Molecular engineering toward large pore-sized covalent organic frameworks
Abstract
In recent years, covalent organic frameworks (COFs) as designable crystalline porous polymers have attracted widespread attention because of their tunable structures and functionalities. In particular, the unique characteristics of COFs, such as readily controllable pore size, high surface area, editable pore surface environment, and exceptional chemical stability, provide a structural basis for loading large-sized organic, inorganic, and biological molecules for hetero-catalysis, energy storage, and other applications. In this review, we discuss state-of-the-art strategies for the structural design and synthesis, properties, and functionalities of large pore size two-dimensional and three-dimensional COFs, spotlighting recent breakthrough achievements and remarkable progress to guide further efforts in this field.
Keywords
INTRODUCTION
Exploring crystalline porous materials has recently become a focal research topic due to their great potential in solving energy and environmental issues and promoting sustainable social development[1-3]. Covalent organic frameworks (COFs) integrate organic building blocks into an ordered lattice through covalent bonds. With the advantages of their ordered and stable pore structure, large specific surface area, and functionalizable skeleton, COFs have elicited growing interest and have been widely applied in numerous fields[4], such as gas separation and sorption, catalysis, energy storage and conversion, sensors, semiconductors, spintronics, drug delivery, water desalination, etc. Since the first synthesis of boronic ester-linked COF by Côté et al. in 2005[5], in 2007, their group reported four examples of three-dimensional (3D) COFs, namely COF-102, COF-103, COF-105, and COF-108[6]. In 2016, Zhuang et al. reported for the first carbon-carbon double-bond connected stable COF, two-dimensional (2D) poly(phenelyenevinylene) framework (2DPPV), which has an attractive application prospect in the field of optoelectronics due to the high degree of π-π conjugation[7]. In 2017, Jin et al. also reported a similar carbon-carbon double-bond connected COF, sp2c-COF, with full sp2c conjugation covering the fundamental properties of COFs in terms of magnetism, free radicals, and so on[8]. In the same year, Ma et al. achieved the first large-size, single-crystal growth and X-ray diffraction (SXRD) structure analysis of 3D COFs based on imine bonding[9]. Over the past few decades, hundreds of COFs with versatile chemical and topological structures have been developed[10-18]. The representative advantages of COFs over other porous analogs, such as zeolites, metal-organic frameworks (MOFs), polymers of intrinsic microporosity (PIMs), covalent triazine frameworks (CTFs), etc., lie in the larger pore size, stable and ordered nature, exceptionally high surface area, and controllable functionalization by pore-surface engineering.
To date, most reported COFs are micro- or small mesoporous COFs with pore sizes ranging from 0.5 to
OVERVIEW OF 2D COFS WITH LARGE PORES
For the skeleton of 2D COFs, organic motifs undergo topological polymerization via condensation to form layered structures, which are further vertically stacked to form periodic columns through interlayer π-π interactions. The pore size and shape of the formed ordered open nano-channels are closely related to the stacking modules of the 2D layers. Conventional strategies to explore large linkers with intense stacking interactions and suitable solubility have enabled the construction of 2D COFs with well-defined porous structures and pore sizes up to 4.0 nm. However, preparing 2D COFs with even larger pore sizes remains challenging. A key fact hindering the development of COFs with larger pores is that the 2D layers tend to stabilize the networks by lateral sliding, a common way of reducing free energy in COFs. In 2010, based on the Density Functional Theory (DFT), Lukose et al. proposed four interlayer stacking modes of 2D COFs, namely, eclipsed (AA), staggered (AB), serrated, and inclined stacking[33,34]. According to this work, 2D COFs tend to stack in a way that exhibits the lowest energy. AA stacking is most likely to construct large pores due to enough space for alleviating steric repulsion, while AB or ABC stacking is difficult to construct large pores due to significant interlayer slipping. Consequently, the pore size and surface area of COFs are diminished because of the structural collapse and pore occlusion, which limits the successful construction of large pore-sized structures.
To date, several promising approaches have been developed to address this crucial issue. They can generally be classified as follows: (A) rational design of 2D COFs with honeycomb (hcb) and kagome (kgm) topologies, which make it easier to form larger pore size compared to other topologies; (B) employing specific linkages such as imides to generate large mesopores in the skeleton of 2D COFs; (C) using large molecule-sized building blocks with charged parts or branched side-chains to prevent the pore shrinkage and interlayer slippage, resulting in the formation of 2D COFs with large pores.
Topology-oriented synthetic strategy
Along with the report of Star-COF-1, Star-COF-2, and Star-COF-3 with pore sizes of 3.9, 4.6, and 4.7 nm by Feng et al. in 2012, the kgm topological COFs with large pores gradually came into view[35]. In contrast to the dual-pore COF[36] with a pore size of 2.7 nm, synthesized by the condensation of 4,4′,4′′,4′′′-(ethene-1,1,2,2-tetrayl)-tetraaniline (ETTA) and terephthalaldehyde, the large cyclic fragments of Star-COFs yield larger pore apertures in the center of topology.
In 2016, Crowe et al. reported three novel COFs containing homogeneous and heterogeneous distributions of π-conjugated dehydrobenzoannulene (DBA), DBA-12, and DBA-18 units with varied triangular molecular sizes[37]. Polycondensation between the C3-symmetric monomeric catechol DBA-12 or DBA-18 and C2-symmetric pyrene-2,7-dibronic acid (PDBA) yielded three kgm topological COFs, 3.8 nm for
Figure 1. Schematics for the synthesis of Py-MV-DBA-COF and Py-DBA-COF 2. (i) 3-Pentanone/Mesitylene = 2/1 (v/v), 105 °C and 3 days; (ii) 3-Pentanone/Mesitylene = 2/1 (v/v), 105 °C and 3 days. DBA: Dehydrobenzoannulene; COF: covalent organic framework.
The polycondensations between two C2-symmetric units or a C3-symmetric cyclic molecule and a
Linkage-oriented synthetic strategy
COFs can be attached through a variety of methods, such as the boronate esters, triazines, azine bonds,
In 2014, Fang et al. successfully synthesized imide-linked COFs for the first time via the condensation reaction between extended aromatic dianhydrides and triamine building blocks[21]. Accordingly, a series of highly crystalline polyimide (PI)-COFs were yielded with a large pore size of 5.3 nm and a considerable surface area of 2,346 m2/g [Figure 2]. These PI-COFs are thermally robust up to 530 °C. Afterward, a large dye molecule, rhodamine B, with dimensions of about 10 × 16 Å2, was incorporated into the pore of PI-COF-3 to make temperature-sensing devices, which is not possible for COFs with pore sizes less than
Figure 2. Skeleton design of PI-COF-3 with imide linkage. (i) Mesitylene/N-methyl-2-pyrrolidone/Isoquinoline = 1/1/1 (v/v/v), 250 °C and 7 days. COF: Covalent organic framework.
The most likely reason for the successful synthesis of large-pore boronate ester and imine-linked COFs is that the identical components of naphthalene diimide or perylene diimide contain fewer structural rotating sites than simple multi-phenyl ligands [Figure 3].
Fragment-oriented synthetic strategies
In 2016, Yu et al. reported an imine-linked polycationic (PC) 2D COF through the condensation of 1,3,5-tris(4-aminophenyl) benzene (TAPB) and 1,1-bis(4-formylphenyl)-4,4′-bipyridinium dichloride
Figure 4. Synthesis route of PC-COF. (i) o-DCB:Ethanol = 9/1 (v/v), HAc (6 M), 120 °C and 7 days. TAPB: 1,3,5-tris(4-aminophenyl) benzene; BFBP2+: 1,1-bis(4-formylphenyl)-4,4′-bipyridinium; COF: covalent organic framework; DCB: dichlorobenzene.
While the pore sizes of most reported MOFs are smaller than 3.0 nm[39], in 2015, Cho et al. reported a
Figure 5. Synthesis routes of dPP-TAB COF and dPP-TAPB COF. (i) Mesitylene/1,4-Dioxane = 7/3 (v/v), HAc (12 M), 120 °C and 3 days; (ii) Mesitylene/1,4-Dioxane = 3/1 (v/v), HAc (6 M), 120 °C and 3 days. TAPB: 1,3,5-tris(4-aminophenyl) benzene; COF: covalent organic framework.
As shown in Figure 6, the incorporation of methoxy substituents close to the imine linkage enables the diminishment of the intrinsic dipole moment and tuning of interlayer interactions. Consequently, the crystallinity of large porous lattices can be well maintained.
Figure 6. Schematic representation of the synthesis mechanism of large-pore dpp-TAPB COF. Reproduced with permission from ref.[29]. Copyright © 2021 The Authors. Published by American Chemical Society. This publication is licensed under CC-BY 4.0. TAPB: 1,3,5-tris(4-aminophenyl) benzene; COF: covalent organic framework.
In 2022, based on the same strategy as above, Mu et al. realized the synthesis of a 2D COF with a record pore size of 10 nm, termed TDCOF-3 [Figure 7][41]. This milestone is achieved by creatively integrating methoxy side chains and alkyne units into a single monomeric structure. Such a combination not only enlarges the length of building blocks but also overcomes the intrinsic flexibility of growing polymeric chains. The resultant large pore-sized 2D COF is capable of separating 7.0 nm pepsin and protecting tyrosinase from heat-induced inactivation. Furthermore, this work also provides conclusive evidence that large mesoporous COFs can efficiently load enzymes of large molecular size and act as a protective layer to avoid enzyme inactivation under stringent conditions.
Figure 7. Schematic of TDCOF-3. (i) Mesitylene/1,4-Dioxane = 1/1 (v/v), HAc (6 M), 120 °C and 5 days.
For TDCOF-3 with a pore size of 10.0 nm, the attachment of dimethoxy groups to the identical side of the phenyl ring also modulates interlayer interaction induced by local polarity. Besides, the insertion of carbon-carbon triple bonds to the main chain enhances the planarity and weakens the steric hindrance, leading to low nonbond energy. As a result, the ordered structure of TDCOF-3 with a record pore size was constructed with low nonbond energy [Figure 8].
Figure 8. Calculation of the series of TDCOFs with the corresponding nonbond energies. Reproduced with permission from ref.[41]. Copyright © 2022, American Chemical Society.
For 3D COFs, the synthesis of large pore sizes is determined by various structural factors, and no definite mechanism has been reported in the literature. Therefore, the related explanations have been left for future work.
From the above examples, the approach of enlarging pore sizes of COFs by growing the length of monomer fragments is mainly available for hcb topological structures. For other topologies, the pore deformation might occur more significantly, making it more challenging to retain large pores.
As shown in Tables 1 and 2, the most common strategy for the synthesis of large pores is extending the length of the monomer fragments to increase the pore sizes of COFs, which ultimately leads to more intense pore collapses. To retain the large pore aperture, unique substituents, including methoxy, alkyl, etc., have to be inserted into the building blocks, which increases the difficulty of monomer synthesis and leads to a sharp drop in total yield. Besides, researchers also choose to develop new topologies to create large pores, which in itself is another challenge for COFs. From the above instances, reaction time plays a vital role in the synthesis of 2D large-pore COFs. The dynamic equilibrium of imine bond formation requires a longer time to form the large-pore COF with high crystallinity. Therefore, prolonging the time can be a potential approach to obtain large pores in 2D COFs.
Summary of the porosity of the 2D COFs mentioned above
No. | Compound | Pore size (nm)c | BET (m2/g) | Major peak position (Degree) | Pore volume (cm3/g) | Ref. |
1 | DCuPc-APDI-COFb | 4.0 | 414 | 2.1 | 0.4 | [38] |
2 | DZnPc-APDI-COFb | 4.0 | 519 | 2.1 | 0.6 | [38] |
3 | Py-MV-DBA-COF | 4.1 | 1,134 | 2.3 | 1.1 | [37] |
4 | Py-DBA-COF 2 | 4.3 | 1,354 | 2.2 | 1.2 | [37] |
5 | Star-COF-2b | 4.6 | 1,538 | 2.2 | 1.0 | [35] |
6 | Star-COF-3b | 4.7 | 1,489 | 2.2 | 1.1 | [35] |
7 | PP-TABa,b | 4.8 | 1,063 | 1.5-2.0 | 1.1-1.5 | [29] |
8 | mPP-TABa,b | 4.8 | 1,823 | 1.5-2.0 | 1.6-2.2 | [29] |
9 | dPP-TABa | 4.8 | 1,467 | 1.5-2.0 | - | [29] |
10 | DTP-ANDI-COFa,b | 5.1 | 478 | 1.9 | 0.7 | [20] |
11 | PI-COF-3 | 5.3 | 2,346 | 2.0 | 1.3 | [21] |
12 | PC-COF | 5.8 | - | 1.8 | - | [22] |
13 | PP-TAPBa | 5.8 | 1,032 | 1.2-1.8 | 3.1-3.3 | [29] |
14 | mPP-TAPBa | 5.8 | 631 | 1.2-1.8 | 0.6-0.8 | [29] |
15 | dPP-TAPBa | 5.8 | 1,670 | 1.2-1.8 | - | [29] |
16 | Ttba-TPDA-COF | 5.8 | 726 | 1.6 | 0.8 | [23] |
17 | COF-122a,b | 6.2 | 646 | 2.1 | 0.6 | [24] |
18 | TDCOF-1 | 7.7 | 1,665 | 1.5 | 2.0 | [41] |
19 | TDCOF-2 | 7.7 | 1,560 | 1.5 | 2.1 | [41] |
20 | TDCOF-3 | 10 | 1,270 | 1.0 | 2.1 | [41] |
Summary of the synthetic strategies of 2D COFs
No. | Compound | Synthetic strategy | Ref. |
1 | Star-COF-2 | Topology-oriented | [35] |
2 | Star-COF-3 | Topology-oriented | [35] |
3 | Py-MV-DBA-COF | Topology-oriented | [37] |
4 | Py-DBA-COF 2 | Topology-oriented | [37] |
5 | DCuPc-APDI-COF | Linkage-oriented | [38] |
6 | DZnPc-APDI-COF | Linkage-oriented | [38] |
7 | DTP-ANDI-COF | Linkage-oriented | [20] |
8 | PI-COF-3 | Linkage-oriented | [21] |
9 | Ttba-TPDA-COF | Linkage-oriented | [23] |
10 | COF-122 | Linkage-oriented | [24] |
11 | PP-TAB | Fragment-oriented | [29] |
12 | mPP-TAB | Fragment-oriented | [29] |
13 | dPP-TAB | Fragment-oriented | [29] |
14 | PC-COF | Fragment-oriented | [22] |
15 | PP-TAPB | Fragment-oriented | [29] |
16 | mPP-TAPB | Fragment-oriented | [29] |
17 | dPP-TAPB | Fragment-oriented | [29] |
18 | TDCOF-1 | Fragment-oriented | [41] |
19 | TDCOF-2 | Fragment-oriented | [41] |
20 | TDCOF-3 | Fragment-oriented | [41] |
OVERVIEW OF 3D COFS WITH LARGE PORES
Compared to 2D COFs with layered structures, the construction of 3D COFs with regular, periodic structures is proven to be a more complicated process. In particular, 3D COFs are generally less stable than 2D layered architectures due to more cavities and the lack of π-π stackings. Furthermore, 3D COFs tend to form cage-like structures when extended in three dimensions, and monomers can continue to react and grow outward in the cavity, which makes it easy for 3D COFs to generate multiple interspersed structures and more prone to collapse and shrinkage. Notably, the interpenetration between pores that occurs in diamondoid (dia) or pts topologies unfortunately leads to a high degree of channel constriction[42]. Taking these facts into account, current strategies for constructing 3D large-pore COFs mainly include (A) synthesizing monomers with side chains to prevent the collapse of large pores and (B) synthesizing novel topologies and fragments to construct large-pore size structures.
In sharp contrast to 2D COFs, the development history of large-pore 3D COFs is relatively new. In 2020, Wang et al. reported the work of expanding the pore size of 3D COFs up to 2.7 nm and achieving a high surface area of 3,023 m2/g through steric hindrance engineering[43]. It is worth noting that all JUC-550, 551, and 552 have non-interpenetrating dia topology by introducing methyl and methoxy groups into the building blocks [Figure 9]. For 3D COFs, it appears easier to shrink the pores or collapse the layers. As shown in Figure 10, either the (A) unmodified or (B) partially modified monomers result in shrinkage or interpenetration of the cavities[43]. Only the pores of the 3D COF built with (C) all modified units can be well maintained after the guest removal. Given that 3D cage-cavity organic structures are more prone to collapse, steric hindrance and expanding monomeric fragments are not feasible in producing large-pore 3D COFs, although they work well for creating large-pore 2D COFs.
Figure 9. Synthesis routes of (A) JUC-550; (B) JUC-551; and (C) JUC-552. (i) 1,4-Dioxane, 120 °C and 3 days; (ii) Mesitylene, 120 °C and 3 days; (iii) Mesitylene, 120 °C and 3 days. Reproduced with permission from ref.[43]. Copyright © 2020, American Chemical Society. 3D: Three-dimensional.
Figure 10. Synthesis for 3D mesoporous COFs for three possible: (A) unmodified; (B) partially modified units; and (C) all modified units. Reproduced with permission from ref.[43]. Copyright © 2020, American Chemical Society. 3D: Three-dimensional; COFs: covalent organic frameworks.
Since the development of 3D COFs is relatively slow compared to 2D COFs and there are only two cases with pore sizes larger than 4.0 nm, we also include and discuss 3D COFs with pore sizes larger than 3.0 nm in this section.
Topology-oriented synthetic strategy
In 2007, the first 3D boronate ester-linked COF-108 was reported by El-Kaderi et al., which exhibited boracite (bor) topology with a pore size of 3.0 nm[6]. In 2022, the first series of imine-linked COFs with bor topology were reported by Li et al.[44]. All three developed 3D-bor-COFs possess large pore sizes, i.e., 3.1 nm for 3D-bor-COF-1, 3.6 nm for 3D-bor-COF-2, and 3.8 nm for 3D-bor-COF-3 [Figure 11]. The obtained 3D COFs are excellent materials for capturing benzene vapor with adsorption capacities of 983.3 mg/g for 3D-bor-COF-1, 953.5 mg/g for 3D-bor-COF-2 and 1,203.9 mg/g for 3D-bor-COF-3.
Figure 11. Synthesis routes of (A) 3D-bor-COF 1; (B) 3D-bor-COF 2; (C) 3D-bor-COF 3; (i) o-DCB, HAc (6 M), 120 °C and 4 days; (ii)
To date, only three COFs with bor topology have been reported, and all of them possess high specific surface areas and excellent crystallinity.
Fragment-oriented synthetic strategy
Recently, the exploration of specific fragments has also been considered a promising strategy for creating large-pore 3D COFs. For example, in 2020, Li et al. reported the synthesis of JUC-564 with an unprecedented stp topology, a pore size of 4.3 nm, and a large BET surface area of 3,383 m2/g [Figure 12][45]. The JUC-564 was synthesized through the condensation of specific 2,3,6,7,14,15-hexa
Figure 12. Synthesis route of JUC-564. (i) Mesitylene/1,4-Dioxane = 3/2 (v/v), HAc (6 M), 120 °C and 3 days. Reproduced with permission from ref.[45]. Copyright © 2020, American Chemical Society. HFPTP: 2,3,6,7,14,15-hexa(4′-formylphenyl)triptycene; TAPPy: 1,3,6,8-tetrakis(4-aminophenyl) pyrene.
On this basis, Ding et al. further reported another series of JUC-COFs using HFPTP as one of the monomers[46]. Notably, JUC-640-H achieved a record large pore size of 4.6 nm in 3D COFs with a surface area of 2,204 m2/g, which abundantly exposed porphyrin moieties (0.845 mmol/g) [Figure 13]. The
Figure 13. Synthesis scheme of JUC-640-M. (i) o-DCB/n-BuOH = 1/1, HAc (6 M), 120 °C and 7 days. Reproduced with permission from ref.[46]. Copyright © 2023, American Chemical Society. HFPTP: 2,3,6,7,14,15-hexa(4′-formylphenyl)triptycene; DCB: dichlorobenzene.
Since the development of 3D COFs is relatively new, synthetic strategies for 3D large-pore COFs rely on exploring novel topologies and specific fragments, as shown in Tables 3 and 4. However, both methods confront bottlenecks. For instance, it has been reported that only HFPTP can form large pores and react with other building blocks with distinct symmetries. The topologies of large pore apertures are limited to bor and stp, which requires further efforts to develop more topologies to build large pore-sized 3D COFs. Notably, because cage cavity structures are more prone to shrinkage, most works adopted immersion powder for activation, and some of them even utilized supercritical carbon dioxide during the post-treatment of COFs.
Summary of the porosity of the 3D COFs mentioned above
CONCLUSION AND OUTLOOK
Similar to other porous materials, the structural characteristics and application performance of COFs are highly dependent on their porosity, especially pore size. In particular, COFs with large mesopores enable the entry of unique large-sized guest molecules, which undoubtedly expands their functionalities. Therefore, it is crucial to develop efficient approaches to design and synthesize large pore-sized COFs rationally. In this work, we review several state-of-the-art strategies for producing large-pore COFs shown in Tables 2 and 4, including (A) designing COFs with specific topologies, such as hcb, kgm, stp, bor, etc.; (B) using imide linkage to prepare target COFs; (C) employing sizeable molecular building blocks with charged part or side chains to prevent shrinkage of large-pore and interlayer slippage; and (D) developing organic unit with unique steric configurations to maintain large pores in 3D COFs. We call upon the fact that despite significant progress in this appealing research area, the synthetic mechanisms remain elusive. Further efforts combining molecular-level simulations and in situ structural characterization may provide a microscopic view of the large-pore formation.
As discussed above, most reported preparations of large pore-sized COFs still rely on simply increasing the length of the building blocks, but this results in low overall reaction yields and a tendency for structural collapse or interlayer interpenetration. Although several fragments with specific steric configurations can yield large pore-sized 3D COFs, the intrinsic relationship between structure and pore size is still unclear. In addition, all COFs with large pores reported to date are prepared using organic units with imine or boronic ester linkages, which also limits stability and subsequent functional exploration. Developing large-pore COFs with other linkages remains a challenge and requires further efforts.
Regardless of 2D COFs or 3D COFs, the pore collapse and blockage of the frameworks due to the extended length and high flexibility cannot be evaded. Therefore, an effective way needs to be proposed to retain the large porous networks. For instance, multi-bond linked COFs can be designed with relatively small pores and robust skeletons. The large-pore COFs can be generated by dissociating one or partial bonds under reverse conditions. Besides, there is an urgent need to explore a facile substituent that can effectively control the interlayer slip of 2D COFs to maintain large pores. As mentioned above, either AB or ABC stacking will lead to a reduction in pore size. Thus, it is crucial to synthesize available groups to construct building blocks that ensure AA stacking. Lastly, a potential template-assisted strategy can be proposed and used to support pores from collapse during post-treatment. Afterward, the templates were completely removed under mild conditions.
We emphasize that an ideal and effective strategy for designing large pore-sized COFs should not only produce regular porous structures with good structural stability but also have simple synthetic processes, high total reaction yields, and great generality. Such an efficient synthetic approach needs to be proposed and developed in the future. It is hoped that the continuous development of large mesoporous COFs will extend and promote their applications in more specific fields, including but not limited to the absorption and separation of macromolecules, bio-catalysis, etc.
DECLARATIONS
Acknowledgments
Jin E acknowledges the support from the start-up grant of Jilin University.
Authors’ contributions
Prepared and revised the manuscript: Li X
Revised the manuscript: Geng K, Fu S
Designed and revised the manuscript: Jin E
All authors contributed to the discussion and preparation of the manuscript.
Availability of data and materials
Not applicable.
Financial support and sponsorship
This work was financially supported by the National Natural Science Foundation of China (22288101, 22201092) and the 111 Project (B17020).
Conflicts of interest
All authors declared that there are no conflicts of interest.
Ethical approval and consent to participate
Not applicable.
Consent for publication
Not applicable.
Copyright
© The Author(s) 2024.
REFERENCES
1. Alsbaiee A, Smith BJ, Xiao L, Ling Y, Helbling DE, Dichtel WR. Rapid removal of organic micropollutants from water by a porous β-cyclodextrin polymer. Nature 2016;529:190-4.
2. Zheng B, Lin X, Zhang X, Wu D, Matyjaszewski K. Emerging functional porous polymeric and carbonaceous materials for environmental treatment and energy storage. Adv Funct Mater 2020;30:1907006.
3. Huang L, Yang J, Zhao Y, et al. Monolithic covalent organic frameworks with hierarchical architecture: attractive platform for contaminant remediation. Chem Mater 2023;35:2661-82.
4. Yuan Y, Yang Y, Meihaus KR, et al. Selective scandium ion capture through coordination templating in a covalent organic framework. Nat Chem 2023;15:1599-606.
5. Côté AP, Benin AI, Ockwig NW, O’Keeffe M, Matzger AJ, Yaghi OM. Porous, crystalline, covalent organic frameworks. Science 2005;310:1166-70.
6. El-Kaderi HM, Hunt JR, Mendoza-Cortés JL, et al. Designed synthesis of 3D covalent organic frameworks. Science 2007;316:268-72.
7. Zhuang X, Zhao W, Zhang F, et al. A two-dimensional conjugated polymer framework with fully sp2-bonded carbon skeleton. Polym Chem 2016;7:4176-81.
8. Jin E, Asada M, Xu Q, et al. Two-dimensional sp2 carbon-conjugated covalent organic frameworks. Science 2017;357:673-6.
9. Ma T, Kapustin EA, Yin SX, et al. Single-crystal x-ray diffraction structures of covalent organic frameworks. Science 2018;361:48-52.
11. Ding SY, Wang W. Covalent organic frameworks (COFs): from design to applications. Chem Soc Rev 2013;42:548-68.
12. Sun C, Sheng D, Wang B, Feng X. Covalent organic frameworks for extracting water from air. Angew Chem Int Ed Engl 2023;62:e202303378.
13. Burke DW, Jiang Z, Livingston AG, Dichtel WR. 2D covalent organic framework membranes for liquid-phase molecular separations: state of the field, common pitfalls, and future opportunities. Adv Mater 2024;36:e2300525.
14. Liang RR, Jiang SY, A RH, Zhao X. Two-dimensional covalent organic frameworks with hierarchical porosity. Chem Soc Rev 2020;49:3920-51.
15. Huang N, Wang P, Jiang D. Covalent organic frameworks: a materials platform for structural and functional designs. Nat Rev Mater 2016;1:16068.
16. Diercks CS, Yaghi OM. The atom, the molecule, and the covalent organic framework. Science 2017;355:eaal1585.
17. Chen X, Geng K, Liu R, et al. Covalent organic frameworks: chemical approaches to designer structures and built-in functions. Angew Chem Int Ed Engl 2020;59:5050-91.
18. Kandambeth S, Dey K, Banerjee R. Covalent organic frameworks: chemistry beyond the structure. J Am Chem Soc 2019;141:1807-22.
19. Jin S, Furukawa K, Addicoat M, et al. Large pore donor-acceptor covalent organic frameworks. Chem Sci 2013;4:4505-11.
20. Xu F, Jin S, Zhong H, et al. Electrochemically active, crystalline, mesoporous covalent organic frameworks on carbon nanotubes for synergistic lithium-ion battery energy storage. Sci Rep 2015;5:8225.
21. Fang Q, Zhuang Z, Gu S, et al. Designed synthesis of large-pore crystalline polyimide covalent organic frameworks. Nat Commun 2014;5:4503.
22. Yu SB, Lyu H, Tian J, et al. A polycationic covalent organic framework: a robust adsorbent for anionic dye pollutants. Polym Chem 2016;7:3392-7.
23. Xu T, An S, Peng C, Hu J, Liu H. Construction of large-pore crystalline covalent organic framework as high-performance adsorbent for rhodamine B dye removal. Ind Eng Chem Res 2020;59:8315-22.
24. Zhao C, Lyu H, Ji Z, Zhu C, Yaghi OM. Ester-linked crystalline covalent organic frameworks. J Am Chem Soc 2020;142:14450-4.
25. Vyas VS, Vishwakarma M, Moudrakovski I, et al. Exploiting noncovalent interactions in an imine-based covalent organic framework for quercetin delivery. Adv Mater 2016;28:8749-54.
26. Liu S, Hu C, Liu Y, Zhao X, Pang M, Lin J. One-pot synthesis of DOX@covalent organic framework with enhanced chemotherapeutic efficacy. Chemistry 2019;25:4315-9.
27. Zhang G, Li X, Liao Q, et al. Water-dispersible PEG-curcumin/amine-functionalized covalent organic framework nanocomposites as smart carriers for in vivo drug delivery. Nat Commun 2018;9:2785.
28. Zhao X, Pachfule P, Li S, et al. Macro/microporous covalent organic frameworks for efficient electrocatalysis. J Am Chem Soc 2019;141:6623-30.
29. Emmerling ST, Schuldt R, Bette S, et al. Interlayer interactions as design tool for large-pore COFs. J Am Chem Soc 2021;143:15711-22.
30. Qian C, Wu H, Teo WL, Liao Y, Zhao Y. Single-crystalline covalent organic frameworks. Trends Chem 2023;5:853-67.
31. Huang L, Yang J, Asakura Y, Shuai Q, Yamauchi Y. Nanoarchitectonics of hollow covalent organic frameworks: synthesis and applications. ACS Nano 2023;17:8918-34.
32. Qian C, Teo WL, Gao Q, Wu H, Liao Y, Zhao Y. Polycrystalline covalent organic frameworks. Mater Today 2023;71:91-107.
33. Lukose B, Kuc A, Frenzel J, Heine T. On the reticular construction concept of covalent organic frameworks. Beilstein J Nanotechnol 2010;1:60-70.
34. Lukose B, Kuc A, Heine T. The structure of layered covalent-organic frameworks. Chemistry 2011;17:2388-92.
35. Feng X, Dong Y, Jiang D. Star-shaped two-dimensional covalent organic frameworks. CrystEngComm 2013;15:1508-11.
36. Zhou TY, Xu SQ, Wen Q, Pang ZF, Zhao X. One-step construction of two different kinds of pores in a 2D covalent organic framework. J Am Chem Soc 2014;136:15885-8.
37. Crowe JW, Baldwin LA, McGrier PL. Luminescent covalent organic frameworks containing a homogeneous and heterogeneous distribution of dehydrobenzoannulene vertex units. J Am Chem Soc 2016;138:10120-3.
38. Jin S, Supur M, Addicoat M, et al. Creation of superheterojunction polymers via direct polycondensation: segregated and bicontinuous donor-acceptor π-columnar arrays in covalent organic frameworks for long-lived charge separation. J Am Chem Soc 2015;137:7817-27.
39. Su Y, Yuan G, Hu J, et al. Recent progress in strategies for preparation of metal-organic frameworks and their hybrids with different dimensions. Chem Synth 2023;3:1.
40. Cho HS, Deng H, Miyasaka K, et al. Extra adsorption and adsorbate superlattice formation in metal-organic frameworks. Nature 2015;527:503-7.
41. Mu Z, Zhu Y, Li B, Dong A, Wang B, Feng X. Covalent organic frameworks with record pore apertures. J Am Chem Soc 2022;144:5145-54.
42. Guan X, Chen F, Fang Q, Qiu S. Design and applications of three dimensional covalent organic frameworks. Chem Soc Rev 2020;49:1357-84.
43. Wang Y, Liu Y, Li H, et al. Three-dimensional mesoporous covalent organic frameworks through steric hindrance engineering. J Am Chem Soc 2020;142:3736-41.
44. Li Z, Hsueh C, Tang Z, et al. Rational design of imine-linked three-dimensional mesoporous covalent organic frameworks with bor topology. SusMat 2022;2:197-205.
45. Li H, Ding J, Guan X, et al. Three-dimensional large-pore covalent organic framework with stp topology. J Am Chem Soc 2020;142:13334-8.
Cite This Article
How to Cite
Download Citation
Export Citation File:
Type of Import
Tips on Downloading Citation
Citation Manager File Format
Type of Import
Direct Import: When the Direct Import option is selected (the default state), a dialogue box will give you the option to Save or Open the downloaded citation data. Choosing Open will either launch your citation manager or give you a choice of applications with which to use the metadata. The Save option saves the file locally for later use.
Indirect Import: When the Indirect Import option is selected, the metadata is displayed and may be copied and pasted as needed.
About This Article
Special Issue
Copyright
Author Biographies
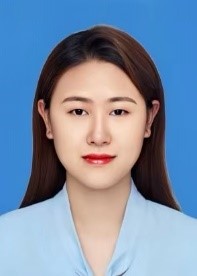
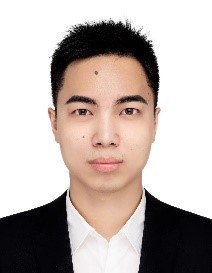
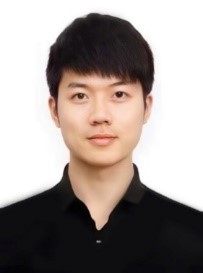
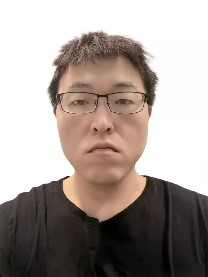
Comments
Comments must be written in English. Spam, offensive content, impersonation, and private information will not be permitted. If any comment is reported and identified as inappropriate content by OAE staff, the comment will be removed without notice. If you have any queries or need any help, please contact us at [email protected].