J Cardiovasc Pharmacother. 2016;2:90-4.
22. Valembois L, Audureau E, Takeda A, Jarzebowski W, Belmin J, Lafuente-Lafuente C. Antiarrhythmics for maintaining sinus rhythm after cardioversion of atrial fibrillation. Cochrane Database Syst Rev. 2019;9:CD005049.
23. Hindricks G, Potpara T, Dagres N, et al; ESC Scientific Document Group. 2020 ESC guidelines for the diagnosis and management of atrial fibrillation developed in collaboration with the European Association for Cardio-Thoracic Surgery (EACTS): the task force for the diagnosis and management of atrial fibrillation of the European Society of Cardiology (ESC) developed with the special contribution of the European Heart Rhythm Association (EHRA) of the ESC. Eur Heart J. 2021;42:373-498.
24. Goldschlager N, Epstein AE, Naccarelli GV, et al; Practice Guidelines Sub-committee. A practical guide for clinicians who treat patients with amiodarone: 2007. Heart Rhythm. 2007;4:1250-9.
25. Raeder EA, Podrid PJ, Lown B. Side effects and complications of amiodarone therapy. Am Heart J. 1985;109:975-83.
26. Ward GH, Yalkowsky SH. Studies in phlebitis. VI: dilution-induced precipitation of amiodarone HCL. J Parenter Sci Technol. 1993;47:161-5.
27. Huynh NT, Passirani C, Saulnier P, Benoit JP. Lipid nanocapsules: a new platform for nanomedicine. Int J Pharm. 2009;379:201-9.
28. Minkov I, Ivanova T, Panaiotov I, Proust J, Saulnier P. Reorganization of lipid nanocapsules at air-water interface: part 2. properties of the formed surface film. Colloids Surf B Biointerfaces. 2005;44:197-203.
29. Vonarbourg A, Saulnier P, Passirani C, Benoit JP. Electrokinetic properties of noncharged lipid nanocapsules: influence of the dipolar distribution at the interface. Electrophoresis. 2005;26:2066-75.
30. Lamprecht A, Bouligand Y, Benoit JP. New lipid nanocapsules exhibit sustained release properties for amiodarone. J Control Release. 2002;84:59-68.
31. Lamprecht A, Ubrich N, Yamamoto H, et al. Design of rolipram-loaded nanoparticles: comparison of two preparation methods. J Control Release. 2001;71:297-306.
32. Polakovic M, Görner T, Gref R, Dellacherie E. Lidocaine loaded biodegradable nanospheres. II. modelling of drug release. J Control Release. 1999;60:169-77.
33. Akbarzadeh A, Rezaei-Sadabady R, Davaran S, et al. Liposome: classification, preparation, and applications. Nanoscale Res Lett. 2013;8:102.
34. Takahama H, Shigematsu H, Asai T, et al. Liposomal amiodarone augments anti-arrhythmic effects and reduces hemodynamic adverse effects in an ischemia/reperfusion rat model. Cardiovasc Drugs Ther. 2013;27:125-32.
35. Zhuge Y, Zheng ZF, Xie MQ, Li L, Wang F, Gao F. Preparation of liposomal amiodarone and investigation of its cardiomyocyte-targeting ability in cardiac radiofrequency ablation rat model. Int J Nanomedicine. 2016;11:2359-67.
36. Mahale NB, Thakkar PD, Mali RG, Walunj DR, Chaudhari SR. Niosomes: novel sustained release nonionic stable vesicular systems - an overview. Adv Colloid Interface Sci. 2012;183-184:46-54.
37. Moghassemi S, Hadjizadeh A. Nano-niosomes as nanoscale drug delivery systems: an illustrated review. J Control Release. 2014;185:22-36.
38. Teaima MH, Helal DA, Alsofany JM, El-Nabarawi MA, Yasser M. Ion-triggered in situ gelling intranasal spray of dronedarone hydrochloride nanocarriers: in vitro optimization and in vivo pharmacokinetic appraisal. Pharmaceutics. 2022;14:2405.
39. Ammar HO, Haider M, Ibrahim M, El Hoffy NM.
40. Conacher M, Alexander J, Brewer JM. Oral immunisation with peptide and protein antigens by formulation in lipid vesicles incorporating bile salts (bilosomes). Vaccine. 2001;19:2965-74.
41. Arzani G, Haeri A, Daeihamed M, Bakhtiari-Kaboutaraki H, Dadashzadeh S. Niosomal carriers enhance oral bioavailability of carvedilol: effects of bile salt-enriched vesicles and carrier surface charge. Int J Nanomedicine. 2015;10:4797-813.
42. Alzhrani GN, Alanazi ST, Alsharif SY, et al. Exosomes: isolation, characterization, and biomedical applications. Cell Biol Int. 2021;45:1807-31.
43. Liu L, Zhang H, Mao H, Li X, Hu Y. Exosomal miR-320d derived from adipose tissue-derived MSCs inhibits apoptosis in cardiomyocytes with atrial fibrillation (AF). Artif Cells Nanomed Biotechnol. 2019;47:3976-84.
44. Zhang W, Man Y, Chen Z. microRNA-148a in exosomes derived from bone marrow mesenchymal stem cells alleviates cardiomyocyte apoptosis in atrial fibrillation by Inhibiting SMOC2. Mol Biotechnol. 2022;64:1076-87.
45. Huang S, Deng Y, Xu J, Liu J, Liu L, Fan C. The role of exosomes and their cargos in the mechanism, diagnosis, and treatment of atrial fibrillation. Front Cardiovasc Med. 2021;8:712828.
46. Salah E, Abouelfetouh MM, Pan Y, Chen D, Xie S. Solid lipid nanoparticles for enhanced oral absorption: a review. Colloids Surf B Biointerfaces. 2020;196:111305.
47. Thi TTH, Suys EJA, Lee JS, Nguyen DH, Park KD, Truong NP. Lipid-based nanoparticles in the clinic and clinical trials: from cancer nanomedicine to COVID-19 vaccines. Vaccines (Basel). 2021;9:359.
48. Shah MK, Madan P, Lin S. Elucidation of intestinal absorption mechanism of carvedilol-loaded solid lipid nanoparticles using Caco-2 cell line as an in-vitro model. Pharm Dev Technol. 2015;20:877-85.
49. Aboud HM, El Komy MH, Ali AA, El Menshawe SF, Abd Elbary A. Development, optimization, and evaluation of carvedilol-loaded solid lipid nanoparticles for intranasal drug delivery. AAPS PharmSciTech. 2016;17:1353-65.
50. Shelley H, Babu RJ. Role of Cyclodextrins in nanoparticle-based drug delivery systems. J Pharm Sci. 2018;107:1741-53.
51. Arima H, Hayashi Y, Higashi T, Motoyama K. Recent advances in cyclodextrin delivery techniques. Expert Opin Drug Deliv. 2015;12:1425-41.
52. Pandey A. Cyclodextrin-based nanoparticles for pharmaceutical applications: a review. Environ Chem Lett. 2021;19:4297-310.
53. Hulsmans M, Clauss S, Xiao L, et al. Macrophages facilitate electrical conduction in the heart. Cell. 2017;169:510-522.e20.
54. Weissleder R, Nahrendorf M, Pittet MJ. Imaging macrophages with nanoparticles. Nat Mater. 2014;13:125-38.
55. Ahmed MS, Rodell CB, Hulsmans M, et al. A supramolecular nanocarrier for delivery of amiodarone anti-arrhythmic therapy to the heart. Bioconjug Chem. 2019;30:733-40.
56. Schaffazick SR, Pohlmann AR, Dalla-Costa T, Guterres SS. Freeze-drying polymeric colloidal suspensions: nanocapsules, nanospheres and nanodispersion. A comparative study. Eur J Pharm Biopharm. 2003;56:501-5.
57. Rao JP, Geckeler KE. Polymer nanoparticles: preparation techniques and size-control parameters. Prog Polym Sci. 2011;36:887-913.
58. Rizvi SAA, Saleh AM. Applications of nanoparticle systems in drug delivery technology. Saudi Pharm J. 2018;26:64-70.
59. Kakkar A, Traverso G, Farokhzad OC, Weissleder R, Langer R. Evolution of macromolecular complexity in drug delivery systems. Nat Rev Chem. 2017;1:63.
60. Wong PT, Choi SK. Mechanisms of drug release in nanotherapeutic delivery systems. Chem Rev. 2015;115:3388-432.
61. Motawea A, Ahmed DAM, Eladl AS, El-Mansy AAE, Saleh NM. Appraisal of amiodarone-loaded PLGA nanoparticles for prospective safety and toxicity in a rat model. Life Sci. 2021;274:119344.
62. Castro KCD, Costa JM, Campos MGN. Drug-loaded polymeric nanoparticles: a review. Int J Polym Mater Polym Biomater. 2022;71:1-13.
63. Bernkop-Schnürch A, Dünnhaupt S. Chitosan-based drug delivery systems. Eur J Pharm Biopharm. 2012;81:463-9.
64. Buyuk NI, Arayici PP, Derman S, Mustafaeva Z, Yucel S. Synthesis of chitosan nanoparticles for controlled release of amiodarone. Indian J Pharm Sci. 2020;82:131-8.
65. Asad ZUA, Yousif A, Khan MS, Al-Khatib SM, Stavrakis S. Catheter Ablation Versus Medical Therapy for Atrial Fibrillation: A Systematic Review and Meta-Analysis of Randomized Controlled Trials. Circ Arrhythm Electrophysiol. 2019;12:e007414.
66. O'Quinn MP, Dormer KJ, Huizar JF, et al. Epicardial injection of nanoformulated calcium into cardiac ganglionic plexi suppresses autonomic nerve activity and postoperative atrial fibrillation. Heart Rhythm. 2019;16:597-605.
67. Ouyang F, Tilz R, Chun J, et al. Long-term results of catheter ablation in paroxysmal atrial fibrillation: lessons from a 5-year follow-up. Circulation. 2010;122:2368-77.
68. Weerasooriya R, Khairy P, Litalien J, et al. Catheter ablation for atrial fibrillation: are results maintained at 5 years of follow-up? J Am Coll Cardiol. 2011;57:160-6.
69. Driessen AHG, Berger WR, Krul SPJ, et al. Ganglion plexus ablation in advanced atrial fibrillation: the AFACT study. J Am Coll Cardiol. 2016;68:1155-65.
70. Katritsis DG, Pokushalov E, Romanov A, et al. Autonomic denervation added to pulmonary vein isolation for paroxysmal atrial fibrillation: a randomized clinical trial. J Am Coll Cardiol. 2013;62:2318-25.
71. Srivastava P, Sharma PK, Muheem A, Warsi MH. Magnetic Nanoparticles: A Review on Stratagems of Fabrication an d its Biomedical Applications. Recent Pat Drug Deliv Formul. 2017;11:101-13.
72. Rahman MM, Islam MR, Akash S, et al. Recent advancements of nanoparticles application in cancer and neurodegenerative disorders: At a glance. Biomed Pharmacother. 2022;153:113305.
73. Farzin A, Etesami SA, Quint J, Memic A, Tamayol A. Magnetic Nanoparticles in Cancer Therapy and Diagnosis. Adv Healthc Mater. 2020;9:e1901058.
74. Yu L, Scherlag BJ, Dormer K, et al. Autonomic denervation with magnetic nanoparticles. Circulation. 2010;122:2653-9.
75. Jiang W, Xu M, Qin M, et al. Role and mechanism of lncRNA under magnetic nanoparticles in atrial autonomic nerve remodeling during radiofrequency ablation of recurrent atrial fibrillation. Bioengineered. 2022;13:4173-84.
76. Yu L, Scherlag BS, Dormer K, et al. Targeted Ganglionated Plexi Denervation Using Magnetic Nanoparticles Carrying Calcium Chloride Payload. JACC Clin Electrophysiol. 2018;4:1347-58.
77. Orrenius S, Zhivotovsky B, Nicotera P. Regulation of cell death: the calcium-apoptosis link. Nat Rev Mol Cell Biol. 2003;4:552-65.
78. Lo LW, Chang HY, Scherlag BJ, et al. Temporary suppression of cardiac ganglionated plexi leads to long-term suppression of atrial fibrillation: evidence of early autonomic intervention to break the vicious cycle of “AF Begets AF”. J Am Heart Assoc. 2016:5.
79. Oh S, Choi EK, Zhang Y, Mazgalev TN. Botulinum toxin injection in epicardial autonomic ganglia temporarily suppresses vagally mediated atrial fibrillation. Circ Arrhythm Electrophysiol. 2011;4:560-5.
80. Sergeevichev D, Fomenko V, Strelnikov A, et al. Botulinum toxin-chitosan nanoparticles prevent arrhythmia in experimental rat models. Mar Drugs. 2020;18:410.
81. Lilei Y, Huang B, Jiang H. GW27-e1123 Left cardiac sympathetic denervation with neuron targeted nanoparticles improves cardiac function and reduces ventricular arrhythmia inducibility in a canine post-infarction heart failure model. J Am Coll Cardiol. 2016;68:C66-7.
82. Yu L, Lai Y, Jiang H. Photothermal inhibition of left stellate ganglion neural activity with gold nanorods to prevent ventricular arrhythmia. J Am Coll Cardiol. 2018;71:A285.
83. Schiffer M, Carls E, Wagner K, Engelbrecht B, Duerr D, Welz A, de la JM, Pfeifer A, Fleischmann K, Roell W. Transplantation of Cx43 expressing fibroblasts: an option for postinfarct arrhythmia prevention? Thorac Cardiovasc Surg. 2019; 67(S 01):S1-S100.
84. Altshuler PJ, Schiazza AR, Luo L, et al. Superoxide dismutase-loaded nanoparticles attenuate myocardial ischemia-reperfusion injury and protect against chronic adverse ventricular remodeling. Adv Ther (Weinh). 2021;4:2100036.
85. Wang Y, Su W, Li Q, et al. Preparation and evaluation of lidocaine hydrochloride-loaded TAT-conjugated polymeric liposomes for transdermal delivery. Int J Pharm. 2013;441:748-56.
86. Mukhopadhyay S, Chakraborty P, Yusuf J, Goyal A, Tyagi S. Phenytoin in treatment of amiodarone-induced Torsades de pointes. Indian J Pharmacol. 2012;44:264-5.
87. Wang LW, Subbiah RN, Kilborn MJ, Dunn RF. Phenytoin: an old but effective antiarrhythmic agent for the suppression of ventricular tachycardia. Med J Aust. 2013;199:209-11.
88. Zhou X, Luo YC, Ji WJ, et al. Modulation of mononuclear phagocyte inflammatory response by liposome-encapsulated voltage gated sodium channel inhibitor ameliorates myocardial ischemia/reperfusion injury in rats. PLoS One. 2013;8:e74390.
89. Mathuria N, Royal ALR, Enterría-Rosales J, et al. Near-infrared sensitive nanoparticle-mediated photothermal ablation of ventricular myocardium. Heart Rhythm. 2022;19:1550-6.
90. Qi Y, Li J, Nie Q, et al. Polyphenol-assisted facile assembly of bioactive nanoparticles for targeted therapy of heart diseases. Biomaterials. 2021;275:120952.
91. Münzel T, Gori T. Nebivolol: the somewhat-different beta-adrenergic receptor blocker. J Am Coll Cardiol. 2009;54:1491-9.
92. Üstündağ-Okur N, Yurdasiper A, Gündoğdu E, Gökçe EH. Modification of solid lipid nanoparticles loaded with nebivolol hydrochloride for improvement of oral bioavailability in treatment of hypertension: polyethylene glycol versus chitosan oligosaccharide lactate. J Microencapsul. 2016;33:30-42.
93. Arshad I, Kanwal A, Zafar I, et al. Multifunctional role of nanoparticles for the diagnosis and therapeutics of cardiovascular diseases. Environ Res. 2024;242:117795.
94. Chen M, von Mikecz A. Formation of nucleoplasmic protein aggregates impairs nuclear function in response to SiO2 nanoparticles. Exp Cell Res. 2005;305:51-62.
95. Suwa T, Hogg JC, Quinlan KB, Ohgami A, Vincent R, van Eeden SF. Particulate air pollution induces progression of atherosclerosis. J Am Coll Cardiol. 2002;39:935-42.
96. Hunt NJ, Mccourt PAG, Kuncic Z, Le Couteur DG, Cogger VC. Opportunities and Challenges for Nanotherapeutics for the Aging Population. Front Nanotechnol. 2022;4:832524.
97. Vinarov Z, Abrahamsson B, Artursson P, et al. Current challenges and future perspectives in oral absorption research: An opinion of the UNGAP network. Adv Drug Deliv Rev. 2021;171:289-331.
Cite This Article

How to Cite
Download Citation
Export Citation File:
Type of Import
Tips on Downloading Citation
Citation Manager File Format
Type of Import
Direct Import: When the Direct Import option is selected (the default state), a dialogue box will give you the option to Save or Open the downloaded citation data. Choosing Open will either launch your citation manager or give you a choice of applications with which to use the metadata. The Save option saves the file locally for later use.
Indirect Import: When the Indirect Import option is selected, the metadata is displayed and may be copied and pasted as needed.
About This Article
Copyright
Data & Comments
Data
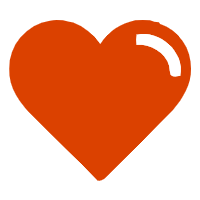
Comments
Comments must be written in English. Spam, offensive content, impersonation, and private information will not be permitted. If any comment is reported and identified as inappropriate content by OAE staff, the comment will be removed without notice. If you have any queries or need any help, please contact us at [email protected].