The tumor microenvironment in hepatocarcinoma: dissecting the functions of cancer-associated fibroblasts
Abstract
Hepatocellular carcinoma (HCC) is the most common primary liver malignancy, deriving from the neoplastic transformation of hepatocytes, most often forced to long-lasting regeneration by the cirrhotic background. HCC is an extremely aggressive tumor with still limited effective treatments, and is characterized by the presence of a very complex and multifaceted tumor microenvironment (TME). Among the variety of cell types populating the TME of HCC, cancer-associated fibroblasts (CAFs) are the most prevalent. CAFs are a specific population of fibroblasts in a persistent state of activation, with a high level of heterogeneity, partly dependent on a wide range of cell origin, which are endowed with a repertoire of functions, profoundly modulating the biology of the tumor. Given the close relationship of HCC with cirrhosis, CAFs are paradigmatic of the role played by activated fibroblasts in promoting the evolution of a chronic, non-resolving, fibro-inflammatory condition towards a neoplastic disease and its aggressive phenotype. In this review, we will discuss the most recent findings regarding the interplay of CAFs with the tumoral epithelial compartment, with the multiple cell elements of the TME (macrophages, neutrophils, myeloid-derived suppressor cells, vascular cells), and with the extracellular matrix. Finally, we will address the translational value of CAF manipulation in HCC to unveil possible ameliorations for the treatment of a still worrisome disease.
Keywords
INTRODUCTION
The functional hallmark of progressive hepatic fibrosis evolving to cirrhosis, which represents the common disease background in the majority of hepatocellular carcinoma (HCC), is represented by the activation, proliferation, and accumulation of fibroblasts. This feature could be, in fact, found in more than 80% of cases of HCC[1]. Among the different etiologies of chronic liver disease with extensive fibrosis, inappropriate alcohol consumption and steatotic liver disease associated with the metabolic syndrome (metabolic dysfunction-associated steatotic liver disease, MASLD) are becoming the most frequent in Western countries[2,3]. These metabolic etiologies are responsible for the continuous growth of incidence of HCC reported in the last decade, though the success of vaccination programs and direct antiviral agents has reduced the occurrence of HCC caused by viral infections (hepatitis B virus, HBV and hepatitis C virus, HCV)[1].
As a prototype of epithelial cancer related to fibrosis, HCC serves as a good model to dissect the intricate role of fibroblasts, the main cell effector of fibrosis in the liver, from tumorigenesis to tumor progression. When activated fibroblasts (myofibroblasts) accumulate in a setting, switching from persistent inflammation to a malignant state, they are termed cancer-associated fibroblasts (CAFs)[4]. In HCC, CAFs are the preponderant cell type hosted in a multicellular “ecosystem” called tumor microenvironment (TME), which regulates a web of interactions of the tumoral component with different stromal cell elements, including immune and vascular cells, surrounded by a perturbed extracellular matrix (ECM)[5,6]. Several lines of evidence support CAF’s ability to influence HCC progression in a multifaceted manner, not only acting on the tumoral cells themselves, but also actively contributing to shaping the TME composition and structure. In fact, by secreting a wide range of soluble factors (cytokines, chemokines, hormones, growth factors), along with exosomes and extracellular vesicles (EVs), CAFs exert both direct and indirect effects on tumoral hepatocytes, and on tumor growth and invasion, respectively. These effects are mediated by ECM remodeling and the recruitment and modification of the biological functions of other cellular elements of the TME[7,8]. Given the close relationship of HCC with cirrhosis, it is tempting to envisage a key role of fibroblasts in promoting the evolution of a chronic, non-resolving inflammatory condition towards a neoplastic disease and its aggressive phenotype. The aim of this review is to provide the reader with updates on the complex role of CAFs in HCC with the aim to unveil novel potential therapeutic interventions in a disease yet penalized by very limited opportunities for effective treatments.
COMPOSITION OF THE TUMOR MICROENVIRONMENT IN HCC: CAFS ARE ACCOMPANIED BY IMMUNE AND NON-IMMUNE CELLS
In HCC, CAFs populate the TME in association with a wide range of different cell types, which are organized into both immune and non-immune compartments. In healthy conditions, the liver contains a large number of immune cell elements, which generate a milieu conducive to tolerance towards the gut-derived microbial components to preserve the homeostatic balance[1]. In the malignant setting, both innate and adaptive immune cells are recruited and acquire variable activation states within the TME. Thanks to the advent of single-cell technologies, it has become clear that in HCC, TME is highly heterogeneous, resulting in a diversity of cell communications that variably sustain tumor invasion[9]. Neutrophils, monocytes, macrophages (both monocyte-derived and tissue-resident), dendritic cells, myeloid-derived suppressor cells (MDSC), natural killer (NK) cells, T and B cells infiltrate in the TME and may exert either immune suppressive or antitumor effects. In particular, MDSC, tumor-associated macrophages (TAM), and regulatory T (Treg) cells sustain immune suppressive activities, and their increased number correlates with tumor progression in HCC[10]. Conversely, the cytotoxic cluster of differentiation (CD)8+ and CD4+ T cells displays a pro-inflammatory/Type 1 T helper (Th1) phenotype, and NK cells induce stimulatory immune functions, including surveillance, identification, and killing of tumor cells. Their presence in the TME warrants an effective immune response to cancer[10]. Recently, the generation of a
Within the non-immune compartment, endothelial cells (ECs) represent the most important element. Indeed, hypervascularization is a hallmark of HCC and serves as a well-established criterion of early detection in imaging studies. Besides actively contributing to angiogenesis, ECs collaborate in procoagulatory and fibrinolytic mechanisms, which are instrumental to tumor progression by favoring vascular invasion. Of note, portal vein thrombosis is a common finding in advanced HCC and a limitation to curative approaches[12]. Platelets are another important cell type deserving attention in the TME and have been reported to act as regulators of tumorigenesis in HCC[13]. Platelet cargo, adhesion and activation but not platelet aggregation were identified as pivotal for hepatocarcinogenesis in MASLD; once recruited to the tumor lesion by Kupffer cells, they affected intrahepatic immune cell trafficking[13].
In the following chapters, we will focus on the functional interactions undertaken by CAFs within the TME, whereas the reader may refer elsewhere for studies reviewing the immune and vascular functions of TME in HCC[14,15]. As a prerequisite for better understanding the wide CAF pleiotropy, we will first highlight the concept that CAFs may originate from several different cell types and, therefore, exhibit a range of activation states, resulting in a high level of heterogeneity that variably affects cancer development. Then, after discussing the crosstalk of CAFs with the tumoral epithelial compartment, we will illustrate the CAF interplay with each cell component of the TME, and the effects of CAFs on the ECM. Finally, we will address the translational value of CAF manipulation in HCC.
CAF HETEROGENEITY
Although historically, CAFs have been regarded as a rather homogeneous population of constitutively activated myofibroblasts, mounting data have demonstrated that instead, they are very heterogeneous in terms of both cell origin and cell differentiation, leading to an extremely plastic and diversified system whose multiple functions heavily influence the malignant behavior of the tumor. In the liver, the main cell sources from which CAFs can originate are hepatic stellate cells (HSCs), portal fibroblasts (PFs), bone marrow (BM)-derived cells (including fibrocytes and mesenchymal stromal cells), and cells undergoing epithelial-to-mesenchymal transition (EMT) [Figure 1][16].
Figure 1. Heterogeneity of CAFs in HCC. CAFs dwelling in the TME of HCC can originate from different cell sources, including HSCs, PFs, and bone marrow-derived cells (both fibrocytes and MSCs) or from epithelial structures undergoing EMT. These cell types, upon inflammatory mediators, can activate or transdifferentiate into CAFs. Recent scRNA-seq experiments have demonstrated that the TME of HCC contains different CAF subpopulations with different genetic signatures and biological properties that can help predict the patient outcome. Using different scRNA-seq approaches, two different groups have been identified: (A) 4 (CAF_0, _1, _2, _3)[17] and (B) 3 (CAF_Ports, _VSMC, _HSC)[18], respectively, in two different cohorts of HCC. CAF: cancer-associated fibroblast; HCC: hepatocellular carcinoma; TME: tumor microenvironment; HSC: hepatic stellate cell; PF: portal fibroblast; MSC: mesenchymal stem cells; EMT: epithelial-mesenchymal transition; Ports: portal; VSMC: vascular smooth muscle cells; scRNA-seq: single-cell RNA sequencing; α-SMA: alpha-smooth muscle actin; FAP-α: fibroblast activation protein-alpha; NTPD2: nucleoside triphosphate diphosphohydrolase-2; IL: interleukin; CD: cluster of differentiation; MHC II: major histocompatibility complex; c-Myc: cellular Myelocytomatosis; FGFR1: fibroblast growth factor receptor 1; MMP2: metalloproteinase 2; CXCL12: C-X-C motif chemokine 12; VEGF: vascular endothelial growth factor; TnC: Tenascin C. Figure partially made using biorender.com.
Hepatic stellate cells
Several papers have identified HSCs as the cell population mainly contributing to the generation of CAFs. HSCs are cells of mesenchymal origin, generally quiescent, which reside in the space of Disse, in close contact with the liver sinusoids. Several stimuli are able to induce HSC activation and their differentiation into myofibroblasts or CAFs. Myofibroblasts and CAFs are characterized by the expression of typical markers such as alpha-smooth muscle actin (α-SMA) and fibroblast activation protein-alpha (FAP-α)[19]. Once activated, CAFs actively secrete ECM proteins, such as collagens, and mediators that act in a paracrine fashion on other cells of the TME, as we will discuss later. Several factors released in the TME of HCC induce CAF activation, such as interleukin (IL)-1 and platelet-derived growth factor (PDGF)-β, together with oxidative stress[20]. Interestingly, the HCC cells themselves may also influence CAF differentiation by secreting exosomes containing microRNA (miRNA)-21, which activates the Phosphatase and TENsin homolog deleted (PTEN)/3-phosphoinositide-dependent protein kinase-1 (PDK1)/AKT pathway. Once activated, CAFs initiate a proangiogenic process by secreting vascular endothelial growth factor (VEGF)-A, metalloproteinase (MMP)-2, MMP-9, basic fibroblast growth factor (bFGF), and transforming growth factor (TGF)-α and -β, essential for tumor survival, growth, and metastatisation[21].
Notably, several fate tracing-based studies have demonstrated that HSCs are the cell population that is mainly involved in sustaining hepatic fibrogenesis following liver injury. A pioneering study by
Portal fibroblasts
PFs are mesenchymal cells lying within the portal space, whereby they form a thin cell rim surrounding the portal vasculature (portal vein and hepatic artery) and the bile ducts. The importance of PFs as a source of CAFs in HCC is controversial, though some studies have shown their major contribution to myofibroblasts in chronic liver diseases[25]. PFs can be distinguished from HSCs by the absence of lipid droplets and vitamin-A[26,27] and by the expression of specific phenotypic markers, such as ecto-ATPase nucleoside triphosphate diphosphohydrolase-2 (NTPD2), elastin, fibulin-2, IL-6, mucin-16, and Thy1[26]. Once activated by mediators such as taurocholic acid and IL-25[27], similarly to what happens for HSCs, PFs start to express α-SMA and to secrete ECM proteins, such as collagen-1α1 and profibrotic mediators such as IL-13, typically promoting liver fibrogenesis in chronic damage[26,27]. Notably, in a cholestatic mouse model (BDL mice), the percentage of PF-derived myofibroblasts exceeded 70% at 5 weeks after surgery[27]. An elegant paper using fate-tracing analysis in multidrug resistance (Mdr2)-/- mice, an accepted model of primary sclerosing cholangitis, expressing a collagen-green fluorescent protein (GFP) reporter, demonstrated that the percentage of Thy1+ PFs increases over time, from 26% at 4 weeks to 56% at 16 weeks of age[28]. Finally, though reaffirming the strong support of HSCs in sustaining fibrogenesis in hepatotoxic models, such as after CCl4 intoxication, a recent scRNA-seq work demonstrated that this is mainly true in the advanced stages of fibrosis, while PFs play a more relevant role in the earlier stages[29].
Bone marrow-derived cells
Besides HSC and PF, myofibroblasts can also originate from two bone marrow-derived populations, fibrocytes and mesenchymal stem cells (MSCs). Data regarding the role of fibrocytes in the liver are actually rather scarce. These cells originate from hematopoietic stem cells and express hematopoietic markers, such as CD45, CD34, CD11b, major histocompatibility complex (MHC) II, and Ly-6G, together with typical fibroblast markers, such as vimentin, fibronectin (FN), and collagen-I[16]. Fibrocytes, once recruited at the site of liver damage, upon TGF-β1 stimulation, become activated, increase the expression of α-SMA and the secretion of collagen-1, and simultaneously decrease the expression of hematopoietic markers[30,31]. These cells may represent varying percentages of the matrix-secreting cells, ranging, in mouse models, from 3% to 50%[31]. However, these studies are in stark contrast to more recent works taking advantage of in-vivo cell tracing systems, which show that fibrocytes sparingly participate in fibrosis deposition[25,27]. These conflicting results call into question the real pathogenic value of this cell population, and claim the need for further investigations. MSCs are multipotent cells that can differentiate into a large number of cell types, including adipocytes, cartilage cells, as well as CAFs. These cells, once recruited to the site of damage by HCC cells, stimulate tumor initiation and progression[32]. Recent studies have highlighted the close interactions of MSC with the TME in their transdifferentiation into CAF-like cells, featuring expression of α-SMA, vimentin, cellular myelocytomatosis (c-Myc), MMP-2, VEGF, IL-6, fibroblast growth factor receptor 1 (FGFR1), IL-8, C-X-C motif chemokine 12 (CXCL12), and tenascin-C (TnC), under the regulation of miR17-5P and
Epithelial-mesenchymal transition
EMT is a pathophysiological process in which epithelial cells reduce or lose the expression of typical epithelial markers, such as cytokeratin (K)-8 and -18, E-cadherin, and epithelial cellular adhesion molecule (EpCAM), in favor of mesenchymal phenotypic markers, such as vimentin, zinc finger protein SNAI1 (SNAIL), Twist, and α-SMA. In addition to these phenotypic changes, cells undergo functional changes, such as the loss of cell polarization and the acquisition of motile and invasive properties[34]. Three types of EMT (types 1, 2, and 3) have been categorized and are involved in different physiological or pathophysiological responses[35]. EMT type 1 is involved in fetal development and is responsible for the generation of fetal mesenchymal structures starting from the primordial epithelium[36]. EMT type 2 takes part in organ repair/regeneration mechanisms. During these processes, under the influence of various mediators, such as TGFβ, PDGF, epithelial growth factor (EGF), FGF-2, and MMPs, wounded epithelial cells transdifferentiate from a mature phenotype to mesenchymal-like cells losing typical epithelial marchers, such as E-cadherin, EpCAM, cytokeratins and zonula occludens-1 (ZO-1), to acquire in turn a mesenchymal phenotype, characterized by the ex novo expression of vimentin, FN, N-cadherin, SNAIL, Twist, S100A4 and others[37]. Concomitantly, these cells gain the ability to dismantle the basal lamina, and to secrete profibrotic mediators or ECM proteins different from the physiologically represented laminin and collagen-IV. Finally, EMT type 3 occurs in tumorigenesis and metastasis. The activation of an EMT program is found in a large variety of tumors, including breast, lung, colon, and liver[38]. Due to EMT, epithelial cells acquire mesenchymal-like phenotypic and biological properties that allow transdifferentiated neoplastic cells not only to respond to the trophic effect of the cell populations present in the TME, but also to acquire motile and invasive properties, thereby favoring tumor metastasis[35,38]. Although several studies indicate that overexpression of EMT signatures in neoplastic cells correlates to a worse outcome for HCC patients[39,40], the data supporting a full EMT conversion are, so far, actually conflicting. In fact, while first lineage-tracing studies in CCl4-treated AlbCre;R26RstoplacZ double-transgenic mice demonstrated that up to 40% of FSP-1+ myofibroblasts derived from tumor hepatocytes[41], following studies using triple transgenic mice expressing ROSA26 stop beta-galactosidase (beta-gal), albumin Cre, and collagen α(I)-GFP or Alfp-Cre × Rosa26-yellow fluorescent protein (YFP) mice treated with BDL, CCl4, and DDC, failed to confirm this observation[42,43].
CAF subpopulations in HCC
Recent scRNA-seq-based studies have addressed the presence of several subpopulations of CAFs with markedly different immunophenotypic traits within the TME of HCC [Figure 1]. Taking this approach,
Notably, recent scRNA-seq studies performed in pancreatic ductal adenocarcinoma[44,45] and in lung cancer[46] have identified a further CAF subpopulation, the antigen-presenting CAF (apCAF). This CAF subtype, unlike other CAF subsets that exert protumorigenic effects, seems to counteract tumor progression. By expressing MHC class II and CD74, apCAFs activate CD4+ T cells in an antigen-specific manner, leading to a significant tumor-suppressive effect. Therefore, the identification of apCAFs in HCC might further dissect the pleiotropic functions of CAFs in its complex TME.
Although these data are preliminary and require further validation, overall, they suggest the idea that CAFs are central in sustaining the pathogenesis and shaping the aggressiveness of HCC, regardless of their cellular origin. To address this concept, we will dissect the diverse ability of CAFs to engage in intimate cross-talking with the tumor compartment and with the multiple components of the TME (vascular structures, immune cells, and ECM) in HCC. These interactions are mediated by a broad spectrum of signals, mutually exchanged, and include cyto- and chemokines, growth factors, morphogens, matrix remodeling enzymes, and vesicles.
CAF INTERACTIONS WITH THE TUMORAL HEPATOCYTES: EFFECTS ON STEMNESS AND METABOLIC REPROGRAMMING
The propensity of CAFs to interact with tumor hepatocytes has been demonstrated in different stages of malignant transformation, involving either tumor initiation or tumor progression. The gain of stem cell-like properties is a key feature of tumorigenesis, but is also relevant for tumor progression by promoting chemoresistance, metastatization, and recurrence. Several studies highlight the concept that CAFs can promote a highly plastic, stem cell-like niche of cancer cells (cancer stem cells, CSC) in HCC cells, actively contributing to tumor growth and invasion by secreting a range of soluble factors. The list of CAF-secreted factors stimulating pro-invasive functions in HCC cells and the relative activated pathways is summarized in Table 1.
List of soluble factors secreted by CAFs inducing pro-invasive phenotype in HCC
CAF-secreted factors | Signaling pathways | Effects on HCC cells | Ref |
HGF |
MET-STEM
ERK1/2-FRA1-HEY1 | Stem-like features | [ 47 ] |
IL-6 | STAT3/Notch | Stem-like features | [ 48 ] |
LSD1 | Notch3 | Stem-like features | [ 49 ] |
CLCF1 | CXCL6/TGF-β |
Stem-like features
Neutrophils recruitment | [ 50 ] |
STC1 | Notch1 | Stem-like features | [ 51 ] |
COMP/CD36 |
PI3K/AKT
MEK/ERK |
EMT-like features
HCC cell proliferation and metastatization | [ 52 ] |
CCL5 | HIF1α/ZEB1 |
EMT-like features
HCC cell metastatization | [ 53 ] |
CCL2,CCL5,CCL7,CXCL1,CXCL6 | Hh/TGF-β | HCC cell metastatization | [ 54 ] |
CXCL11 | circUBAP2/miR-4756/IFIT1 | HCC cell migration | [ 55 ] |
TGF-β | TGF-β/SMAD | HCC cell proliferation | [ 56 ] |
TGF-α | TGF-α/EGFR | Stem-like features | [ 57 ] |
Among these, some show unique mechanisms acting through intricate axis, which may create positive loops involving other cell types of the TME, and deserve detailed discussion to underline their utility as biomarkers or therapeutic targets. Cardiotrophin-like cytokine factor-1 (CLCF1) released by CAFs increased CXCL6 and TGF-β secretion by HCC cells acting on the ciliary neurotrophic factor receptor. CXCL6 and TGF-β have both autocrine and paracrine effects. Autocrinally, they drive tumor stemness, while paracrinally, they promote intratumoral infiltration and polarization of tumor-associated neutrophils (TAN) that induce tumor angiogenesis and further stimulate stemness. In turn, HCC cell-derived CXCL6 and TGF-β cooperatively enhance CAF expression of CLCF1 via ERK1/2, leading to a feedforward loop that sustains HCC aggressiveness[50]. The translational significance of this working model is supported by the correlation of CLCF1/CXCL6/TGF-β cascade with tumor stage and poor outcome in HCC, thus behaving as a putative prognostic biomarker[50]. It is also worth mentioning that TGF-α, another cytokine secreted by CAFs[58] and other cell types, including tumor hepatocytes[59] and macrophages[60], cooperate with TGF-β in promoting hepatocarcinogenesis. Using an in-vivo model of HCC, Baek et al.[61] suggest that the activation of the TGF-α-Ras-MAPK signaling coupled with TGF-β downregulation is responsible for the development of HCC through the loss of the Raf kinase inhibitor protein (RKIP), the decrease of p21, the increase of cyclin E levels. Notably, TGF-α, sharing the 35%-40% sequence homology and having a similar tridimensional structure to EGF, is able to bind EGFR, inducing a sustained proliferative response[62], increased angiogenesis[63] and acquisition of stem cell traits in HCC cells through an EGFR-mediated pathway[57].
Activation of Notch signaling is a hallmark of cancer stemness frequently found in HCC (30% of cases), and represents a target of several paracrine cues originating from CAFs (IL-6, lysine-specific demethylase (LSD1), stanniocalcin-1 (STC1)). A recent work provides evidence that STC1, a glycoprotein abnormally overexpressed by CAFs in a variety of cancers (colorectal, lung, and breast), activates Notch1 signaling to induce stemness of HCC cells, and Notch1 directly upregulates STC-1 expression in CAFs to unleash an amplifying circuit. Nonetheless, serum levels of STC1 correlate with the worst clinical outcome of HCC patients, suggesting it may have tumoral biomarker properties[51].
Stromal-derived cartilage oligomeric matrix protein (COMP), a soluble pentameric glycoprotein, originally described for its hepatic pro-fibrogenic actions, stimulates EMT and stemness features in HCC cells by interacting with CD36 to activate phosphatidylinositol 3-kinase (PI3K)/AKT and mitogen-activated protein kinase (MEK)/extracellular-signal-regulated kinase (ERK)[52]. Of note, COMP can be secreted by CAF, and this mechanism can be hampered in a paracrine manner by Resolvin D1 (RvD1), an endogenous anti-inflammatory lipid mediator derived from DHA upon lipoxygenase, which can be released by inflammatory cells in the TME of HCC. COMP-suppressing effects of RvD1 are mediated by formyl peptide receptor-2 (FPR2)/reactive oxygen species (ROS) to abrogate forkhead box protein M1 (FOXM1) recruitment to the COMP promoter. Thus, RvD1 holds potentialities for therapeutic intervention in HCC[64].
Interest in the metabolic interplay between tumors and the stromal reaction has been growing in the last few years. It is renowned that CAFs (playing in a catabolic state) and HCC cells (anabolic state) are reciprocally coupled by exchanging a range of metabolic signals, mediated by exosomes harboring miRNA, ROS and energy-rich metabolites, like lipids and amino acids. This functional interaction aims at providing tumor cells with nutrients to support their highly demanding needs, and thus, a subset of tumor cells with a closer spatial relationship with CAFs may take a selective advantage due to their pro-invasive capabilities. Perturbation of glycolysis in cancer cells is a paradigmatic example of this concept. A reciprocal metabolic reprogramming with lactate rather than glucose utilization in response to a decreased expression of the glucose transporter protein type 1 (GLUT-1) by cancer cells has been originally shown in breast cancer cells[65]. Then, it was confirmed in other tumor settings, leading to the concept of ‘metabolic asymmetry’, which enables cancer cells to proliferate and migrate in energy-deprivation conditions[66]. Activation of hypoxia-inducible factor 1 (HIF-1), typically found in HCC, may mediate this lactate shuttle[67]. Metabolic asymmetry with inhibition of mitochondrial oxidative phosphorylation and stimulation of both aerobic and anaerobic glycolysis has been described in HCC cells, and found to be regulated by CAF-derived chemokyne ligand (CCL) 20 secretion modulated by myeloid differentiation primary response 88 (MYD88) and by exosomes harboring amino acids, lipids and tricarboxylic acid[68,69]. However, compared to studies conducted in other cancer cell types (breast, ovarian), the ability of CAFs to regulate metabolism in HCC cells, including lipid and oxidative metabolism in addition to glucose metabolism, is less understood and represents an area ripe for further investigation.
CAF INTERACTIONS WITH THE COMPONENTS OF TME
Tumor immune microenvironment
TIME is a comprehensive definition that brings together all the immune cells within the TME, which are involved in the generation of a TIB in HCC[11]. Among them, TAMs, TANs, and MDSC are the most influenced by CAFs in HCC[70] [Figure 2].
Figure 2. Central role of CAFs involved in modification of the TME. By secreting a range of cytokines and chemokines, CAFs are able to recruit cells of the TIME in a direct (monocytes and MDSCs) and indirect (TAN) route. TAN are recruited around the tumor cells through the action of CAF-secreted CLCF1 that, in turn, induces the secretion of the chemotactic mediators CXCL5, CXCL6, and TGF-β by HCC cells. Besides monocyte recruitment, CAFs induce a M1-M2 switch of macrophages. Furthermore, CAFs are actively involved in angiogenic processes by secreting PGF, VEGF, and Ang-1. Finally, CAFs are able to modify the composition of the ECM surrounding tumor cells by secreting MMP9 and downregulating the expression of decorin. CAF: cancer-associated fibroblast; ϕ: macrophage; HCC: hepatocellular carcinoma; TAN: tumor-associated neutrophil; ECM: extracellular matrix; MDSC: myeloid-derived suppressor cells; Gas6: growth arrest-specific protein 6; PAI 1: plasminogen activator inhibitor 1; IL: interleukin; TGF-β: transforming growth factor β; CLCF1: cardiotrophin-like cytokine factor-1; MMP-9: metalloproteinase 9; CXCL12: C-X-C motif chemokine 12; CCL2: chemokine ligand 2; PGF: placental growth factor; VEGF: vascular endothelial growth factor; Ang-1: angiopoietin 1; EZH2: enhancer of zeste homolog-2; VASH1: vasohibin 1. Figure partially made using biorender.com.
Tumor-associated macrophages
TAMs are macrophages, typically M2-like phenotype, infiltrating different solid tumors (breast, pancreas, liver, and others) where they stimulate pro-invasive functions, such as proliferation, metastasis, tumoral angiogenesis, and chemoresistance[71,72]. Several studies have pointed out CAF capability not only to attract macrophages to the tumor site, but also to skew them from the M1/pro-inflammatory to the M2/pro-fibrogenic and cancer-related phenotype. Indeed, CAFs recruit monocytes from the bloodstream through several redundant pathways by activating the CXCL12/C-X-C Motif Chemokine Receptor 4 (CXCR4)[25,73] signaling pathway, and by overexpressing endosialin. By binding to its receptor CD68, endosialin induces the recruitment of macrophages[74]. Endosialin also promotes the M1-M2 shift by activating the growth arrest-specific protein 6 (GAS6) pathway[74]. In addition to Gas6, CAFs also overexpress the plasminogen activator inhibitor 1 (PAI 1), another mediator of the M1-M2 transdifferentiation[75]. Furthermore, this phenotypic shift can be induced by CAFs through the secretion of numerous other mediators, such as IL-6, IL-10, IL-13, and TGF-β[76,77]. Notably, the presence of M2 macrophages has a strong impact on several malignant features of HCC, such as cell proliferation, tendency to metastasis, and induction of stem-like signatures. Indeed, TAMs secrete a wide array of cyto- and chemokine mediators, such as IL-6[78], IL-8[79], CXCL8[80], and CCL2[78], which stimulate tumor cell invasiveness and migration, and IL-23, which stimulates tumor vascular invasion[81]. Furthermore, TAMs interact with osteopontin to promote the increase in CSC markers, such as SRY-box transcription factor 2 (Sox2) and octamer-binding transcription factor 4 (Oct4) through an integrin αvβ3-nuclear factor kappa B (NF-κB)-HIF-1α-dependent pathway[82].
Tumor-associated neutrophils
TANs are a subpopulation of pro-tumorigenic neutrophils whose presence inversely correlates with the survival of patients with HCC[72], partly related to their ability to exacerbate cancer cell invasiveness and chemoresistance, for example, to Sorafenib[83]. Although elucidating the role of TANs has become a theme of interest, data regarding the action of CAFs on TANs are, however, still limited. Recent data indicate that CAFs indirectly promote TAN recruitment to the tumor site. These effects are mediated by their secretion of CLCF1, which enables HCC cells to produce CXCL6 and TGF-β. These factors have a dual action: the active recruitment of neutrophils and their phenotypic switch from N1 (pro-inflammatory/anti-tumorigenic) to N2 (anti-inflammatory/pro-tumorigenic)[50]. Furthermore, TANs secrete high amounts of trophic mediators for HCC cells, such as CCL2 and CCL17[72], and induce the expression of miR-301b-3p by HCC cells, which regulate the NF-kB-mediated secretion of CXCL5. In turn, CXCL5 recruits to the tumor area and activates further TANs[84], locking them in a mechanically self-sustaining pro-invasive loop.
Myeloid-derived suppressor cells
MDSCs encompass a heterogeneous group of bone marrow-derived immune cells belonging to the myeloid lineage with a marked ability to suppress immunity, thereby displaying a tumor-favoring activity. CAFs are able to recruit MDSCs both through the secretion of interleukins, such as IL-6 and IL-33[85,86], and through the CCL2/C-C chemokine receptor type 2 (CCR2) axis[87]. MDSCs show strong immunosuppressive functions due to their capacity to dampen the cytotoxic activity of NK cells and enhance immune checkpoint signaling, along with the expression of PD-L1[88]. Notably, the inhibition of the CCL2 signaling pathway in Ccr2-defective mice disrupted MDSC recruitment and resulted in improved HCC outcomes in mice[89].
CAF interaction with vascular structures
The ability of CAFs to play an active role in promoting tumor-associated angiogenesis by recruiting vascular cells is well-established. This has been particularly demonstrated in cholangiocarcinoma, where CAFs have been observed to contribute to lymphangiogenesis[90]. A significant increase in the microvascular density (MVD) of blood vessels is typical of HCC[16], unlike what occurs, for example, in cholangiocarcinoma[91]. As aforementioned, several growth factors involved in tumor angiogenesis, including placental growth factor (PGF), Angiopoietin-1 (Ang-1), and VEGF, are secreted by CAFs. Huynh et al.[92] observed that after activation, mucosal fibroblasts overexpressed CD90 and a-SMA and transitioned into CAFs in several types of tumors. CD90 and a-SMA were found to be highly expressed and colocalized in the stroma of HCC tissues and isolated CAFs. Previous studies demonstrated a profound correlation between CD90 and PGF enriched-CAFs with tumor angiogenesis markers (CD31, CD34, and CD105), pointing to the role of CAFs in facilitating angiogenesis[93]. Notably, some studies demonstrated a strong association between CAFs and poor prognosis in several types of cancer, including HCC[94]. In-vitro studies performed by Liu et al. demonstrated that patients-derived CAFs and LX-2 cells both overexpressed PGF and PGF expression closely correlated with MVD in human HCC sections[93]. Furthermore, in a model of nude (nu/nu) mice xenotransplanted with human HCC cells and CAF, Lin et al. showed that silencing of Ang-1 in CAFs significantly reduced the MVD evaluated as CD34+ morphometry in the tumor area[95]. Furthermore, VEGF secretion by CAFs is a key mechanism of HCC neo-angiogenesis. Using both in-vivo and in-vitro models, Huang et al. demonstrated that CAF-derived VEGF increased the expression of enhancer of zeste homolog-2 (EZH2). Acting as a tumor suppressor gene by repressing the expression of vasohibin-1 (VASH1), EZH2, in turn, stimulated the proliferation of ECs. Conversely, inhibition of the VEGF/EZH2 axis led to up-regulation of VASH1, which decreased tumor-associated angiogenesis[96].
Besides tumor-associated angiogenesis, portal vein tumor thrombosis (PVTT) might also depend on CAFs’ abilities to interact with vascular structures and stimulate invasive properties of HCC cells, though studies devoted to this theme are hitherto lacking. PVTT is a common complication of HCC, mostly in advanced stages, and its occurrence limits its suitability for curative treatments. Moreover, the range of soluble factors released by CAFs in the TME can also be responsible for cancer-associated thrombosis (CAT), and in this respect, elucidation of underlying molecular mechanisms acting through chemokine-activated Hedgehog and TGF-β pathways may help to identify patients at a higher risk of thrombosis and select appropriate candidates for thromboprophylaxis[54]. Although the role of CAFs in CAT remains unclear, recent studies have shown that CAFs exacerbate CAT in several types of cancers[97]. In addition, an increased metastatic burden has been correlated in various cancer types with the expression of podoplanin (PDPN), a cell-surface mucin-like glycoprotein that is well conserved across species and serves a wide variety of functions. PDPN is upregulated in certain types of tumor cells, including CAFs, to promote cancer progression[98]. To exert its functions, PDPN utilizes other proteins, such as C-type lectin-like receptor-2 (CLEC-2), through which it induces platelet aggregation, and mediates the adhesion of cancer cells to platelets. Activated platelets, in turn, protect cancer cells in circulation and facilitate the extravasation of cancer cells at the metastatic niche. The exact mechanism by which PDPN induces thrombosis in cancer cells is not known. However, in the study by Sasano et al.[99], PDPN-expressing EVs released from PDPN+ ovarian cancer cells caused venous thrombosis more frequently than PDPN- EVs, and PDPN-expressing EVs resulted in thrombi with more platelets compared with thrombi induced by PDPN- EVs. Therefore, PDPN and CLEC-2 may be regarded as promising molecular links between CAFs and CAT, and the hypothesis that CAFs exacerbate CAT through the PDPN/CLEC-2 axis is gaining increasing consideration[98]. In the recent study conducted by Shirai et al. in a lung cancer mouse model[97], PDPN/CLEC-2 targeting was harnessed to develop an anti-CAT strategy. In this study, CAFs actually contribute to CAT by their massive PDPN expression induced by the TME. Interestingly, they observed that both direct cell-cell interaction and EV-mediated interaction with platelets were able to induce CLEC-2-dependent platelet aggregation and finally promote venous thrombosis. However, these are only preliminary data and the exact pro-thrombotic roles of CAFs in HCC are far to be determined, also based on the well-established concept that several factors collaborate in CAT pathogenesis.
CAFs and ECM remodeling
ECM components provide the three-dimensional (3D) scaffold that, beyond the well-established role in liver development, is crucial to direct cellular responses to hepatic damage, especially in the progression to a malignant state[100]. In the healthy liver, ECM is composed of collagens
CAF TARGETING FOR HCC TREATMENT
Based on what was discussed, CAFs may be regarded as putative targets amenable to intervention in solid tumors, including HCC. The main strategies pursued by researchers aimed at CAF manipulation can be ascribed to three fundamental approaches: (A) the depletion of CAFs using surface markers; (B) the inhibition of protumorigenic paracrine signaling pathways induced by CAFs; and (C) targeting of CAF precursors, by inhibiting the activation of PFs and HSCs.
Depletion of CAFs
There are no specific markers uniquely expressed by CAFs in HCC and other solid tumors. For example, αSMA is commonly expressed by multiple cell types of mesenchymal origin, and its targeting could be detrimental to treatment. Currently, the membrane protein most widely used to selectively delete CAFs is FAP-α, which, as mentioned above, is an endopeptidase typically expressed by myofibroblasts at the membrane level in the activated but not in the quiescent state. Currently, this approach, although tested in several cancer types, such as breast and lung cancer, or melanoma, has not been studied for the HCC treatment[117]. In addition to selective targeting of membrane proteins, another line of treatment has been the utilization of BCL2 homology 3 (BH3) mimetics, such as Navitoclax, which induces apoptosis of CAFs but not of non-tumoral myofibroblasts. However, this approach has been shown to be beneficial in mouse models of CCA[91,118], but not of HCC.
Targeting of protumorigenic mediators secreted by CAFs
Another widely used approach of potential interest for the treatment of HCC is based on the use of small molecules able to inhibit the paracrine effects of CAFs on tumor cells (proliferation, invasion, stemness, EMT). To inhibit CAF-induced tumor growth, some efficacy has been shown by T0901317, a liver X receptors (LXR) agonist[119], and by LY2109761, an inhibitor of the TGF-β/mothers against decapentaplegic homolog (SMAD) pathway[56], both halting HCC cell proliferation. Other compounds have instead been shown to be effective by inhibiting motility, such as anti-CCL2, CCL5, and CCL7 antibodies[54] or RvD1[64]. The latter, together with IL-6 neutralizing antibodies, has also shown the ability to hamper stemness in HCC[48].
Inhibition of fibroblast activation
Preventing activation of CAF precursors, i.e., HSCs and PFs, is another strategy that is potentially exploitable. For this purpose, various drugs, even those considered for drug repositioning, have been proposed, such as Metformin[120], Dorsomorphin[121] and Sibrotuzumab[122], and shown promising results in mouse models of HCC, though they are waiting to be evaluated in clinical trials. Finally, good results
CONCLUSIONS AND FUTURE DIRECTIONS
Available evidence indicates that the role of CAFs in HCC has been hitherto less elucidated compared to that reported in CCA, in which the typical desmoplastic reaction has drawn great attention. However, in HCC, CAF effects extend well beyond tumor invasiveness and are likely to play a role in tumor initiation as well. Despite the large body of data regarding the cellular and molecular pathogenesis of HCC, it is rather surprising that there are many important nuances in the multiple functions of CAFs that have yet to be fully understood. The advent of scRNA-seq, with its high-resolution capacity to analyze the molecular signatures of individual cells, has enabled the discrimination of different subtypes of CAFs, revealing their extensive heterogeneity in HCC. A vast range of interactions between CAFs and tumor cells and multiple other cell types and structural components (vessels, ECM) within the TME are becoming increasingly evident, unveiling a multitude of functions, which are frequently pro-tumorigenic but may also be anti-tumorigenic. Among the mechanisms elicited by CAFs to incite HCC progression, PVTT and CAT will deserve consideration by future studies, and possibly provide valuable insights well beyond HCC, given CAT relevance as a leading cause of morbidity and mortality in several types of malignancies. Currently, strategies of intervention aimed at CAFs are still very preliminary but with auspicious potentialities. Starting from observations derived from other cancer types, CAF manipulation might improve the response of current treatment modalities, such as immunotherapy and anti-angiogenesis, which in HCC have been quite disappointing so far compared with results obtained in other malignancies. However, the main limitation of current approaches specifically designed for CAF manipulation is the difficulty in discriminating them from normal fibroblasts. The advent of new and powerful technologies, such as scRNA-seq and artificial intelligence, could be of paramount importance to identify specific markers enabling selective targeting of CAFs, and thus make intervention more effective on pro-tumorigenic activities. Moreover, we believe that dissecting further the variety of phenotypes of CAFs in HCC will provide an asset to devise novel prospects of combination therapies, by adding existing or experimental compounds to immune checkpoint blockade or, eventually, to loco-regional treatments as well to improve their efficacy. In particular, although clinical trials involving the use of immunotherapy or targeted therapy to selectively reduce this cellular component in the TRS are currently not planned neither for HCC nor for other cancer types, preclinical findings support their attractivity as potential therapeutic targets. The ability to manipulate the presence of CAFs and their biological properties would provide a great opportunity to attenuate the aggressive behavior of HCC and, consequently, ameliorate the outcome of these patients.
DECLARATIONS
Authors’ contributions
Conceptualized, drafted, and finalized the manuscript: Cadamuro M, Nuozzi G, Simioni P, Fabris L
Availability of data and materials
Not applicable.
Financial support and sponsorship
PRIN 2022AHM4AA to LF is gratefully acknowledged.
Conflicts of interest
All authors declared that there are no conflicts of interest.
Ethical approval and consent to participate
Not applicable.
Consent for Publication
Not applicable.
Copyright
© The Author(s) 2023.
REFERENCES
1. Llovet JM, Kelley RK, Villanueva A, et al. Hepatocellular carcinoma. Nat Rev Dis Primers 2021;7:6.
2. Myers S, Neyroud-Caspar I, Spahr L, et al. NAFLD and MAFLD as emerging causes of HCC: a populational study. JHEP Rep 2021;3:100231.
3. Vitale A, Svegliati-Baroni G, Ortolani A, et al. Italian Liver Cancer (ITA. LI.CA) group. Epidemiological trends and trajectories of MAFLD-associated hepatocellular carcinoma 2002-2033: the ITA.LI.CA database. Gut 2023;72:141-52.
4. Ping Q, Yan R, Cheng X, et al. Cancer-associated fibroblasts: overview, progress, challenges, and directions. Cancer Gene Ther 2021;28:984-99.
5. Kubo N, Araki K, Kuwano H, Shirabe K. Cancer-associated fibroblasts in hepatocellular carcinoma. World J Gastroenterol 2016;22:6841-50.
6. Baglieri J, Brenner DA, Kisseleva T. The role of fibrosis and liver-associated fibroblasts in the pathogenesis of hepatocellular carcinoma. Int J Mol Sci 2019;20:1723.
7. Wright K, Ly T, Kriet M, Czirok A, Thomas SM. Cancer-associated fibroblasts: master tumor microenvironment modifiers. Cancers 2023;15:1899.
8. Minini M, Fouassier L. Cancer-associated fibroblasts and extracellular matrix: therapeutical strategies for modulating the cholangiocarcinoma microenvironment. Curr Oncol 2023;30:4185-96.
9. Donne R, Lujambio A. The liver cancer immune microenvironment: therapeutic implications for hepatocellular carcinoma. Hepatology 2023;77:1773-96.
10. Feng H, Zhuo Y, Zhang X, et al. Tumor microenvironment in hepatocellular carcinoma: key players for immunotherapy. J Hepatocell Carcinoma 2022;9:1109-25.
11. Liu Y, Xun Z, Ma K, et al. Identification of a tumour immune barrier in the HCC microenvironment that determines the efficacy of immunotherapy. J Hepatol 2023;78:770-82.
12. Tao ZW, Cheng BQ, Zhou T, Gao YJ. Management of hepatocellular carcinoma patients with portal vein tumor thrombosis: a narrative review. Hepatobiliary Pancreat Dis Int 2022;21:134-44.
13. Malehmir M, Pfister D, Gallage S, et al. Platelet GPIbα is a mediator and potential interventional target for NASH and subsequent liver cancer. Nat Med 2019;25:641-55.
14. Zhu AX, Duda DG, Sahani DV, Jain RK. HCC and angiogenesis: possible targets and future directions. Nat Rev Clin Oncol 2011;8:292-301.
15. Llovet JM, Castet F, Heikenwalder M, et al. Immunotherapies for hepatocellular carcinoma. Nat Rev Clin Oncol 2022;19:151-72.
16. Ying F, Chan MSM, Lee TKW. Cancer-associated fibroblasts in hepatocellular carcinoma and cholangiocarcinoma. Cell Mol Gastroenterol Hepatol 2023;15:985-99.
17. Yu L, Shen N, Shi Y, et al. Characterization of cancer-related fibroblasts (CAF) in hepatocellular carcinoma and construction of CAF-based risk signature based on single-cell RNA-seq and bulk RNA-seq data. Front Immunol 2022;13:1009789.
18. Chiavarina B, Ronca R, Otaka Y, et al. Fibroblast-derived prolargin is a tumor suppressor in hepatocellular carcinoma. Oncogene 2022;41:1410-20.
20. Wu F, Yang J, Liu J, et al. Signaling pathways in cancer-associated fibroblasts and targeted therapy for cancer. Signal Transduct Target Ther 2021;6:218.
21. Zhou Y, Ren H, Dai B, et al. Hepatocellular carcinoma-derived exosomal miRNA-21 contributes to tumor progression by converting hepatocyte stellate cells to cancer-associated fibroblasts. J Exp Clin Cancer Res 2018;37:324.
22. Mederacke I, Hsu CC, Troeger JS, et al. Fate tracing reveals hepatic stellate cells as dominant contributors to liver fibrosis independent of its aetiology. Nat Commun 2013;4:2823.
23. Wang SS, Tang XT, Lin M, et al. Perivenous stellate cells are the main source of myofibroblasts and cancer-associated fibroblasts formed after chronic liver injuries. Hepatology 2021;74:1578-94.
24. Filliol A, Saito Y, Nair A, et al. Opposing roles of hepatic stellate cell subpopulations in hepatocarcinogenesis. Nature 2022;610:356-65.
25. Zhang J, Gu C, Song Q, et al. Identifying cancer-associated fibroblasts as emerging targets for hepatocellular carcinoma. Cell Biosci 2020;10:127.
26. Dranoff JA, Wells RG. Portal fibroblasts: underappreciated mediators of biliary fibrosis. Hepatology 2010;51:1438-44.
27. Iwaisako K, Jiang C, Zhang M, et al. Origin of myofibroblasts in the fibrotic liver in mice. Proc Natl Acad Sci U S A 2014;111:E3297-305.
28. Nishio T, Hu R, Koyama Y, et al. Activated hepatic stellate cells and portal fibroblasts contribute to cholestatic liver fibrosis in MDR2 knockout mice. J Hepatol 2019;71:573-85.
29. Yang W, He H, Wang T, et al. Single-cell transcriptomic analysis reveals a hepatic stellate cell-activation roadmap and myofibroblast origin during liver fibrosis in mice. Hepatology 2021;74:2774-90.
30. Quan TE, Bucala R. Culture and analysis of circulating fibrocytes. Methods Mol Med 2007;135:423-34.
31. Kisseleva T, Uchinami H, Feirt N, et al. Bone marrow-derived fibrocytes participate in pathogenesis of liver fibrosis. J Hepatol 2006;45:429-38.
32. Yin Z, Jiang K, Li R, Dong C, Wang L. Multipotent mesenchymal stromal cells play critical roles in hepatocellular carcinoma initiation, progression and therapy. Mol Cancer 2018;17:178.
33. Salah RA, Nasr MA, El-Derby AM, et al. Hepatocellular carcinoma cell line-microenvironment induced cancer-associated phenotype, genotype and functionality in mesenchymal stem cells. Life Sci 2022;288:120168.
34. Zeisberg M, Neilson EG. Biomarkers for epithelial-mesenchymal transitions. J Clin Invest 2009;119:1429-37.
35. Kalluri R, Weinberg RA. The basics of epithelial-mesenchymal transition. J Clin Invest 2009;119:1420-8.
36. Acloque H, Adams MS, Fishwick K, Bronner-Fraser M, Nieto MA. Epithelial-mesenchymal transitions: the importance of changing cell state in development and disease. J Clin Invest 2009;119:1438-49.
37. Strutz F, Zeisberg M, Ziyadeh FN, et al. Role of basic fibroblast growth factor-2 in epithelial-mesenchymal transformation. Kidney Int 2002;61:1714-28.
38. Ye X, Weinberg RA. Epithelial-mesenchymal plasticity: a central regulator of cancer progression. Trends Cell Biol 2015;25:675-86.
39. Zhu G, Xia H, Tang Q, Bi F. An epithelial-mesenchymal transition-related 5-gene signature predicting the prognosis of hepatocellular carcinoma patients. Cancer Cell Int 2021;21:166.
40. Kong W, Mao Z, Han C, et al. A novel epithelial-mesenchymal transition gene signature correlated with prognosis, and immune infiltration in hepatocellular carcinoma. Front Pharmacol 2022;13:863750.
41. Zeisberg M, Yang C, Martino M, et al. Fibroblasts derive from hepatocytes in liver fibrosis via epithelial to mesenchymal transition. J Biol Chem 2007;282:23337-47.
42. Taura K, Miura K, Iwaisako K, et al. Hepatocytes do not undergo epithelial-mesenchymal transition in liver fibrosis in mice. Hepatology 2010;51:1027-36.
43. Chu AS, Diaz R, Hui JJ, et al. Lineage tracing demonstrates no evidence of cholangiocyte epithelial-to-mesenchymal transition in murine models of hepatic fibrosis. Hepatology 2011;53:1685-95.
44. Huang H, Wang Z, Zhang Y, et al. Mesothelial cell-derived antigen-presenting cancer-associated fibroblasts induce expansion of regulatory T cells in pancreatic cancer. Cancer Cell 2022;40:656-73.e7.
45. Elyada E, Bolisetty M, Laise P, et al. Cross-species single-cell analysis of pancreatic ductal adenocarcinoma reveals antigen-presenting cancer-associated fibroblasts. Cancer Discov 2019;9:1102-23.
46. Kerdidani D, Aerakis E, Verrou KM, et al. Lung tumor MHCII immunity depends on in situ antigen presentation by fibroblasts. J Exp Med 2022;219:e20210815.
47. Lau EYT, Lo J, Cheng BYL, et al. Cancer-associated fibroblasts regulate tumor-initiating cell plasticity in hepatocellular carcinoma through C-Met/FRA1/HEY1 signaling. Cell Rep 2016;15:1175-89.
48. Xiong S, Wang R, Chen Q, et al. Cancer-associated fibroblasts promote stem cell-like properties of hepatocellular carcinoma cells through IL-6/STAT3/Notch signaling. Am J Cancer Res 2018;8:302-16.
49. Liu C, Liu L, Chen X, et al. LSD1 stimulates cancer-associated fibroblasts to drive notch3-dependent self-renewal of liver cancer stem-like cells. Cancer Res 2018;78:938-49.
50. Song M, He J, Pan QZ, et al. Cancer-associated fibroblast-mediated cellular crosstalk supports hepatocellular carcinoma progression. Hepatology 2021;73:1717-35.
51. Bai S, Zhao Y, Chen W, et al. The stromal-tumor amplifying STC1-Notch1 feedforward signal promotes the stemness of hepatocellular carcinoma. J Transl Med 2023;21:236.
52. Li Q, Wang C, Wang Y, et al. HSCs-derived COMP drives hepatocellular carcinoma progression by activating MEK/ERK and PI3K/AKT signaling pathways. J Exp Clin Cancer Res 2018;37:231.
53. Xu H, Zhao J, Li J, et al. Cancer associated fibroblast-derived CCL5 promotes hepatocellular carcinoma metastasis through activating HIF1α/ZEB1 axis. Cell Death Dis 2022;13:478.
54. Liu J, Chen S, Wang W, et al. Cancer-associated fibroblasts promote hepatocellular carcinoma metastasis through chemokine-activated hedgehog and TGF-β pathways. Cancer Lett 2016;379:49-59.
55. Liu G, Sun J, Yang ZF, et al. Cancer-associated fibroblast-derived CXCL11 modulates hepatocellular carcinoma cell migration and tumor metastasis through the circUBAP2/miR-4756/IFIT1/3 axis. Cell Death Dis 2021;12:260.
56. Mazzocca A, Fransvea E, Dituri F, Lupo L, Antonaci S, Giannelli G. Down-regulation of connective tissue growth factor by inhibition of transforming growth factor beta blocks the tumor-stroma cross-talk and tumor progression in hepatocellular carcinoma. Hepatology 2010;51:523-34.
57. Kitisin K, Pishvaian MJ, Johnson LB, Mishra L. Liver stem cells and molecular signaling pathways in hepatocellular carcinoma. Gastrointest Cancer Res 2007;1:S13-21.
58. Yoshida GJ. Regulation of heterogeneous cancer-associated fibroblasts: the molecular pathology of activated signaling pathways. J Exp Clin Cancer Res 2020;39:112.
59. Collier JD, Guo K, Gullick WJ, Bassendine MF, Burt AD. Expression of transforming growth factor alpha in human hepatocellular carcinoma. Liver 1993;13:151-5.
60. Capece D, Fischietti M, Verzella D, et al. The inflammatory microenvironment in hepatocellular carcinoma: a pivotal role for tumor-associated macrophages. Biomed Res Int 2013;2013:187204.
61. Baek JY, Morris SM, Campbell J, Fausto N, Yeh MM, Grady WM. TGF-beta inactivation and TGF-alpha overexpression cooperate in an in vivo mouse model to induce hepatocellular carcinoma that recapitulates molecular features of human liver cancer. Int J Cancer 2010;127:1060-71.
62. Thoresen GH, Guren TK, Sandnes D, Peak M, Agius L, Christoffersen T. Response to transforming growth factor α (TGFα) and epidermal growth factor (EGF) in hepatocytes: Lower EGF receptor affinity of TGFα is associated with more sustained activation of p42/p44 mitogen-activated protein kinase and greater efficacy in stimulation of DNA synthesis. J Cell Physiol 1998;175:10-8.
63. Schiffer E, Housset C, Cacheux W, et al. Gefitinib, an EGFR inhibitor, prevents hepatocellular carcinoma development in the rat liver with cirrhosis. Hepatology 2005;41:307-14.
64. Sun L, Wang Y, Wang L, et al. Resolvin D1 prevents epithelial-mesenchymal transition and reduces the stemness features of hepatocellular carcinoma by inhibiting paracrine of cancer-associated fibroblast-derived COMP. J Exp Clin Cancer Res 2019;38:170.
65. Fiaschi T, Marini A, Giannoni E, et al. Reciprocal metabolic reprogramming through lactate shuttle coordinately influences tumor-stroma interplay. Cancer Res 2012;72:5130-40.
66. Martinez-Outschoorn UE, Sotgia F, Lisanti MP. Metabolic asymmetry in cancer: a "balancing act" that promotes tumor growth. Cancer Cell 2014;26:5-7.
67. Martinez-Outschoorn UE, Lisanti MP, Sotgia F. Catabolic cancer-associated fibroblasts transfer energy and biomass to anabolic cancer cells, fueling tumor growth. Semin Cancer Biol 2014;25:47-60.
68. Yuan Q, Zhang J, Liu Y, et al. MyD88 in myofibroblasts regulates aerobic glycolysis-driven hepatocarcinogenesis via ERK-dependent PKM2 nuclear relocalization and activation. J Pathol 2022;256:414-26.
69. De Matteis S, Ragusa A, Marisi G, et al. Aberrant metabolism in hepatocellular carcinoma provides diagnostic and therapeutic opportunities. Oxid Med Cell Longev 2018;2018:7512159.
70. Oura K, Morishita A, Tani J, Masaki T. Tumor immune microenvironment and immunosuppressive therapy in hepatocellular carcinoma: a review. Int J Mol Sci 2021;22:5801.
71. Noy R, Pollard JW. Tumor-associated macrophages: from mechanisms to therapy. Immunity 2014;41:49-61.
72. Arvanitakis K, Koletsa T, Mitroulis I, Germanidis G. Tumor-associated macrophages in hepatocellular carcinoma pathogenesis, prognosis and therapy. Cancers 2022;14:226.
73. Feig C, Jones JO, Kraman M, et al. Targeting CXCL12 from FAP-expressing carcinoma-associated fibroblasts synergizes with anti-PD-L1 immunotherapy in pancreatic cancer. Proc Natl Acad Sci U S A 2013;110:20212-7.
74. Yang F, Wei Y, Han D, et al. Interaction with CD68 and regulation of GAS6 expression by endosialin in fibroblasts drives recruitment and polarization of macrophages in hepatocellular carcinoma. Cancer Res 2020;80:3892-905.
75. Chen S, Morine Y, Tokuda K, et al. Cancer-associated fibroblast-induced M2-polarized macrophages promote hepatocellular carcinoma progression via the plasminogen activator inhibitor-1 pathway. Int J Oncol 2021;59:59.
76. Fabregat I, Caballero-Díaz D. Transforming growth factor-β-induced cell plasticity in liver fibrosis and hepatocarcinogenesis. Front Oncol 2018;8:357.
77. Zulaziz N, Chai SJ, Lim KP. The origins, roles and therapies of cancer associated fibroblast in liver cancer. Front Oncol 2023;13:1151373.
78. Higashino N, Koma YI, Hosono M, et al. Fibroblast activation protein-positive fibroblasts promote tumor progression through secretion of CCL2 and interleukin-6 in esophageal squamous cell carcinoma. Lab Invest 2019;99:777-92.
79. Zheng T, Ma G, Tang M, Li Z, Xu R. IL-8 secreted from M2 macrophages promoted prostate tumorigenesis via STAT3/MALAT1 pathway. Int J Mol Sci 2018;20:98.
80. Hosono M, Koma YI, Takase N, et al. CXCL8 derived from tumor-associated macrophages and esophageal squamous cell carcinomas contributes to tumor progression by promoting migration and invasion of cancer cells. Oncotarget 2017;8:106071-88.
81. Li J, Lau G, Chen L, et al. Interleukin 23 promotes hepatocellular carcinoma metastasis via NF-kappa B induced matrix metalloproteinase 9 expression. PLoS One 2012;7:e46264.
82. Cao L, Fan X, Jing W, et al. Osteopontin promotes a cancer stem cell-like phenotype in hepatocellular carcinoma cells via an integrin-NF-κB-HIF-1α pathway. Oncotarget 2015;6:6627-40.
83. Zhou SL, Zhou ZJ, Hu ZQ, et al. Tumor-associated neutrophils recruit macrophages and t-regulatory cells to promote progression of hepatocellular carcinoma and resistance to sorafenib. Gastroenterology 2016;150:1646-58.e17.
84. Zhou SL, Yin D, Hu ZQ, et al. A positive feedback loop between cancer stem-like cells and tumor-associated neutrophils controls hepatocellular carcinoma progression. Hepatology 2019;70:1214-30.
85. Xu M, Zhao Z, Song J, et al. Interactions between interleukin-6 and myeloid-derived suppressor cells drive the chemoresistant phenotype of hepatocellular cancer. Exp Cell Res 2017;351:142-9.
86. Wang W, Wu J, Ji M, Wu C. Exogenous interleukin-33 promotes hepatocellular carcinoma growth by remodelling the tumour microenvironment. J Transl Med 2020;18:477.
87. Huang B, Lei Z, Zhao J, et al. CCL2/CCR2 pathway mediates recruitment of myeloid suppressor cells to cancers. Cancer Lett 2007;252:86-92.
88. Hoechst B, Voigtlaender T, Ormandy L, et al. Myeloid derived suppressor cells inhibit natural killer cells in patients with hepatocellular carcinoma via the NKp30 receptor. Hepatology 2009;50:799-807.
89. Yang X, Lin Y, Shi Y, et al. FAP Promotes immunosuppression by cancer-associated fibroblasts in the tumor microenvironment via STAT3-CCL2 signaling. Cancer Res 2016;76:4124-35.
90. Cadamuro M, Romanzi A, Guido M, et al. Translational value of tumor-associated lymphangiogenesis in cholangiocarcinoma. J Pers Med 2022;12:1086.
91. Cadamuro M, Brivio S, Mertens J, et al. Platelet-derived growth factor-D enables liver myofibroblasts to promote tumor lymphangiogenesis in cholangiocarcinoma. J Hepatol 2019;70:700-9.
92. Huynh PT, Beswick EJ, Coronado YA, et al. CD90(+) stromal cells are the major source of IL-6, which supports cancer stem-like cells and inflammation in colorectal cancer. Int J Cancer 2016;138:1971-81.
93. Liu Z, Chen M, Zhao R, et al. CAF-induced placental growth factor facilitates neoangiogenesis in hepatocellular carcinoma. Acta Biochim Biophys Sin 2020;52:18-25.
94. Sukowati CHC, Anfuso B, Crocé LS, Tiribelli C. The role of multipotent cancer associated fibroblasts in hepatocarcinogenesis. BMC Cancer 2015;15:188.
95. Lin N, Meng L, Lin J, et al. Activated hepatic stellate cells promote angiogenesis in hepatocellular carcinoma by secreting angiopoietin-1. J Cell Biochem 2020;121:1441-51.
96. Huang B, Huang M, Li Q. Cancer-associated fibroblasts promote angiogenesis of hepatocellular carcinoma by VEGF-mediated EZH2/VASH1 pathway. Technol Cancer Res Treat 2019;18:1533033819879905.
97. Shirai T, Tsukiji N, Sasaki T, et al. Cancer-associated fibroblasts promote venous thrombosis through podoplanin/CLEC-2 interaction in podoplanin-negative lung cancer mouse model. J Thromb Haemost 2023;21:3153-65.
98. Krishnan H, Rayes J, Miyashita T, et al. Podoplanin: an emerging cancer biomarker and therapeutic target. Cancer Sci 2018;109:1292-9.
99. Sasano T, Gonzalez-Delgado R, Muñoz NM, et al. Podoplanin promotes tumor growth, platelet aggregation, and venous thrombosis in murine models of ovarian cancer. J Thromb Haemost 2022;20:104-14.
100. Fabris L, Cadamuro M, Cagnin S, Strazzabosco M, Gores GJ. Liver matrix in benign and malignant biliary tract disease. Semin Liver Dis 2020;40:282-97.
101. Cadamuro M, Stecca T, Brivio S, et al. The deleterious interplay between tumor epithelia and stroma in cholangiocarcinoma. Biochim Biophys Acta Mol Basis Dis 2018;1864:1435-43.
102. Filliol A, Schwabe RF. Contributions of fibroblasts, extracellular matrix, stiffness, and mechanosensing to hepatocarcinogenesis. Semin Liver Dis 2019;39:315-33.
103. Affo S, Yu LX, Schwabe RF. The role of cancer-associated fibroblasts and fibrosis in liver cancer. Annu Rev Pathol 2017;12:153-86.
104. Lai KK, Shang S, Lohia N, et al. Extracellular matrix dynamics in hepatocarcinogenesis: a comparative proteomics study of PDGFC transgenic and Pten null mouse models. PLoS Genet 2011;7:e1002147.
105. Liu XY, Liu RX, Hou F, et al. Fibronectin expression is critical for liver fibrogenesis in vivo and in vitro. Mol Med Rep 2016;14:3669-75.
106. Matsuo M, Sakurai H, Ueno Y, Ohtani O, Saiki I. Activation of MEK/ERK and PI3K/Akt pathways by fibronectin requires integrin alphav-mediated ADAM activity in hepatocellular carcinoma: a novel functional target for gefitinib. Cancer Sci 2006;97:155-62.
107. Fransvea E, Mazzocca A, Antonaci S, Giannelli G. Targeting transforming growth factor (TGF)-betaRI inhibits activation of beta1 integrin and blocks vascular invasion in hepatocellular carcinoma. Hepatology 2009;49:839-50.
108. Zheng X, Wang P, Li L, et al. Cancer-associated fibroblasts promote vascular invasion of hepatocellular carcinoma via downregulating decorin-integrin β1 signaling. Front Cell Dev Biol 2021;9:678670.
109. Giannelli G, Fransvea E, Bergamini C, Marinosci F, Antonaci S. Laminin-5 chains are expressed differentially in metastatic and nonmetastatic hepatocellular carcinoma. Clin Cancer Res 2003;9:3684-91.
110. Bergamini C, Sgarra C, Trerotoli P, et al. Laminin-5 stimulates hepatocellular carcinoma growth through a different function of alpha6beta4 and alpha3beta1 integrins. Hepatology 2007;46:1801-9.
111. Ke AW, Shi GM, Zhou J, et al. CD151 amplifies signaling by integrin α6β1 to PI3K and induces the epithelial-mesenchymal transition in HCC cells. Gastroenterology 2011;140:1629-41.e15.
112. Zhang R, Yao RR, Li JH, et al. Activated hepatic stellate cells secrete periostin to induce stem cell-like phenotype of residual hepatocellular carcinoma cells after heat treatment. Sci Rep 2017;7:2164.
113. Zhao M, Laissue JA, Zimmermann A. Tenascin and type IV collagen expression in liver cell dysplasia and in hepatocellular carcinoma. Histol Histopathol 1996;11:323-33.
114. Nong Y, Wu D, Lin Y, Zhang Y, Bai L, Tang H. Tenascin-C expression is associated with poor prognosis in hepatocellular carcinoma (HCC) patients and the inflammatory cytokine TNF-α-induced TNC expression promotes migration in HCC cells. Am J Cancer Res 2015;5:782-91.
115. Passi M, Zahler S. Mechano-signaling aspects of hepatocellular carcinoma. J Cancer 2021;12:6411-21.
116. Wei J, Yao J, Yang C, et al. Heterogeneous matrix stiffness regulates the cancer stem-like cell phenotype in hepatocellular carcinoma. J Transl Med 2022;20:555.
117. Kaps L, Schuppan D. Targeting cancer associated fibroblasts in liver fibrosis and liver cancer using nanocarriers. Cells 2020;9:2027.
118. Mertens JC, Fingas CD, Christensen JD, et al. Therapeutic effects of deleting cancer-associated fibroblasts in cholangiocarcinoma. Cancer Res 2013;73:897-907.
119. Morén A, Bellomo C, Tsubakihara Y, et al. LXRα limits TGFβ-dependent hepatocellular carcinoma associated fibroblast differentiation. Oncogenesis 2019;8:36.
120. Shankaraiah RC, Callegari E, Guerriero P, et al. Metformin prevents liver tumourigenesis by attenuating fibrosis in a transgenic mouse model of hepatocellular carcinoma. Oncogene 2019;38:7035-45.
121. Mano Y, Yoshio S, Shoji H, et al. Bone morphogenetic protein 4 provides cancer-supportive phenotypes to liver fibroblasts in patients with hepatocellular carcinoma. J Gastroenterol 2019;54:1007-18.
122. Kelly T. Fibroblast activation protein-alpha and dipeptidyl peptidase IV (CD26): cell-surface proteases that activate cell signaling and are potential targets for cancer therapy. Drug Resist Updat 2005;8:51-8.
Cite This Article
How to Cite
Download Citation
Export Citation File:
Type of Import
Tips on Downloading Citation
Citation Manager File Format
Type of Import
Direct Import: When the Direct Import option is selected (the default state), a dialogue box will give you the option to Save or Open the downloaded citation data. Choosing Open will either launch your citation manager or give you a choice of applications with which to use the metadata. The Save option saves the file locally for later use.
Indirect Import: When the Indirect Import option is selected, the metadata is displayed and may be copied and pasted as needed.
About This Article
Copyright
Data & Comments
Data
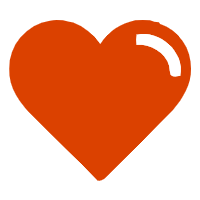
Comments
Comments must be written in English. Spam, offensive content, impersonation, and private information will not be permitted. If any comment is reported and identified as inappropriate content by OAE staff, the comment will be removed without notice. If you have any queries or need any help, please contact us at [email protected].