Modulating carrier-catalyst heterointerfaces to boost catalytic polysulfide conversion in lithium-sulfur batteries
Abstract
Enhancing the catalytic activity of sulfur cathode hosts is critical for suppressing the shuttle effect and accelerating the polysulfides redox kinetics in lithium-sulfur (Li-S) batteries. However, efficient polysulfide adsorption and catalysis conversion rely on synergistic interactions between the catalyst and the supporting carrier, particularly in optimizing catalytic site density and electron/ion transport rates. Herein, we modulate the carrier-catalyst heterointerface to enhance polysulfide conversion. Metallic 1T-phase MoS2 nanospheres are uniformly dispersed onto the nitrogen-doped graphene (N-G) sheets, forming a composite host material (1T-MoS2/N-G) for Li-S batteries. N-G serves as both a conductive substrate for charge transfer and a support for catalyst loading, while 1T-MoS2, rich in catalytic sites, functions as an efficient electrocatalyst, promoting ion diffusion, adsorbing soluble polysulfides, and accelerating their transformation into solid lithium sulfide. Benefiting from these structural and catalytic advantages, the S/1T-MoS2/N-G cathode exhibits an initial capacity of 1,296.8 mAh g-1 at 0.2 C and demonstrates outstanding cycle stabilization, with a capacity decay rate of only 0.015% per cycle over 500 cycles at 1.0 C. Even under demanding conditions, such as a sulfur loading of 6.5 mg cm-2 and a lean electrolyte of
Keywords
INTRODUCTION
Lithium-sulfur (Li-S) batteries have attracted considerable attention from both academia and industry, attributable to their exceptional theoretical specific capacity (1,675 mAh g-1 of sulfur) and economic benefits[1-4], positioning them as a promising alternative energy storage solution[5-7]. Nevertheless, the sluggish redox kinetics of sulfur results in the severe polysulfides shuttle effect and low sulfur utilization, primarily brought by the electronic insulation of active sulfur and discharge products (Li2S2/Li2S), as well as the complex solid-liquid-solid reactions involved[8-10]. To address these challenges, traditional carbon-based materials have been extensively utilized as hosts for the sulfur cathode, including carbon nanotubes (CNT)[11], graphene (G)[12], nitrogen-doped graphene (N-G)[13] and porous carbon[14], due to their high electronic conductivity and ability to confine polysulfides. However, the insufficient interaction ability between polysulfides and carbon materials is unable to efficiently suppress polysulfides shuttling[15,16]. This becomes particularly problematic in practical applications, where high sulfur loading and lean electrolyte conditions are required, making efficient polysulfides transport difficult to achieve through simple physical or chemical adsorption in host materials alone[17,18]. Thus, host materials with highly efficient catalytic polysulfides transport capabilities have significant potential for achieving high-performance Li-S batteries[19,20].
Transition metal compounds (TMCs) have been shown to effectively inhibit polysulfides shuttle effect through polar adsorption of polysulfides catalysis of intermediate transformations, thereby accelerating sulfur redox reaction and kinetics. Examples of such materials include oxides[21], sulfides[22], nitrides[23], carbides[24] and borides[25], which have exhibited advantages as host materials for high-performance sulfur cathodes[26,27]. Among these, the transition metal sulfides offer outstanding electrochemical advantages due to their high conductivity and increased edge active sites for catalytic intermediate reactions[28]. For instance, MoS2[29], Co9S8[30], VS2[31] and ZnS[32] have been confirmed improvements in polysulfides transformation kinetics. The stable chemistry of the cathode/electrolyte interface is essential for rapid charge transfer during electrocatalysis, which largely depends on the catalytically active site at the surface interface of the host material[33]. Therefore, the unique structural engineering of catalysts, particularly in regulating electron/ion transfer and polysulfides conversion, plays a critical role in achieving high-performance Li-S batteries. As a typical 2D layered structure material, there has been extensive research on the structural engineering design of MoS2 for energy storage, especially for the metallic phase 1T-MoS2 with octahedral coordination structure, which has superior electrochemical advantages compared to the semiconductive 2H-MoS2 with a triangular prismatic coordination mode. The high crystal symmetry of metallic phase 1T-MoS2 results in an accelerated charge transfer and more catalysis sites exposed in the electrocatalytic process, which obviously enhances the catalytic activity of 1T-MoS2[34]. Currently, various catalyst design methods, including single-atom catalysts[35], heterostructures[36] and defect engineering[37], have been shown to improve catalytic activity for polysulfides. However, the interaction between the catalyst and its supporting carrier is often overlooked in catalyst design. The carrier typically serves as a conductive substrate that facilitates electron transfer and catalysis, contributing to enhanced catalytic activity and accelerated sulfur redox kinetics.
In this work, we modulate the carrier-catalyst interface of 1T-MoS2/N-G to enhance catalytic activity for polysulfides transformation. The flower-like 1T-phase MoS2 (1T-MoS2) nanospheres are uniformly dispersed onto N-G sheets, forming a composite host material for the sulfur cathode. The metallic attributes of 1T-MoS2 effectively accelerate electron/ion transfer and polysulfides transformation, thereby suppressing the shuttle effect and boosting the performance of the sulfur cathodes. As a result, S/1T-MoS2/N-G exhibits stable performance with a capacity of 845.4 mAh g-1 after 500 cycles at 1 C, accompanied by a capacity decay rate of just 0.015%. Even under the sulfur loading of 6.5 mg cm-2 and electrolyte usage of 7 μL mg-1, the S/1T-MoS2/N-G cathode provides an initial capacity of 7.2 mAh cm-1, and maintains 4.8 mAh cm-1 after 100 cycles. These results demonstrate a promising approach for designing host materials that enable high-capacity and stable cycling Li-S cells.
EXPERIMENTAL
Material preparation
All chemicals for materials preparation were analytical grade without further purification in the actual operation. The synthesis of 1T-MoS2/N-G involved two separate steps: hydrothermal treatment and annealing. Firstly, PEG-20000 (0.24 mM), N-doped graphene (4 mM), (NH4)2MoO4·2H2O (10 mM) and thioacetamide (TAA) (20 mM) were separately added to a solution containing 20 mL of deionized water and 20 mL of anhydrous ethanol, stirred and ultrasonication for 0.5 h, forming a homogenous solution. And then, the obtained medley was quickly shifted into a 60 mL stainless steel autoclave with a sustained high temperature of 200 °C for 24 h until cooled to ambient temperature, after which the solid powder deposits were washed many times with deionized water and anhydrous ethanol separately, accompanied by a subsequent 60 °C oven-baked dry processing. Secondly, the acquired product was operated in a calcination process under an Ar atmosphere at 300 °C for 2 h to yield 1T-MoS2/N-G.
For S/1T-MoS2/N-G, the typical melt-diffusion technique was employed for the composite preparation. Thus, the mixture of acquired 1T-MoS2/N-G and sulfur was continuously ground for 0.5 h with a weighting ratio of 28:72, followed by uninterrupted heating for 12 h at 155 °C in an environment filled with Ar. Yet the preparation of S/N-G was adopted to use the same technique, except for the commercial N-G employed as the host materials.
Material characterization
Scanning electron microscopy (SEM, Hitachi FlexSEM1000) and transmission electron microscopy (TEM, JEOL-JEM-2100F) were applied to analyze the morphology and microstructure of the acquired materials. The power X-ray diffraction (XRD, BRUKER D8 Advance) was analyzed in the Cu Kα radiation, covering a range of 5°-80°. The Raman spectra were acquired using a RENISHAW InVia Raman Spectrometer by using a 532 nm laser. Nitrogen adsorption/desorption analysis was recorded on the BELSORP MAX II apparatus. The specific surface area calculation was used by the Barret-Joyner-Halenda (BET) model, and the pore size profile calculation was used by the Barrett-Joyner-Halenda (BJH) method. Using the thermogravimetric analyzer (Mettler Toledo, TGA/DSC) to measure sulfide content with a temperature span of 30-800 °C and a heating rate of 10 °C min-1.
Electrochemical measurements
To prepare the sulfur cathodes, the acquired composites, super P and polyvinylidene difluoride (PVDF) were mixed into N-methyl-2-pyrrolidone (NMP) respectively with a mass ratio of 8:1:1, obtaining a uniform mixture. The mixture was applied to Carbon-coated aluminum foil, then vacuum desiccated at
Polysulfides adsorption
All the samples were vacuum-dried at 60 °C overnight before the absorption test. Li2S6 solution as the identifier of polysulfides absorption was prepared by dissolving an amount of S and Li2S (5:1) in a 1:1 (V/V) mixture of DOL and DME. The solution was vigorously stirred at 60 °C in a glovebox filled with Ar for
Symmetric cell measurements
The symmetrical cells were assembled without sulfur in electrode and absence of lithium plates as counter electrodes. Li2S6 solution of (0.2 mol L-1) was made by blending stoichiometric S and Li2S (1:5) in DOL and DME with a volume of 1:1, followed by continuous stirring to form a homogeneous solution. The symmetric cell was configured with two identical electrodes with 1T-MoS2/N-G or N-G as the working electrodes and counter electrodes. 40 μL Li2S6 (0.2 mol L-1) electrolyte was dripped onto the electrode. CV was measured at a rate of 3 mV s-1 from -1 to 1 V on CHI760E.
Deposition of Li2S
Li2S8 solution was used as electrolyte for lithium sulfide deposition, and 1T-MoS2/N-G or N-G was served as working electrode and lithium flake as counter electrode for cells assembled. 0.2 M Li2S8 was prepared by dissolving stoichiometric S and Li2S (1:7) in tetraglyme with 1.0 M LiTFSI with continuous agitation to form a uniform solution. The blank electrolyte was made by 25 μL of Li2S8 electrolyte (0.2 M) being dripped onto the work electrode, and afterwards, 25 μL of contrast electrolyte, without Li2S8, was dripped onto the counter electrode. The assembled cell was galvanostatically discharged to 2.05 V at 0.03 C, and then the potential was maintained at 2.0 V on a PARSTAT MC electrochemical workstation until the current reduction to less than 10-5 A.
RESULTS AND DISCUSSION
For achieving high-performance Li-S batteries, the innovative preparation of a cathodic host for efficient sulfur redox kinetics is designed in Figure 1. As shown in Figure 1A, although the carbon-based materials introduced polar elements such as N can inhibit shuttle effect by the pure adsorption of polysulfides, the kinetics of polysulfides conversion is still sluggish. After adjusting the carrier-catalyst heterointerface by loading metallic 1T-MoS2 on the N-G [Figure 1B], the kinetics of polysulfides conversion can be obviously enhanced by the efficient catalytic activity on polysulfides and accelerated charge transfer rate via the optimized catalytic capacity and conductive properties of 1T-MoS2, which can significantly inhibit the polysulfides shuttle and facilitate the deposition of Li2S compared with the host of N-G [Figure 1C].
Figure 1. Regulating the sulfur conversion mechanism based on sulfur-host materials; (A) Carrier without catalytic active sites; (B) Modulated heterointerface of carrier-catalyst with catalyst; (C) Details of polysulfide conversion mechanism on N-G and 1T-MoS2/N-G hosts, respectively.
Structural characterization of 1T-MoS2/N-G
To obtain 1T-MoS2/N-G host material, TAA and ammonium molybdate [(NH4)2MoO4] serve as reactants for the vulcanization and molybdenum source, respectively, as schematically shown in
Figure 2. SEM pictures of (A) N-G and (B) 1T-MoS2/N-G; (C) EDS elemental mapping of 1T-MoS2/N-G; (D) TEM and (E) HRTEM pictures of 1T-MoS2/N-G [(E), inserted the corresponding FFT patterns]); The IFFT patterns of (F) Lattice profiles and (G) Lattice spacing profiles, respectively (based on the yellow boxed area in HRTEM picture (E); (F), in the covered atomic configurations, purple dot represents molybdenum atom, yellow dot represents sulfur atom); (H) XRD pattern and (I) Raman spectrum of 1T-MoS2/N-G; (J) The pore size distribution of 1T-MoS2/N-G and N-G.
As illustrated in Figure 2B and Supplementary Figure 2, the nanospheres composed of flower-like 1T-MoS2 nanosheets are uniformly dispersed on N-G, and have a size of ~500 nm. The element distribution predicted by energy-dispersion spectroscopy (EDS) of 1T-MoS2/N-G [Figure 2C] verifies that MoS2 is successfully synthesized and the load is uniformly dispersed on N-G. The crystal phase structure analysis of 1T-MoS2/N-G was further performed with TEM. The flower-like structures are further verified in Figure 2D. Upon the high-resolution TEM (HRTEM) picture in Figure 2E, in which the crystal spacing of 0.476 nm and the inserted Fast Fourier Transform (FFT) pattern correspond to the new (004) plane of 1T-MoS2. Especially, the Inverse FFT (IFFT) consequence corresponding to the boxed area in Figure 2E shows a characteristic atomic distribution of 1T-MoS2 [Figure 2F]. In addition, the lattice fringe arrangement
The XRD patterns of 1T-MoS2/N-G and N-G [Figure 2H, Supplementary Figure 3] indicate new crystal faces (002) and (004) of 1T-MoS2[38], which are in agreement with the result of the HRTEM picture in Figure 2E. Furthermore, the Raman spectrum in Figure 2I shows characteristic vibration mode peaks at 143.3 cm-1 (J1), 234.7 cm-1 (J2), 278.9 cm-1 (E1g) and 334.7 cm-1 (J3), which corresponds to the typic modes of 1T-MoS2 crystalline phase[39,40]. N2 adsorption-desorption isotherms are tested for the specific surface area and pore size profile of 1T-MoS2/N-G and N-G. The results [Figure 2J,
Effect of 1T-MoS2 on catalytic performance
The electrocatalytic transmission of soluble intermediate polysulfides is a critical factor in the continuous transformation of diffusion, adsorption, and charge-transfer process, which can ensure efficient sulfur utilization in the cathode[41]. For electrocatalytic process, the effective adsorption between base material and polysulfides plays a vital role in front of the subsequent catalytic conversion process. Firstly, the static adsorption experiments were employed to detect the adsorption performance for polysulfides by immersing 1T-MoS2/N-G or N-G into Li2S6 solution. After 5 h, the supernatant was gained and analyzed using a UV-Vis spectrophotometer [Figure 3A]. The colorless liquid after interaction with Li2S6 and a weak absorption peak in the UV-Vis spectra indicate that 1T-MoS2 can effectively strengthen the adsorption capacity of polysulfides. The bonding states and valence of 1T-MoS2/N-G before and after adsorption of Li2S6 were analyzed by XPS. In the high-resolution spectra of the Mo 3d orbit (Figure 3B, before adsorption of Li2S6), the strong peaks at 228.5 (Mo 3d5/2) and 231.6 eV (Mo 3d3/2) correspond to Mo4+ (1T-MoS2), and the weaker peaks at 225.9 and 234.8 eV correspond to the peak binding energy of S 2s and Mo6+, respectively. After the interaction with Li2S6, the XPS spectral characteristic peak of the Mo 3d orbital negatively shifts to lower binding energy, which can be attributed to the electron transfer from Li2S6 to the Mo atoms. In addition, the peaks located at 161.5 and 162.9 eV separately belong to terminal (ST-1) and bridging sulfur (SB0) atoms in the S 2p of 1T-MoS2/N-G-Li2S6, which are obviously shift to lower binding energy compared with the S 2p of proto-Li2S6 ST-1 (161.7 eV) and SB0 (163.1 eV), respectively[42] [Figure 3C]. The negative shift of the S 2p spectral peak in 1T-MoS2/N-G-Li2S6 indicates a chemical interaction between the polysulfide and 1T-MoS2. Secondly, the catalytic dynamic of 1T-MoS2 to polysulfides was investigated; the symmetric cells were assembled with 1T-MoS2/N-G and N-G as catalytic cathodes. Electrochemical impedance spectroscopy (EIS) in Figure 3D shows that the smaller interface charge transfer resistance (Rct) of 1T-MoS2/N-G (Supplementary Table 2, fitted equivalent circuit in Supplementary Figure 5) indicates a faster charge transfer rate than N-G, which is ascribed to the profitable conductivity of metallic 1T-MoS2. Furthermore, the exchange current density j0 of a catalytic electrode
Figure 3. (A) Visualization of the adsorption effect of Li2S6 by 1T-MoS2/N-G and N-G after 5 h submersion and the supernatants for UV-vis test; XPS spectra of (B) Mo 3d and (C) S 2p on interaction of 1T-MoS2/N-G with Li2S6; (D) EIS spectra and (E) exchange current density measured by symmetric cells with Li2S6 solution of 1T-MoS2/N-G and N-G; (F) CV curves measured by symmetric cells with
where R, T, n and F represent gas content, absolute temperature, number of elements being transferred and Faraday content, respectively, and Rct is charge transfer impedance from Figure 3D, Supplementary Table 2. The optimized exchange current density of 1T-MoS2/N-G exhibits a faster charge transfer from the electrode reaction compared to N-G. In addition, the CV curves of the symmetric cell deliver a significantly high current response of 1T-MoS2/N-G, thus demonstrating an efficient catalytic activity for polysulfides conversion[44] [Figure 3F], which is well matched with the results of j0.
Due to the continuous conversion reactions of dissolution-deposition mechanism of sulfur cathode, the catalytic efficiency of polysulfides mainly depends on the conversion capacity from soluble Li2Sx to Li2S2 and Li2S[45]. To further examine how catalysts regulate the nucleation and growth of solid discharge products, the Li2S deposition experiment was carried out. As we can see in Figure 3G and H, the Li2S deposition amount on 1T-MoS2/N-G substrate is obviously larger and the response time is shorter than N-G, which has a consistent trend with the EIS analysis, indicating a diminished energy barrier for Li2S deposition on 1T-MoS2 substrate surface. Thus, the calculated Li2S deposition capacity for 1T-MoS2/N-G is 298.1 mAh g-1 based on Faraday's law[46], which is higher than that of N-G (96.9 mAh g-1), showing a stronger redox kinetics to catalyze polysulfides to Li2S.
Based on the above electrochemical analysis, the comprehensive electrochemical performance of the 1T-MoS2/N-G host materials can be quantitatively assessed. Also, the Dc reflects the amount of Li2S deposition, the peak current density Ip of the CV curve corresponds to ionic diffusion rate at the substrate interface and the exchange current density j0 of catalytic electrode demonstrates the sulfur electrode reaction kinetics. Therefore, Dc, j0, and Ip are summarized in Figure 3I for synthetic comparison. Obviously, the catalytic capacity of 1T-MoS2/N-G is stronger, resulting in improved redox kinetics for polysulfides intermediate transformation.
Characterization of structure and electrochemical performance of S/1T-MoS2/N-G and S/N-G
The S/1T-MoS2/N-G and S/N-G were synthesized by the classical molten diffusion strategy[47]. The SEM images in Supplementary Figure 6 show that the sulfur is uniformly loaded on the host material, and the overall structure morphology after merging sulfur remains basically unchanged, indicating a dense recombination with sulfur. Moreover, XRD pattern [Supplementary Figure 7A] reveals that sulfur exists in the composite as the monoclinic phase[48], and the sulfur content of the two composites is basically maintained at ~72 wt% on the basis of thermogravimetric results (TGA) in Supplementary Figure 7B. The specific surface area and pore size profile of 1T-MoS2/N-G and N-G after sulfur melting
Furthermore, S/1T-MoS2/N-G and S/N-G were used to assemble the Li-S batteries for electrochemical testing. Figure 4A showcases the typical CV curves of Li-S batteries[49] using S/1T-MoS2/N-G and S/N-G as sulfur cathodes. Compared to S/N-G, S/1T-MoS2/N-G exhibits a reinforced peak current and a sharper peak shape in the cathodic/anodic reaction [Supplementary Figure 9]. Especially, the CV peak of S/1T-MoS2/N-G battery has less overpotential in the redox electrochemical process [Figure 4B]. The Tafel curves were applied to further assess the electrocatalytic properties of 1T-MoS2 during redox reaction. Tafel slope is used to describe the polarization of the electrode based on the relationship between overpotential and current density during electrochemical reaction. The smaller value reveals an accelerated electrode response and enhanced electrochemical kinetics. In Li-S batteries, the smaller Tafel slope verifies a strong dynamic in sulfur redox reaction and an efficient catalytic polysulfide conversion[50]. As displayed in Figure 4C-E, the fitting slopes of peaks a, b, and c for S/1T-MoS2/N-G cell are 44.79, 25.24 and 40.85 mV dec-1, respectively, which are distinctly lower than S/N-G cell (46.26, 42.57, 49.73 mV dec-1, respectively), indicating an accelerated polysulfides redox kinetics than S/N-G. The consistent electrochemical property is also demonstrated by EIS test in Figure 4F. Comparing the fitting circuit element data analysis in
Figure 4. (A) CV curves of S/1T-MoS2/N-G and S/N-G cathodes with a scan rate of 0.1 mV s-1 and (B) overpotential diagram; Tafel plots of (C) peak c, (D) peak b, and (E) peak a based on the CV curves of (A); (F) EIS spectra of S/1T-MoS2/N-G and S/N-G cathodes before cycle; (G) CV curves of S/1T-MoS2/N-G at the different scan rate from 0.05 to 0.4 mV s-1, and (H and I) the relationships between the peak current and scan rate for different reaction processes based on (G).
The batteries with S/1T-MoS2/N-G and S/N-G as the cathode were used to estimate the cyclic performance in Figure 5. The capacity contribution of 1T-MoS2/N-G is negligible [Supplementary Figure 12A and B] in the voltage interval of Li-S batteries (1.7-2.8 V vs. Li+/Li). The initial charge-discharge plateaus of S/1T-MoS2/N-G [Figure 5A] show a typical multistep discharge profile and deliver a higher discharge capacity of 1,296.8 mAh g-1 at 0.2 C, which is higher than S/N-G (1,002.1 mAh g-1). Besides, the overpotential of S/1T-MoS2/N-G is significantly smaller (0.172 V) than that of S/N-G cathode (0.204 V, in
Figure 5. (A) The initial charge-discharge plateaus of S/1T-MoS2/N-G and S/N-G electrodes at 0.2 C and (B) corresponding enlarged view; (C) Cycling performance at 0.2 C; (D) Valuation of the multiplicity cycles and (E) charge-discharge plateaus of S/1T-MoS2/N-G cathode; (F) Cycling performance with highly sulfur loading and lean electrolyte/sulfur ratio; (G) Long cycling performances at 1 C; (H) Comprehensive performance comparison diagram.
Furthermore, S/1T-MoS2/N-G cathodes can also achieve superior electrochemical performance with harsh conditions of high sulfur loading and low E/S (electrolyte/sulfur ratio) for practical inquiry. The typical multistep discharge profile of S/1T-MoS2/N-G can be maintained with a slight decrease in discharge potential. Thus, the initial discharge capacity can reach 1,108 mAh g-1 with a sulfur loading of
The crystal phase structure of 1T-MoS2 can be stably maintained in the charging and discharging cycle. After cycling 200 times at 0.2 C, the S/1T-MoS2/N-G cathode was characterized by TEM. As revealed in Supplementary Figure 16, the cycled electrodes show a typical 2D sheet stack morphology
CONCLUSIONS
In summary, by modulating the carrier-catalyst heterointerfaces, the kinetics of polysulfides transformation in the sulfur cathode are significantly enhanced. The metallic 1T-MoS2 phase, featuring a nanoflower structure, is successfully incorporated into the sulfur cathode, resulting in high-performance Li-S batteries. The metallic 1T-MoS2, composed of numerous nanosheets, provides efficient conductivity for electron and ion transport. Additionally, 1T-MoS2 loaded on N-G not only effectively chemisorbs intermediate polysulfides but also accelerates the interconversion of soluble lithium polysulfides and solid Li2S2/Li2S, thereby improving the sulfur redox kinetics. Benefiting from the superb catalytic activity of 1T-MoS2, the
DECLARATIONS
Authors’ contributions
Experimentation: Jin, H.
Investigation, methodology: Jin, H.; Deng, T.; Jin, Z.; Zhang, Y.
Materials characterization: Jin, H.; Yang, C.; Ren, Y.; Li, Y.; Chen, X.; Yang, H.
Data analysis: Jin, H.; Li, H.; Yang, C.; Pan, S.; Yin, S.
Conceptualization and supervision: Li, H.; Wang, X.; Pan, K.
Writing-original draft: Jin, H.; Li, H.; Xi, K.; Yang, C.
Review and editing: Li, H.; Xi, K.
Availability of data and materials
The data are available upon request from the authors.
Financial support and sponsorship
This work was supported by the National Energy Storage Platform, Key Technologies R & D Program of Henan Province (232102240009, 252102240069), Major Science and Technology Projects of Henan Province (241100240400), Major Scientific and Technological Innovation Project in Henan Province (231100220100), Longmen Laboratory Free Exploration Project (LMQYTSKT012, LMQYTSKT015) and Natural Science Foundation Project of Henan Province (232300420087), the National Natural Science Foundation of China (No. 92472124 and No. 22278329).
Conflicts of interest
All authors declared that there are no conflicts of interest.
Ethical approval and consent to participate
Not applicable.
Consent for publication
Not applicable.
Copyright
© The Author(s) 2025.
Supplementary Materials
REFERENCES
1. Wang, P.; Xi, B.; Huang, M.; Chen, W.; Feng, J.; Xiong, S. Emerging catalysts to promote kinetics of lithium-sulfur batteries. Adv. Energy. Mater. 2021, 11, 2002893.
2. Chung, S. H.; Manthiram, A. Current status and future prospects of metal-sulfur batteries. Adv. Mater. 2019, 31, e1901125.
3. Evers, S.; Nazar, L. F. New approaches for high energy density lithium-sulfur battery cathodes. Acc. Chem. Res. 2013, 46, 1135-43.
4. Xia, S.; Xu, X.; Wu, W.; et al. Advancements in functionalized high-performance separators for lithium-sulfur batteries. Mater. Sci. Eng. R. Rep. 2025, 163, 100924.
5. Kamaya, N.; Homma, K.; Yamakawa, Y.; et al. A lithium superionic conductor. Nat. Mater. 2011, 10, 682-6.
6. Wang, J.; Li, G.; Luo, D.; et al. Engineering the conductive network of metal oxide-based sulfur cathode toward efficient and longevous lithium-sulfur batteries. Adv. Energy. Mater. 2020, 10, 2002076.
7. He, X.; Bresser, D.; Passerini, S.; et al. The passivity of lithium electrodes in liquid electrolytes for secondary batteries. Nat. Rev. Mater. 2021, 6, 1036-52.
8. Guo, Y.; Niu, Q.; Pei, F.; et al. Interface engineering toward stable lithium-sulfur batteries. Energy. Environ. Sci. 2024, 17, 1330-67.
9. Qu, Z.; Zhang, X.; Xiao, R.; Sun, Z.; Li, F. Application of organosulfur compounds in lithium-sulfur batteries. Acta. Phys. Chim. Sin. 2023, 39, 2301019.
10. Bi, C. X.; Yao, N.; Li, X. Y.; et al. Unveiling the reaction mystery between lithium polysulfides and lithium metal anode in lithium-sulfur batteries. Adv. Mater. 2024, 36, e2411197.
11. Cheng, X.; Huang, J.; Zhang, Q.; Peng, H.; Zhao, M.; Wei, F. Aligned carbon nanotube/sulfur composite cathodes with high sulfur content for lithium-sulfur batteries. Nano. Energy. 2014, 4, 65-72.
12. Fang, R.; Chen, K.; Yin, L.; Sun, Z.; Li, F.; Cheng, H. M. The regulating role of carbon nanotubes and graphene in lithium-ion and lithium-sulfur batteries. Adv. Mater. 2019, 31, e1800863.
13. Qiu, Y.; Li, W.; Zhao, W.; et al. High-rate, ultralong cycle-life lithium/sulfur batteries enabled by nitrogen-doped graphene. Nano. Lett. 2014, 14, 4821-7.
14. Li, G.; Sun, J.; Hou, W.; Jiang, S.; Huang, Y.; Geng, J. Three-dimensional porous carbon composites containing high sulfur nanoparticle content for high-performance lithium-sulfur batteries. Nat. Commun. 2016, 7, 10601.
15. Zhou, G.; Chen, H.; Cui, Y. Formulating energy density for designing practical lithium-sulfur batteries. Nat. Energy. 2022, 7, 312-9.
16. Li, J.; Gao, L.; Pan, F.; et al. Engineering strategies for suppressing the shuttle effect in lithium-sulfur batteries. Nanomicro. Lett. 2023, 16, 12.
17. Li, S.; Wang, W.; Duan, H.; Guo, Y. Recent progress on confinement of polysulfides through physical and chemical methods. J. Energy. Chem. 2018, 27, 1555-65.
18. Lian, Z.; Ma, L.; Wu, H.; et al. Accelerating sulfur redox kinetics by rare earth single-atom electrocatalysts toward efficient lithium-sulfur batteries. Appl. Catal. B. Environ. Energy. 2025, 361, 124661.
19. Huang, Y.; Lin, L.; Zhang, C.; et al. Recent advances and strategies toward polysulfides shuttle inhibition for high-performance Li-S batteries. Adv. Sci. 2022, 9, e2106004.
20. Wang, P.; Xi, B.; Xiong, S. Insights into the optimization of catalytic active sites in lithium-sulfur batteries. Acc. Chem. Res. 2024, 57, 2093-104.
21. Wang, B.; Ren, Y.; Zhu, Y.; et al. Construction of Co3O4/ZnO heterojunctions in hollow N-doped carbon nanocages as microreactors for lithium-sulfur full batteries. Adv. Sci. 2023, 10, e2300860.
22. Liu, L.; Yan, M.; Zhao, X.; Pan, H. A novel pathway for sustained sulfides conversion via electrocatalyst-modified separator in lithium-sulfur batteries. Nano. Energy. 2024, 130, 110122.
23. Yang, M.; Liu, P.; Qu, Z.; et al. Nitrogen-vacancy-regulated Mo2N quantum dots electrocatalyst enables fast polysulfides redox for high-energy-density lithium-sulfur batteries. Nano. Energy. 2022, 104, 107922.
24. Yuan, H.; Zheng, J.; Lu, G.; et al. Formation of 2D amorphous lithium sulfide enabled by Mo2C clusters loaded carbon scaffold for high-performance lithium sulfur batteries. Adv. Mater. 2024, 36, e2400639.
25. He, J.; Bhargav, A.; Manthiram, A. Molybdenum boride as an efficient catalyst for polysulfide redox to enable high-energy-density lithium-sulfur batteries. Adv. Mater. 2020, 32, e2004741.
26. Chen, L.; Cao, G.; Li, Y.; et al. A review on engineering transition metal compound catalysts to accelerate the redox kinetics of sulfur cathodes for lithium-sulfur batteries. Nanomicro. Lett. 2024, 16, 97.
27. Pan, H.; Cheng, Z.; Zhou, Z.; et al. Boosting lean electrolyte lithium-sulfur battery performance with transition metals: a comprehensive review. Nanomicro. Lett. 2023, 15, 165.
28. Wu, J.; Ye, T.; Wang, Y.; et al. Understanding the catalytic kinetics of polysulfide redox reactions on transition metal compounds in Li-S batteries. ACS. Nano. 2022, 16, 15734-59.
29. Zhang, J.; Xie, Z.; Xi, W.; et al. 3D printing of tungstate anion modulated 1T-MoS2 composite cathodes for high-performance lithium-sulfur batteries. Adv. Energy. Mater. 2024, 14, 2401792.
30. Li, H.; Song, Y.; Xi, K.; et al. Sulfur vacancies in Co9S8-x/N-doped graphene enhancing the electrochemical kinetics for high-performance lithium-sulfur batteries. J. Mater. Chem. A. 2021, 9, 10704-13.
31. Liu, G.; Zeng, Q.; Fan, Z.; et al. Boosting sulfur catalytic kinetics by defect engineering of vanadium disulfide for high-performance lithium-sulfur batteries. Chem. Eng. J. 2022, 448, 137683.
32. Wang, J.; Zhao, Y.; Li, G.; et al. Aligned sulfur-deficient ZnS1-x nanotube arrays as efficient catalyzer for high-performance lithium/sulfur batteries. Nano. Energy. 2021, 84, 105891.
33. Zhong, Y.; Yin, L.; He, P.; Liu, W.; Wu, Z.; Wang, H. Surface chemistry in cobalt phosphide-stabilized lithium-sulfur batteries. J. Am. Chem. Soc. 2018, 140, 1455-9.
34. Chen, X.; Wang, Z.; Wei, Y.; et al. High phase-purity 1T-MoS2 ultrathin nanosheets by a spatially confined template. Angew. Chem. Int. Ed. 2019, 58, 17621-4.
35. Wang, J.; Cao, G.; Duan, R.; Li, X.; Li, X. Advances in single metal atom catalysts enhancing kinetics of sulfur cathode. Acta. Phys. Chim. Sin. 2023, 39, 2212005.
36. Hu, S.; Wang, T.; Lu, B.; et al. Ionic-liquid-assisted synthesis of FeSe-MnSe heterointerfaces with abundant Se vacancies embedded in N,B co-doped hollow carbon microspheres for accelerating the sulfur reduction reaction. Adv. Mater. 2022, 34, e2204147.
37. Wu, X.; Xie, R.; Cai, D.; et al. Engineering defect-rich bimetallic telluride with dense heterointerfaces for high-performance lithium-sulfur batteries. Adv. Funct. Mater. 2024, 34, 2315012.
38. Li, H.; Chen, S.; Jia, X.; et al. Amorphous nickel-cobalt complexes hybridized with 1T-phase molybdenum disulfide via hydrazine-induced phase transformation for water splitting. Nat. Commun. 2017, 8, 15377.
39. Qi, K.; Cui, X.; Gu, L.; et al. Single-atom cobalt array bound to distorted 1T MoS2 with ensemble effect for hydrogen evolution catalysis. Nat. Commun. 2019, 10, 5231.
40. Yu, Y.; Nam, G. H.; He, Q.; et al. High phase-purity 1T'-MoS2- and 1T'-MoSe2-layered crystals. Nat. Chem. 2018, 10, 638-43.
41. Shi, Z.; Ding, Y.; Zhang, Q.; Sun, J. Electrocatalyst modulation toward bidirectional sulfur redox in Li-S batteries: from strategic probing to mechanistic understanding. Adv. Energy. Mater. 2022, 12, 2201056.
42. Ye, Z.; Jiang, Y.; Li, L.; Wu, F.; Chen, R. Synergetic anion vacancies and dense heterointerfaces into bimetal chalcogenide nanosheet arrays for boosting electrocatalysis sulfur conversion. Adv. Mater. 2022, 34, e2109552.
43. Li, G. R.; Gao, X. P. Low-cost counter-electrode materials for dye-sensitized and perovskite solar cells. Adv. Mater. 2020, 32, e1806478.
44. Deng, T.; Wang, J.; Zhao, H.; et al. Dynamically regulating polysulfide degradation via organic sulfur electrolyte additives in lithium-sulfur batteries. Adv. Energy. Mater. 2024, 14, 2402319.
45. Li, Z.; Li, P.; Meng, X.; Lin, Z.; Wang, R. The interfacial electronic engineering in binary sulfiphilic cobalt boride heterostructure nanosheets for upgrading energy density and longevity of lithium-sulfur batteries. Adv. Mater. 2021, 33, e2102338.
46. Wang, J.; Yi, S.; Liu, J.; et al. Suppressing the shuttle effect and dendrite growth in lithium-sulfur batteries. ACS. Nano. 2020, 14, 9819-31.
47. Zhang, L.; Liu, D.; Muhammad, Z.; et al. Single nickel atoms on nitrogen-doped graphene enabling enhanced kinetics of lithium-sulfur batteries. Adv. Mater. 2019, 31, e1903955.
48. Li, H.; Xi, K.; Wang, W.; Liu, S.; Li, G.; Gao, X. Quantitatively regulating defects of 2D tungsten selenide to enhance catalytic ability for polysulfide conversion in a lithium sulfur battery. Energy. Storage. Mater. 2022, 45, 1229-37.
50. Wang, M.; Fan, L.; Sun, X.; et al. Nitrogen-doped CoSe2 as a bifunctional catalyst for high areal capacity and lean electrolyte of Li-S battery. ACS. Energy. Lett. 2020, 5, 3041-50.
51. Liu, Y. T.; Liu, S.; Li, G. R.; Yan, T. Y.; Gao, X. P. High volumetric energy density sulfur cathode with heavy and catalytic metal oxide host for lithium-sulfur battery. Adv. Sci. 2020, 7, 1903693.
52. Zhang, G.; Feng, L.; Yu, J.; Wang, S. Full potential catalysis of Co0.4Ni1.6P-V/CNT with phosphorus vacancies for Li2S1-2 deposition/decomposition and S8/Li2Sn (3 ≤ n ≤ 8) conversion in Li-S batteries. ACS. Appl. Mater. Interfaces. 2023, 15, 49170-80.
53. Hu, S.; Huang, X.; Zhang, L.; et al. Vacancy-defect topological insulators Bi2Te3-x embedded in N and B Co-doped 1D carbon nanorods using ionic liquid dopants for kinetics-enhanced Li-S batteries. Adv. Funct. Mater. 2023, 33, 2214161.
Cite This Article

How to Cite
Download Citation
Export Citation File:
Type of Import
Tips on Downloading Citation
Citation Manager File Format
Type of Import
Direct Import: When the Direct Import option is selected (the default state), a dialogue box will give you the option to Save or Open the downloaded citation data. Choosing Open will either launch your citation manager or give you a choice of applications with which to use the metadata. The Save option saves the file locally for later use.
Indirect Import: When the Indirect Import option is selected, the metadata is displayed and may be copied and pasted as needed.
About This Article
Copyright
Data & Comments
Data
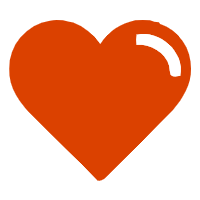
Comments
Comments must be written in English. Spam, offensive content, impersonation, and private information will not be permitted. If any comment is reported and identified as inappropriate content by OAE staff, the comment will be removed without notice. If you have any queries or need any help, please contact us at [email protected].