Autophagy-lysosome pathway as a source of candidate biomarkers for Parkinson’s disease
Abstract
Parkinson’s disease (PD) is a neurodegenerative disorder characterized by progressive motor disturbances and affects more than 1% of the worldwide population. Diagnosis of PD relies on clinical history and physical examination, but misdiagnosis is common in early stages. Despite considerable progress in understanding PD pathophysiology, including genetic and biochemical causes, diagnostic approaches lack accuracy and interventions are restricted to symptomatic treatments. Identification of biomarkers for PD may allow early and more precise diagnosis and monitoring of dopamine replacement strategies and disease-modifying treatments. Increasing evidence suggests that autophagic dysregulation causes the accumulation of abnormal proteins, such as aberrant α-synuclein, a protein critical to PD pathogenesis. Mutations in the GBA gene are a major PD risk factor and β-glucocerebrosidase (GCase) is also emerging as an important molecule in PD pathogenesis. Consequently, proteins involved in the autophagy-lysosome pathway and GCase protein levels and activity are prime targets for the research and development of new PD biomarkers. The studies so far in PD biological material have yielded some consistent results, particularly regarding the levels of Hsc70, a component of the chaperone-mediated autophagy pathway, and the enzymatic activity of GCase in GBA mutation carriers. In the future, larger longitudinal studies, corroborating previous research on possible biomarker candidates, as well as extending the search for possible candidates for other lysosomal components, may yield more definitive results.
Keywords
Introduction
Parkinson’s disease (PD) is the most common neurodegenerative movement disorder affecting approximately 0.5% of the general population, with a prevalence of 3%-4% in people over 80 years old[1,2]. Typical PD symptoms include resting tremor, movement slowness, rigidity, and postural instability (motor symptoms)[3]. In addition, hyposmia, REM sleep behavior disorder, autonomic dysfunction and neuropsychiatric symptoms have more recently been recognized as equally important non-motor features of the disease[4]. The current diagnostic criteria for PD require the presence of parkinsonism, defined as the presence of bradykinesia with either resting tremor or rigidity[5]. There are currently no known disease-modifying treatments, with the main therapeutic target being the management of dopamine deficiency[6].
Unfortunately, the first motor symptoms appear after there is already at least a 40% neuronal loss in the substantia nigra[7], which presents a significant barrier in the discovery and administration of disease-modifying therapies. Therefore, the development of early diagnostic biomarkers able to accurately diagnose the disease years before the manifestation of the first symptoms is imperative. Recently, new criteria for prodromal PD have been proposed to facilitate PD biomarker and progression research to detect the earliest possible stage[8].
To better understand the nature of PD and develop successful biomarkers, and to find effective neuroprotective therapies as well, we need to focus on understanding the pathophysiology of the disease. PD is a neurodegenerative disease characterized by the accumulation of intracellular proteinacious inclusions known as Lewy bodies, a pathological hallmark of both sporadic and familial PD. These inclusions are positive for ubiquitin and α-synuclein (α-syn)[9,10]. α-Syn levels are critical to PD pathogenesis, and the accumulation of aberrant PD-linked forms of the protein may result from dysfunction of protein degradation pathways.
Although the exact function and mechanism of α-syn in degradation are not known, several molecular pathways have been associated with the emergence of Lewy-body pathology, including oxidative stress, mitochondrial function, endoplasmic reticulum stress, the ubiquitin-proteasome system, and the autophagy lysosomal pathway (ALP)[11,12]. ALP is one of the most important pathways in α-syn regulation, since it has been shown that wild-type α-syn is degraded via ALP subpathways in neuronal cells[13-15].
The lysosome is the cellular organelle responsible for the degradation and recycling of organelles and long-lived proteins. It also takes part in the regulation of cellular membrane receptors and molecules[16]. ALP’s main function is to select the cellular components that need to be lysed and lead them to the lysosome for their final degradation[17]. This process can be activated by different cellular stress conditions such as nutrient deprivation[18], or stress derived from accumulated misfolded proteins[19]. This pathway differs from ubiquitin-proteasome system, with the most prominent way being the ability to degrade whole organelles[20].
The importance of lysosome impairment in PD is further supported by emerging evidence of the existence of a link between PD and lysosomal storage disorders. In the last decade, it became apparent that heterozygous mutations in the GBA gene, which encodes β-glucocerebrosidase (GCase), a lysosomal hydrolase, are the most common genetic risk factor for PD[21-24]. Heterozygous carriers of GBA mutations have an increased risk of developing PD, and subjects with GBA mutations who develop Parkinsonism demonstrate on autopsy the typical neuropathological hallmarks of PD[21]. Although GBA is the main focus of contemporary research, there is growing evidence that the genetic burden of gene variants in other lysosomal storage disorder genes is also associated with PD susceptibility[25].
There is a great wealth of information concerning different ALP components in brain cells, especially in the dopaminergic neurons of the substantia nigra, and their possible role in PD pathogenesis. We have chosen to focus only on results concerning either peripheral human tissues, such as blood cells or skin fibroblasts, or readily accessible body fluids, such as plasma, serum or cerebrospinal fluid (CSF). The reasoning behind this was that a biomarker must be obtainable from an easily accessible source with minimally invasive techniques.
Autophagy-lysosome pathway
The main autophagy lysosomal pathways include microautophagy, macroautophagy, and chaperone-mediated autophagy (CMA)[17,26]. Of these, microautophagy is the least understood process[27,28]. In macroautophagy, cup- or rod-shaped double-membrane sequestering structures, called phagophores, are first formed within the cell[17].
Cytosolic constituents such as organelles, proteins and lipids are engulfed by these structures[17,29]. The autophagosome fuses with lysosomes, creating a structure called the autophagolysosome, wherein the vesicular components are eventually degraded[30]. On the other hand, CMA is highly selective, since only cytosolic proteins bearing a KFERQ-like (CMA targeting) motif represent CMA substrates[31,32]. Wild-type α-syn bears such a motif and it has been shown to be degraded by the CMA pathway[14,33,34]. In fact, ALP is considered one of the major routes for the intracellular degradation of α-syn in cells[35,36].
CMA
CMA is a selective mechanism for the degradation of specific cytosolic proteins within lysosomes, and it does not involve vesicle formation. In this process, cytosolic proteins with the particular pentapeptide motif KFERQ or a biochemically related sequence are recognized by a complex of chaperones and cochaperones in the cytosol[31]; the chaperone–cochaperone complex involved in recognition is made up of the 70-kDa heat shock cognate protein (Hsc70), a cytosolic member of the Hsp70 chaperone family[37] and Hsp70 cochaperones (such as Hsp40, Hsp90, Hip, Hop and Bag-1). Following said recognition and binding, substrate proteins are then translocated one by one to the lysosomal membrane, where they bind to the lysosomal transmembrane protein lysosome-associated membrane protein type 2A (Lamp2A) and its surrounding complex, which includes, amongst other components, lysosomal Hsc70; importantly, Lamp2A is considered the rate-limiting step of the pathway, as CMA activity is directly dependent on Lamp2A levels at the lysosomal membrane[38]. Lamp2A is an alternatively spliced form of the LAMP2 gene, and the only one known to participate in CMA[39,40]. Through this binding, which leads to multimerization of Lamp2A and the formation of a cylindrical pore at the lysosomal membrane, substrate proteins are finally threaded into the lysosomes and degraded[32,34].
Consistent results using Western immunoblot analysis show that Hsc70 protein levels are decreased in peripheral blood mononuclear cells (PBMCs) from idiopathic PD (iPD) patients[41,42]. In addition, the same decrease is present in HSC70 mRNA levels in PBMCs from iPD patients. Likewise, PMBCs from genetic PD forms, i.e., PD carriers of the pathogenic A53T SNCA mutation or GBA mutations, also show decreased HSC70 protein levels[42], with no accompanying reduction in the respective mRNA levels. Despite the consistency of the results so far, the value of Hsc70 as a biomarker for PD appears rather low, as the differences between controls and PD are small, albeit highly statistically significant.
Because of its critical function as the rate-limiting step of CMA, Lamp2A represents a commonly used CMA marker. While one study found decreased Lamp2A protein and mRNA levels in the leukocytes of iPD patients compared to controls[43], no difference was evident in PBMCs of such patients in another study[42]. Moreover, there was no difference in Lamp2a protein or mRNA levels in PBMCs from A53T-SNCA or GBA mutation carriers[42].
Macroautophagy
Two important indices of macroautophagic activity levels are p62 (sequestosome-1 or ubiquitin-binding protein p62) and LC-3 (microtubule-associated protein 1A/1B light chain 3, MAP1LC3 or Atg8). LC-3 mediates the further expansion of autophagosomes. This process begins with the establishment of a covalent link between LC-3 and phosphatidylethanolamine. This linkage leads to two changes that are useful as specific indices of macroautophagy: the change of LC3 labeling from a diffuse to a punctate pattern, which can be detected by immunostaining, and a shift of the apparent molecular mass of the protein, which can be detected on Western immunoblots as LC3-II[17]. On the other hand, p62 appears to be a substrate for macroautophagy; hence, its levels increase when macroautophagy is inhibited, and decrease when macroautophagy is induced[17,44].
Results from studies comparing p62 protein levels in PD and healthy controls are conflicting for both idiopathic and genetic PD cases. Most of these studies focused on fibroblasts, with little or no data on p62 levels in PBMCs. In fibroblasts from iPD patients, p62 levels measured by Western immunoblotting showed no differences in one study[45] and were decreased in another[46]. p62 protein levels in fibroblasts derived from parkin (PRKN gene) mutation-carrying PD patients were found to be decreased compared to healthy controls[47], while no differences in p62 levels were found in fibroblasts grown from GBA mutation-carrying PD patients[48]. Finally, a CSF study did not find any difference in p62 levels[49]. It should be noted, however, that changes in p62 can also occur as a result of the Nrf2-Keap1-ARE pathway, associated with oxidative stress[50], and dysregulation of transcription factor EB, a key regulator of autophagic pathways[51]. Both of these pathways have been associated with PD and may contribute to any of the observed differences.
Similarly, LC-3 protein level studies have not produced any conclusive results. One study failed to demonstrate any difference in the ratio of LC-3-I to LC-3-II in PBMCs from iPD patients compared with healthy controls[52], while other studies showed an increase in total LC-3 levels[43,53] in PBMCs. Also, an increase in LC-3 mRNA levels has been found in PBMCs from iPD patients[43]. The same ambiguity exists in results from fibroblasts, where most studies showed no differences[45,54], while a single study found decreased LC-3 levels[46]. Some studies attempted to answer the same question in fibroblasts derived from genetic forms of PD. LC-3 protein levels from PD patients with PRKN mutations were decreased[48], while fibroblasts from PD patients with GBA mutations showed no such difference[47]. Overall, such studies have not shown any major alteration in core maroautophagy read-outs in the context of PD.
Although p62 and LC-3 remain the main targets for macroautophagy-related PD biomarker development in PBMCs, one study measured protein and mRNA levels of different molecules implicated in autophagy regulation and phagophore formation[52]. More specifically, the authors performed a whole-transcriptome assay, which revealed downregulation of mRNAs for 6 core regulators of autophagy [UNC-51-like kinase (ULK) 3, Atg2A, Atg4B, Atg5, Atg16L1, and histone deacetylase 6], while showing upregulated protein levels of ULK1, Beclin1, and autophagy/beclin1 regulator 1[52].
Case of GCase
Homozygous and compound heterozygous GBA mutations cause an inherited autosomal recessive lysosomal storage disorder known as Gaucher disease (GD), characterized by a loss of function of GCase, a lysosomal enzyme involved in sphingolipid metabolism[55], leading to accumulation of its substrates glucosylceramide and glucosylsphingosine in different tissues and organs, such as the liver, blood, spleen, lungs, and nervous system[56,57]. It is estimated that up to 10% of iPD patients (i.e., patients without known family history) are carriers of GBA mutations[58], while asymptomatic carriers have an approximately 5-fold increased risk to develop PD[59,60]. In addition, GBA mutation carriers usually manifest a more severe form of PD with earlier onset, greater cognitive decline and faster progression[61-63].
In general, the results concerning GCase levels in different human samples have been inconclusive. GCase protein levels assessed by Western immunoblotting were decreased in monocytes of iPD patients compared to controls[64] and the same was seen in PBMCs derived from PD patients with the A53T mutation in the SNCA gene and different mutations in the GBA gene, although no differences were found in the case of iPD patients[42]. For the case of GBA-PD patients specifically, both an accompanying increase in GBA mRNA levels[42] and a decrease have been reported[65].
In fibroblasts, most studies have focused on samples acquired from GBA mutation carriers, where both no difference[54] and a decrease[48] in GCase levels were reported. Also, lower GBA mRNA levels were found in patients harboring the L444P GBA mutation[48], while no differences were found in those carrying the N370S or homozygous E326K mutations[48].
Because GCase is an enzyme with known substrates, it is possible to measure directly its enzymatic activity instead of only measuring the respective protein levels, which might give us further insight into the different lysosomal processes implicated in PD pathogenesis and help in the development of clinically useful biomarkers.
Many studies have searched for differences in GCase activity in various different biological samples with conflicting results, mainly regarding GCase activity in PD patients. In CSF, some studies reported decreased enzyme activity[66-68], while other studies refute this finding[69]; similarly with dried blood spots, while one study found a reduction in activity[70], this was not reproduced in another study[71]. Finally, there are reports of decreased GCase activity in serum of iPD patients[66], but no differences seen in lymphocytes[72]. On the other hand, decreased GCase activity is a common finding in dried blood spots of GBA-PD patients, when compared with healthy controls or with PD patients without known mutations[70,71,73]. Surprisingly, increased GCase activity was found in dried blood spots of LRRK2 mutation carriers[70].
In PMBCs, GCase activity was found to be decreased only in GBA mutation carriers compared to healthy controls, with no difference in A53T mutation carriers or iPD patients[42]. Similarly, in another study in peripheral leukocytes, iPD and PRKN mutation carriers showed no differences in GCase activity compared to controls[74]. However, in a study that specifically assessed monocytes, iPD patients showed reduced GCase activity[64]. GCase activity was also lower in GBA-PD patients and in asymptomatic GBA mutation carriers[64].
Additionally, no difference in GCase activity was found in fibroblasts derived from iPD patients and healthy controls[48,54], but decreased activity was found in GBA mutation carriers, including PD patients, asymptomatic carriers and GD patients[48,54].
It therefore appears that a reduction in GCase activity, an assay that is relatively straightforward and easy to perform, in GBA-PD is a consistent finding emerging from a series of studies. Overall, there is a substantial decrease in activity, reaching 50% in some studies. Although values of controls and PD patients on the one hand and GBA-PD patients on the other do overlap to a certain extent, this test with reasonably high sensitivity and specificity may be able to serve as a screening tool for the identification of GBA-PD patients. Specific studies assessing the value of this test as a screening tool need to be conducted.
A word of caution is warranted though; the observed differences in enzyme activities in studies performed by different laboratories could be due to the way enzyme activity is measured. What is observed in vitro may not be entirely reflective of the efficiency of the enzymatic reaction in vivo. This is especially important in PD research where diseased cells with presumably altered lysosomal activity are utilized.
Another, potentially more clinically relevant, use of this test may be the evaluation of enzymatic activity following therapeutic interventions aimed at enhancing GCase activity, e.g., administration of ambroxol. Such interventions are in the process of being evaluated in clinical trials[75,76]. In contrast, more work needs to be done to ascertain whether GCase activity is indeed reduced, perhaps in a cell-specific manner, in peripheral tissues derived from iPD patients.
As mentioned before, mutations in the GBA gene are a major genetic risk factor for developing PD. The actual mechanism through which GBA mutations predispose to PD is unknown, but a growing number of studies indicate that GCase malfunction may lead to α-syn accumulation, whereas at the same time increased/aberrant α-syn may inhibit normal GCase function, generating thus a bidirectional pathogenic loop[77]. As a result, many groups have proposed different oligomeric species of α-syn or lipid substrates of GCase as potential PD biomarkers in PD patients carrying mutations in the GBA gene (GBA-PD).
One study reported elevated α-syn levels in the plasma of GBA-PD patients, with no correlation between lysosomal hydrolases and α-syn levels in dry blood spots[71]. In contrast, α-syn levels in the plasma of healthy GBA carriers did not differ from plasma from non-carriers[78]. Dimer α-syn levels in erythrocyte membranes were higher in GBA-PD individuals compared to healthy controls[79], also seen in iPD patients[79]. Erythrocyte membrane-associated dimeric α-syn was also higher in GD, and correlated with glucosylceramide levels[80,81], suggesting that the conformation of α-syn may be altered in response to the accumulation of GCase substrates and the resultant change in the composition of the erythrocyte membrane. Additionally, a recent study found a decrease in CSF α-syn levels in GBA mutation-carrying patients in comparison with iPD patients and healthy controls[82]. In fact, this decrease was more prominent in GBA mutations associated with more severe forms of PD[82], which may also present in GBA-associated Lewy-body dementia[83].
As mentioned earlier GCase activity levels were reported to be decreased in monocytes of iPD patients. In the same study, a similar decrease in the levels of 36 out of 59 ceramide species in plasma was reported, with a positive correlation between GCase activity and ceramide species levels[64].
Other lysosomal components
Although an important link exists between GCase and the development of PD, GCase is not the only lysosomal enzyme studied in connection with PD. Other such proteins are in the families of galactosidases and cathepsins and the enzyme β-hexosaminidase. In addition, genetic loci implicated in other lysosomal storage disorders have been associated with increased PD risk, reinforcing the importance of lysosomal mechanisms in PD pathogenesis[25].
Mutations in the gene encoding α-galactosidase (GLA), a lysosomal glycoside hydrolase, result in the rare lysosomal storage disorder Fabry disease, with an X-linked inheritance pattern[84]. Although no indications have been uncovered for a direct link between mutations in the GLA gene and PD risk, α-galactosidase (α-gal) is an interesting candidate PD biomarker. Both α-gal protein levels and enzymatic activity were found to be decreased in leukocytes from iPD patients compared to healthy controls[43,85]. At the same time, the enzymatic activity of α-gal in dried blood spots was found to be either reduced[86] or not different[71,87] in iPD patients, and decreased in GBA mutation carriers[71]. In the same vein with GCase, increased activity was found in LRRK2 mutation carriers[86], suggesting the possibility that in these cases there is a general compensatory upregulation of lysosomal activity.
Another member of the same family is β-galactosidase (β-gal). As with α-gal, the results of its enzymatic activity levels in iPD patients are mixed. There are reports of an increase in serum[87] and CSF[69], and of no difference again in serum[66] and CSF[66,67]. When compared with α-gal activity, β-gal activity was found to be elevated in serum samples from iPD patients[87].
In the cases of β-hexosaminidase and cathepsins D and E, the results are not very clear. There are conflicting accounts for the activity of cathepsins D and E in the CSF, with either no differences[69] or a decrease in cathepsin D[68] and an increase in cathepsin E[69]. Regarding β-hexosaminidase, only one study reported an elevation in activity in the CSF of iPD patients[67], while most other studies uncovered no such difference in CSF[66,68,69], serum[87], or leukocytes[74,85].
Total lysosomal function
Although disturbances in the ALP subcomponents is the main focus of most PD biomarker studies, a number of interesting results have recently emerged measuring lysosomal activity as a whole. Such studies consider the properties of lysosomes as organelles and their ability to perform their main function of degrading and recycling cellular proteins.
A recent study from our laboratory showed a reduction in total lysosomal activity in PBMCs derived from iPD patients using 3H-leucine pulse-chase experiments, measuring the rate of long-lived intracellular proteins[88]. The same reduction was also apparent in the macroautophagy and CMA pathways[88]. Similar results were obtained with fibroblasts from PRKN mutation carriers, where compared to healthy controls, decreased proteolytic activity and decreased levels of lysosomal function markers such as RAB7A and ATP6V1G1 were found in PD patients[89]. In the same study, a possibly compensatory increase in lysosomal compartments (lysosome number and size), stained with lysosomal fluorescent markers, was reported[89].
Conclusion
Although ALP is firmly established as one of the main cellular mechanisms implicated in the pathogenesis of PD, the use of its component proteins as PD biomarkers cannot be clearly established with the present amount of information. The decrease in the essential CMA component Hsc70 in PBMCs of PD patients appears to be a robust finding, but it is not suitable as a biomarker, since differences between PD patients and controls, although significant, are relatively small. GCase activity, and possibly other biochemical indices resulting from GCase dysfunction, may prove useful in screening or following progression and response in disease-modifying treatments in GBA-PD patients, but this will require further study. Lysosomal proteolytic activity deserves further scrutiny as a potential biomarker that may be able to segregate different PD subtypes. Setting aside their usefulness as biomarkers, indices of ALP function in PD biological material deserve to be examined further, as they provide insights into disease pathogenesis and the development of novel disease-modifying treatments.
Declarations
Authors’ contributions
Performed a literature search on the topic, wrote the first draft of the paper, as well as its revised version following the reviews: Papagiannakis N
Conceived the study, and edited the first and final drafts of the paper: Stefanis L
Availability of data and materials
Not applicable.
Financial support and sponsorship
None.
Conflicts of interest
All authors declared that there are no conflicts of interest.
Ethical approval and consent to participate
Not applicable.
Consent for publication
Not applicable.
Copyright
© The Author(s) 2021.
REFERENCES
2. Hirsch L, Jette N, Frolkis A, Steeves T, Pringsheim T. The incidence of Parkinson’s disease: a systematic review and meta-analysis. Neuroepidemiology 2016;46:292-300.
3. Jankovic J. Parkinson’s disease: clinical features and diagnosis,. J Neurol Neurosurg Psychiatry 2008;79:368-76.
4. Todorova A, Jenner P, Ray Chaudhuri K. Non-motor Parkinson’s: integral to motor Parkinson’s, yet often neglected. Pract Neurol 2014;14:310-22.
5. Postuma RB, Berg D, Stern M, Poewe W, Olanow CW, et al. MDS clinical diagnostic criteria for Parkinson’s disease. Mov Disord 2015;30:1591-601.
6. Connolly BS, Lang AE. Pharmacological treatment of Parkinson disease: a review. JAMA 2014;311:1670-83.
7. Cheng HC, Ulane CM, Burke RE. Clinical progression in Parkinson disease and the neurobiology of axons. Ann Neurol 2010;67:715-25.
8. Berg D, Postuma RB, Adler CH, Bloem BR, Chan P, et al. MDS research criteria for prodromal Parkinson’s disease. Mov Disord 2015;30:1600-11.
9. Spillantini MG, Schmidt ML, Lee VM, Trojanowski JQ, Jakes R, et al. Alpha-synuclein in Lewy bodies. Nature 1997;388:839-40.
10. Spillantini MG, Crowther RA, Jakes R, Hasegawa M, Goedert M. alpha-Synuclein in filamentous inclusions of Lewy bodies from Parkinson’s disease and dementia with lewy bodies. Proc Natl Acad Sci U S A 1998;95:6469-73.
11. Klemann CJHM, Martens GJM, Sharma M, Martens MB, Isacson O, et al. Integrated molecular landscape of Parkinson’s disease. NPJ Parkinsons Dis 2017;3:14.
12. Xicoy H, Peñuelas N, Vila M, Laguna A. Autophagic- and Lysosomal-Related Biomarkers for Parkinson’s Disease: Lights and Shadows. Cells 2019;8:1317.
13. Cuervo AM, Stefanis L, Fredenburg R, Lansbury PT, Sulzer D. Impaired degradation of mutant alpha-synuclein by chaperone-mediated autophagy. Science 2004;305:1292-5.
14. Vogiatzi T, Xilouri M, Vekrellis K, Stefanis L. Wild type alpha-synuclein is degraded by chaperone-mediated autophagy and macroautophagy in neuronal cells. J Biol Chem 2008;283:23542-56.
15. Mak SK, McCormack AL, Manning-Bog AB, Cuervo AM, Di Monte DA. Lysosomal degradation of alpha-synuclein in vivo. J Biol Chem 2010;285:13621-9.
17. Xilouri M, Stefanis L. Autophagic pathways in Parkinson disease and related disorders. Expert Rev Mol Med 2011;13:e8.
18. Menzies FM, Fleming A, Caricasole A, Bento CF, Andrews SP, et al. Autophagy and Neurodegeneration: Pathogenic Mechanisms and Therapeutic Opportunities. Neuron 2017;93:1015-34.
19. Rashid HO, Yadav RK, Kim HR, Chae HJ. ER stress: Autophagy induction, inhibition and selection. Autophagy 2015;11:1956-77.
20. Müller O, Sattler T, Flötenmeyer M, Schwarz H, Plattner H, et al. Autophagic tubes: vacuolar invaginations involved in lateral membrane sorting and inverse vesicle budding. J Cell Biol 2000;151:519-28.
21. Neumann J, Bras J, Deas E, O’Sullivan SS, Parkkinen L, et al. Glucocerebrosidase mutations in clinical and pathologically proven Parkinson’s disease. Brain 2009;132:1783-94.
22. Sidransky E, Nalls MA, Aasly JO, Aharon-Peretz J, Annesi G, et al. Multicenter analysis of glucocerebrosidase mutations in Parkinson’s disease. N Engl J Med 2009;361:1651-61.
23. Nalls MA, Duran R, Lopez G, Kurzawa-Akanbi M, McKeith IG, et al. A multicenter study of glucocerebrosidase mutations in dementia with Lewy bodies. JAMA Neurol 2013;70:727-35.
25. Robak LA, Jansen IE, van Rooij J, Uitterlinden AG, Kraaij R, et al; International Parkinson’s Disease Genomics Consortium (IPDGC). Excessive burden of lysosomal storage disorder gene variants in Parkinson’s disease. Brain 2017;140:3191-203.
27. Martinez-vicente M, Cuervo AM. Autophagy and neurodegeneration: when the cleaning crew goes on strike. Lancet Neurol 2007;6:352-61.
28. Li WW, Li J, Bao JK. Microautophagy: lesser-known self-eating. Cell Mol Life Sci 2012;69:1125-36.
29. Yang YP, Liang ZQ, Gu ZL, Qin ZH. Molecular mechanism and regulation of autophagy. Acta Pharmacol Sin 2005;26:1421-34.
30. Köchl R, Hu XW, Chan EY, Tooze SA. Microtubules facilitate autophagosome formation and fusion of autophagosomes with endosomes. Traffic 2006;7:129-45.
31. Dice JF. Peptide sequences that target cytosolic proteins for lysosomal proteolysis. Trends Biochem Sci 1990;15:305-9.
32. Dice JF, Terlecky SR, Chiang HL, Olson TS, Isenman LD, et al. A selective pathway for degradation of cytosolic proteins by lysosomes. Semin Cell Biol 1990;1:449-55.
33. Xilouri M, Brekk OR, Landeck N, Pitychoutis PM, Papasilekas T, et al. Boosting chaperone-mediated autophagy in vivo mitigates α-synuclein-induced neurodegeneration. Brain 2013;136:2130-46.
34. Cuervo AM, Wong E. Chaperone-mediated autophagy: roles in disease and aging. Cell Res 2014;24:92-104.
35. Xilouri M, Brekk OR, Stefanis L. Autophagy and alpha-synuclein: relevance to Parkinson’s disease and related synucleopathies. Mov Disord 2016;31:178-92.
36. Moors T, Paciotti S, Chiasserini D, Calabresi P, Parnetti L, et al. Lysosomal dysfunction and α-synuclein aggregation in Parkinson’s disease: diagnostic links. Mov Disord 2016;31:791-801.
37. Chiang HL, Terlecky SR, Plant CP, Dice JF. A role for a 70-kilodalton heat shock protein in lysosomal degradation of intracellular proteins. Science 1989;246:382-5.
38. Bandyopadhyay U, Cuervo AM. Entering the lysosome through a transient gate by chaperone-mediated autophagy. Autophagy 2008;4:1101-3.
39. Hatem CL, Gough NR, Fambrough DM. Multiple mRNAs encode the avian lysosomal membrane protein LAMP-2, resulting in alternative transmembrane and cytoplasmic domains. J Cell Sci 1995;108:2093-100.
40. Cuervo AM, Dice JF. Unique properties of lamp2a compared to other lamp2 isoforms. J Cell Sci 2000;113:4441-50.
41. Sala G, Stefanoni G, Arosio A, Riva C, Melchionda L, et al. Reduced expression of the chaperone-mediated autophagy carrier hsc70 protein in lymphomonocytes of patients with Parkinson’s disease. Brain Res 2014;1546:46-52.
42. Papagiannakis N, Xilouri M, Koros C, Stamelou M, Antonelou R, et al. Lysosomal alterations in peripheral blood mononuclear cells of Parkinson’s disease patients. Mov Disord 2015;30:1830-4.
43. Wu G, Wang X, Feng X, Zhang A, Li J, et al. Altered expression of autophagic genes in the peripheral leukocytes of patients with sporadic Parkinson’s disease. Brain Res 2011;1394:105-11.
44. Bjørkøy G, Lamark T, Pankiv S, Øvervatn A, Brech A, et al. Monitoring autophagic degradation of p62/SQSTM1. Methods Enzymol 2009;452:181-97.
45. Ambrosi G, Ghezzi C, Sepe S, Milanese C, Payan-Gomez C, et al. Bioenergetic and proteolytic defects in fibroblasts from patients with sporadic Parkinson’s disease. Biochim Biophys Acta 2014;1842:1385-94.
46. Teves JMY, Bhargava V, Kirwan KR, Corenblum MJ, Justiniano R, et al. Parkinson’s disease skin fibroblasts display signature alterations in growth, redox homeostasis, mitochondrial function, and autophagy. Front Neurosci 2017;11:737.
47. González-Casacuberta I, Juárez-Flores DL, Ezquerra M, Fucho R, Catalán-García M, et al. Mitochondrial and autophagic alterations in skin fibroblasts from Parkinson disease patients with Parkin mutations. Aging (Albany NY) 2019;11:3750-67.
48. McNeill A, Magalhaes J, Shen C, Chau KY, Hughes D, et al. Ambroxol improves lysosomal biochemistry in glucocerebrosidase mutation-linked Parkinson disease cells. Brain 2014;137:1481-95.
49. Boman A, Svensson S, Boxer A, Rojas JC, Seeley WW, et al. Distinct lysosomal network protein profiles in parkinsonian syndrome cerebrospinal fluid. J Parkinsons Dis 2016;6:307-15.
50. Jiang T, Harder B, Rojo de la Vega M, Wong PK, Chapman E, et al. p62 links autophagy and Nrf2 signaling. Free Radic Biol Med 2015;88:199-204.
51. Cortes CJ, La Spada AR. TFEB dysregulation as a driver of autophagy dysfunction in neurodegenerative disease: molecular mechanisms, cellular processes, and emerging therapeutic opportunities. Neurobiol Dis 2019;122:83-93.
52. Miki Y, Shimoyama S, Kon T, Ueno T, Hayakari R, et al. Alteration of autophagy-related proteins in peripheral blood mononuclear cells of patients with Parkinson’s disease. Neurobiol Aging 2018;63:33-43.
53. Prigione A, Piazza F, Brighina L, Begni B, Galbussera A, et al. Alpha-synuclein nitration and autophagy response are induced in peripheral blood cells from patients with Parkinson disease. Neurosci Lett 2010;477:6-10.
54. Collins LM, Drouin-Ouellet J, Kuan WL, Cox T, Barker RA. Dermal fibroblasts from patients with Parkinson’s disease have normal GCase activity and autophagy compared to patients with PD and GBA mutations. Version 2. F1000Res 2017;6:1751.
56. Lieberman RL. A Guided Tour of the Structural Biology of Gaucher Disease: Acid-β-Glucosidase and Saposin C. Enzyme Res 2011;2011:973231.
57. Stirnemann J, Belmatoug N, Camou F, Serratrice C, Froissart R, et al. A Review of Gaucher Disease Pathophysiology, Clinical Presentation and Treatments. Int J Mol Sci 2017;18:441.
58. Li Y, Sekine T, Funayama M, Li L, Yoshino H, et al. Clinicogenetic study of GBA mutations in patients with familial Parkinson’s disease. Neurobiol Aging 2014;35:935.e3-8.
59. Anheim M, Elbaz A, Lesage S, Durr A, Condroyer C, et al; French Parkinson Disease Genetic Group. Penetrance of Parkinson disease in glucocerebrosidase gene mutation carriers. Neurology 2012;78:417-20.
60. Rana HQ, Balwani M, Bier L, Alcalay RN. Age-specific Parkinson disease risk in GBA mutation carriers: information for genetic counseling. Genet Med 2013;15:146-9.
61. Winder-Rhodes SE, Evans JR, Ban M, Mason SL, Williams-Gray CH, et al. Glucocerebrosidase mutations influence the natural history of Parkinson’s disease in a community-based incident cohort. Brain 2013;136:392-9.
62. Liu G, Boot B, Locascio JJ, Jansen IE, Winder-Rhodes S, et al; International Genetics of Parkinson Disease Progression (IGPP) Consortium. Specifically neuropathic Gaucher’s mutations accelerate cognitive decline in Parkinson’s. Ann Neurol 2016;80:674-85.
63. Simitsi A, Koros C, Moraitou M, Papagiannakis N, Antonellou R, et al. Phenotypic characteristics in GBA-associated Parkinson’s disease: a study in a greek population. J Parkinsons Dis 2018;8:101-5.
64. Atashrazm F, Hammond D, Perera G, Dobson-Stone C, Mueller N, et al. Reduced glucocerebrosidase activity in monocytes from patients with Parkinson’s disease. Sci Rep 2018;8:15446.
65. Ichinose Y, Ishiura H, Tanaka M, Yoshimura J, Doi K, et al. Neuroimaging, genetic, and enzymatic study in a Japanese family with a GBA gross deletion. Parkinsonism Relat Disord 2019;61:57-63.
66. Balducci C, Pierguidi L, Persichetti E, Parnetti L, Sbaragli M, et al. Lysosomal hydrolases in cerebrospinal fluid from subjects with Parkinson’s disease. Mov Disord 2007;22:1481-4.
67. Parnetti L, Chiasserini D, Persichetti E, Eusebi P, Varghese S, et al. Cerebrospinal fluid lysosomal enzymes and alpha-synuclein in Parkinson’s disease. Mov Disord 2014;29:1019-27.
68. Parnetti L, Paciotti S, Eusebi P, Dardis A, Zampieri S, et al. Cerebrospinal fluid β-glucocerebrosidase activity is reduced in parkinson’s disease patients. Mov Disord 2017;32:1423-31.
69. van Dijk KD, Persichetti E, Chiasserini D, Eusebi P, Beccari T, et al. Changes in endolysosomal enzyme activities in cerebrospinal fluid of patients with Parkinson’s disease. Mov Disord 2013;28:747-54.
70. Alcalay RN, Levy OA, Waters CC, Fahn S, Ford B, et al. Glucocerebrosidase activity in Parkinson’s disease with and without GBA mutations. Brain 2015;138:2648-58.
71. Pchelina S, Emelyanov A, Baydakova G, Andoskin P, Senkevich K, et al. Oligomeric α-synuclein and glucocerebrosidase activity levels in GBA-associated Parkinson’s disease. Neurosci Lett 2017;636:70-6.
72. Cerri S, Ghezzi C, Sampieri M, Siani F, Avenali M, et al. The exosomal/total α-synuclein ratio in plasma is associated with glucocerebrosidase activity and correlates with measures of disease severity in PD patients. Front Cell Neurosci 2018;12:125.
73. Ortega RA, Torres PA, Swan M, Nichols W, Boschung S, et al. Glucocerebrosidase enzyme activity in GBA mutation Parkinson’s disease. J Clin Neurosci 2016;28:185-6.
74. Kim HJ, Jeon B, Song J, Lee WW, Park H, et al. Leukocyte glucocerebrosidase and β-hexosaminidase activity in sporadic and genetic Parkinson disease. Parkinsonism Relat Disord 2016;23:99-101.
75. Silveira CRA, MacKinley J, Coleman K, Li Z, Finger E, et al. Ambroxol as a novel disease-modifying treatment for Parkinson’s disease dementia: protocol for a single-centre, randomized, double-blind, placebo-controlled trial. BMC Neurol 2019;19:20.
76. Mullin S, Smith L, Lee K, D’Souza G, Woodgate P, et al. Ambroxol for the treatment of patients with Parkinson disease with and without glucocerebrosidase gene mutations: a nonrandomized, noncontrolled trial. JAMA Neurol 2020:427.
77. Mazzulli JR, Xu YH, Sun Y, Knight AL, McLean PJ, et al. Gaucher disease glucocerebrosidase and α-synuclein form a bidirectional pathogenic loop in synucleinopathies. Cell 2011;146:37-52.
78. Pchelina SN, Nuzhnyi EP, Emelyanov AK, Boukina TM, Usenko TS, et al. Increased plasma oligomeric alpha-synuclein in patients with lysosomal storage diseases. Neurosci Lett 2014;583:188-93.
79. Papagiannakis N, Koros C, Stamelou M, Simitsi AM, Maniati M, et al. Alpha-synuclein dimerization in erythrocytes of patients with genetic and non-genetic forms of Parkinson’s Disease. Neurosci Lett 2018;672:145-9.
80. Argyriou A, Dermentzaki G, Papasilekas T, Moraitou M, Stamboulis E, et al. Increased dimerization of alpha-synuclein in erythrocytes in Gaucher disease and aging. Neurosci Lett 2012;528:205-9.
81. Moraitou M, Dermentzaki G, Dimitriou E, Monopolis I, Dekker N, et al. α-Synuclein dimerization in erythrocytes of Gaucher disease patients: correlation with lipid abnormalities and oxidative stress. Neurosci Lett 2016;613:1-5.
82. Lerche S, Wurster I, Roeben B, Zimmermann M, Riebenbauer B, et al. Parkinson’s disease: glucocerebrosidase 1 mutation severity is associated with CSF alpha-synuclein profiles. Mov Disord 2020;35:495-9.
83. Lerche S, Machetanz G, Wurster I, Roeben B, Zimmermann M, et al. Dementia with lewy bodies: GBA1 mutations are associated with cerebrospinal fluid alpha-synuclein profile. Mov Disord 2019;34:1069-73.
85. Wu G, Yan B, Wang X, Feng X, Zhang A, et al. Decreased activities of lysosomal acid alpha-D-galactosidase A in the leukocytes of sporadic Parkinson’s disease. J Neurol Sci 2008;271:168-73.
86. Alcalay RN, Wolf P, Levy OA, Kang UJ, Waters C, et al. Alpha galactosidase A activity in Parkinson’s disease. Neurobiol Dis 2018;112:85-90.
87. Niimi Y, Ito S, Mizutani Y, Murate K, Shima S, et al. Altered regulation of serum lysosomal acid hydrolase activities in Parkinson’s disease: A potential peripheral biomarker? Parkinsonism Relat Disord 2019;61:132-7.
88. Papagiannakis N, Xilouri M, Koros C, Simitsi AM, Stamelou M, et al. Autophagy dysfunction in peripheral blood mononuclear cells of Parkinson’s disease patients. Neurosci Lett 2019;704:112-5.
Cite This Article

How to Cite
Download Citation
Export Citation File:
Type of Import
Tips on Downloading Citation
Citation Manager File Format
Type of Import
Direct Import: When the Direct Import option is selected (the default state), a dialogue box will give you the option to Save or Open the downloaded citation data. Choosing Open will either launch your citation manager or give you a choice of applications with which to use the metadata. The Save option saves the file locally for later use.
Indirect Import: When the Indirect Import option is selected, the metadata is displayed and may be copied and pasted as needed.
About This Article
Copyright
Data & Comments
Data
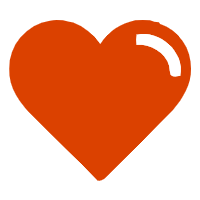
Comments
Comments must be written in English. Spam, offensive content, impersonation, and private information will not be permitted. If any comment is reported and identified as inappropriate content by OAE staff, the comment will be removed without notice. If you have any queries or need any help, please contact us at [email protected].