A baseline for microplastic occurrence in three New England estuaries
Abstract
Although microplastics (MP) have been documented in estuarine habitats, limited published data exist for New Hampshire and northern Massachusetts hampering meaningful, regional comparison with other geographies. Here we synthesize previously unpublished data from several independent baseline studies spanning three estuarine systems including Great Bay Estuary (GBE), Hampton-Seabrook Estuary (HSE), and Great Marsh Estuary (GME) to compare geographic data for MP to other published regional studies. Data include water column in GBE (n = 179 from 7 sites), surface waters and salt marsh sediment cores from HSE (n = 72 water samples from 12 sites and
Keywords
INTRODUCTION
As early as the 1970s, marine scientists noted the occurrence of microplastic particles (MP, particles < 5mm) in nearly every aquatic system studied[1-7] and because of their persistence, MP is an emerging water pollutant of concern[8]. Recent studies have confirmed the roles that coastal systems play in attenuating microplastics[9], including deposition in marine sediments[10] and salt marsh peat[11-13]. Subsequently plastics have been found to serve as the substrate for the growth of microbes, both prokaryote and eukaryote[6,14-17], and as such, scientists have posited that MP are responsible for movement and redistribution of organisms both benign and harmful, within and across ecosystems[15,16,18-23]. Having codified that this new “Plastisphere”[17] realm constitutes a new and diverse microbial niche with biogeochemical consequences[21,24-26], there now exists a series of questions regarding the environmental fate and consequences of plastics and their epiplastic communities[27]. Numerous studies show that zooplankton, mollusks, fishes, crustaceans, birds, and cetaceans consume MP, and those particles are found in soft tissues, muscle, liver, and gut (10-1,000 s of particles per individual)[8,28-37]. Recent reports quantify actual levels of MP in seafood[38-42] with specific reference to MP location (gut, gills, tissues) within organisms. Numerous studies indicate that MP may damage aquatic organisms (including higher trophic levels) by blocking digestive tracts, and by altering feeding and reproductive patterns. To understand how MP are acquired and concentrated in living resources, data are needed for the ambient occurrence of MP in aquatic systems. A recent study of MP in Great Bay Estuary sediments[10] found levels as high as 100 ± 50 MP·g-1. Results of inputting MP sediment data into a hydrodynamic and particle transport model suggested the highest MP deposition occurs in regions with weaker hydrodynamic flows and lower bed shear stress such as eelgrass meadows and along the fringes of the Bay. Other models of MP settlement in estuaries recently have been published[43], and together such tools will enable prediction of the tributary sources, movements, and fate of MP within these aquatic habitats. To better inform such hydrodynamic models, data are needed for the ambient quantities, types, densities, sizes, etc. of MP currently present within these estuaries. Because MP have not been well studied in estuarine habitats of New England, additional studies were performed in three estuarine systems in this geography, including Great Bay Estuary (GBE) in New Hampshire, Hampton-Seabrook Estuary (HSE) in New Hampshire and Great Marsh Estuary (GME) in Massachusetts to gather baseline knowledge of ambient levels of MP in the water column and intertidal sediments. Provided here is a summary of MP data from samples derived from contemporaneous independent studies conducted by our group. While it is important to note there are differences in approach, analysis, and study goals reported herein, the data constitute an important regional summary and a baseline in support of future work in this region.
METHODS
Sampled regions
The coastal GBE is surrounded by rural, agricultural, and forested areas and is fed by 7 tributaries that are typically low-discharge systems, providing limited freshwater[44]. In contrast, HSE and GME are barrier marsh systems that were formed behind the multi-state barrier beach system, serving as the primary coastal landform that protects homes, businesses, infrastructure, and estuarine resources of the marsh complex. Although these two systems each have their individual tidesheds, both ecologically and hydrologically, HSE is a component of GME, collectively spanning 10 municipalities from Cape Ann, Massachusetts to Hampton, New Hampshire. These three estuarine systems [Figure 1] contain some of the most outstanding ecological and economic resources on the Eastern Seaboard thanks to unparalleled conservation actions and coordination of multiple regional organizations and agencies.
Figure 1. Map of New England (top left) and individual maps of estuarine sites where samples were analyzed for microplastics between 2018-2023. (A) GBE, New Hampshire; (B) HSE, New Hampshire; (C) GME, Massachusetts. GBE: Great Bay Estuary; GME: Great Marsh Estuary; HSE: Hampton-Seabrook Estuary.
Multiple sites and sampling approaches within three New England regions were accessed to assemble a regional summary of MP in the geography provided herein [Table 1]. Although each of these studies is reported here, it should be noted that these efforts were not necessarily coordinated efforts; thus there are differences in methodology and approach. Nevertheless, all resulting data were analyzed using a uniform approach to create this summary regional report. In GBE and HSE, both in New Hampshire, water samples of different types (bulk and manta trawl) were collected. The GBE water column samples had been collected previously (2018-2022) for a multiyear survey of oyster larva abundance. Analysis of archived samples from a previous study of sediment size characteristics provided data for MP in GBE sediments[10]. Thus, sediment cores were collected only in HSE (at high and low marsh areas in 2021). There were no prior samples from GME, which is situated on the north shore of Massachusetts. At that site, only water samples were collected between 2021-2023 [Figure 1].
Details of samples from three New England estuaries that were investigated for microplastic content
Sampling location | Sampling sites | Number of samples | Sampling period | Collection method | Flow rate (m·s-1) | Filter size cutoff |
GBE water (Column) | 7 | 179 | Feb-Nov 2018-2022 | Subsurface trawl | 0.5-1.0 | 5 μm - 5 mm |
HSE water (Surface) | 12 | 72 | Jul-Sep 2021 | Bulk | N/A | 5 μm - 5 mm |
HSE water (Surface) | 12 | 72 | Jul-Sep 2021 | Manta trawl | 0.2 ± 0.01 (0.003-0.6) | 5 μm - 5 mm |
HSE intertidal sediment | 9 | 18 | Jul 2021 | 4 cm × 10 cm cores | N/A | 1 μm - 5 mm |
GME water (Surface) | 17 | 42 | May-Nov 2021-2023 | Manta trawl | 0.5 ± -0.04 (0.2-1.3) | 5 μm - 5 mm |
Field collections
Water sampling (GBE, HSE, and GME)
Water column samples from GBE were derived from samples archived from a prior study designed to document presence of oyster larvae. Previously preserved samples were derived from replicate horizontal tows using a 64 μm mesh net held at ~0.3 m below the surface, resulting in an average of 38 m3 of water sampled per tow[45]. In a separate study area, surface water in HSE was sampled using both manta trawl with 330 μm mesh[46,47] and bulk sampling[47] whereas surface water sampling in GME employed only the 330 μm mesh manta trawl. At both HSE and GME, horizontal surface-water tows were performed resulting in an average of 166 m3 of water sampled per tow. Tows in GBE were made at slack tide and sample collections were timed to be as unaffected as possible by rainfall. Tows in HSE and GME were collected on an outgoing tide and rainfall within 48 hours was noted. The collected material was preserved and stored in the dark until processed for MP. Field blanks were integrated with sampling; one blank was collected for every six environmental samples[47-49]. Manta trawl blanks were taken by rinsing the outside of the clean net with
Bulk water sampling (HSE)
Bulk samples were collected in tandem with all manta trawl samples at HSE. At each site, 1 L glass jars triple-rinsed with filtered H2O were used to collect water samples by submerging the jar just below the surface, allowing it to fill, and capping it underwater. Field blanks were integrated into the sampling plan, with one blank taken for every six samples collected by pouring ~1 L of DI water into a glass jar in the field[47]. All blanks were taken at established sampling locations to best replicate actual sampling conditions[47-49]. To assess variability among samples, replicates also were collected in the field[50,51] immediately following the initial sample collection.
Sediment core sampling
A prior published study[10] provides MP data for GBE. To investigate the potential for differential deposition by habitat location, HSE sediments were sampled in both high and low marsh habitats. A piston coring device (4 cm diameter and 10 cm long) was used to collect sediments proximal to surface water sampling sites in HSE [Figure 1]. The top 10 cm of the core was used for MP determinations, representing approximately 35-40 years of sediment accretion based on regional accretion rate data[52]. Cores were held on ice for transportation to the laboratory[46] and stored at 40 oF until processed.
Laboratory analyses
Efforts completed across the three estuaries were disparate, and various approaches were used to minimize cross contamination in the field and lab. All studies included filtering all reagents (0.2 μm), triple-rinsing glassware with filtered diH2O, performing manipulations under a fume hood, wearing cotton garb, and covering all containers with aluminum, glass, or paper lids whenever possible. Given that this report synthesizes data from a number of discrete studies that were not uniform in design or goals, the individual methods applied in each case follow.
Water column MP (GBE)
Preserved material from water column tows was thoroughly mixed and 3-5 μm filtered (47 mm PTFE; Sigma-Aldrich, USA) in a glass funnel system. Biofilms and organic matter were removed by stoppering the funnel and adding a 1:1 mixture of KOH (30%) with NaClO (14%, Alfa Aesar) to the filter and holding at
Microplastic particle counts were estimated initially using a fluorescent staining and automated imaging approach. Confocal microscopy was performed by scanning filters (or portions thereof) using excitation: 487 nm and emission: 525 nm for Nile Red and (only for the HSE samples - see below) excitation: 409 and emission: 450 nm for DAPI using a Nikon A1R HD according to standard methods described by[10,49,54,56,58]. Depending on the number of particles, either whole, half, or one-quarter of each filter was scanned and the images stitched together to create a composite. Automated image analysis of the full scan was accomplished using the NIS-Elements program (Nikon Instruments Inc., USA).
Following confocal analysis, filters from representative samples (samples across years, sites, and with both high and low MP counts) were handled according to the manufacturer’s recommendation to remove MP and analyze them by LDIR. The prior filters were placed in a glass beaker with 2 mL of 70% ethanol and stirred for 15 min to release MP particles into solution. The solution containing MP was introduced into Fastwells™ reagent barrier (Grace Bio-labs, Bend, Oregon, USA) attached to a Kevley MirrIR™ slide (Kevley Technology, Chesterfield, Ohio, USA), covered with a petri dish, and allowed to dry completely. Prepared slides were marked with a black marker along the horizontal edge to improve sample flatness detection prior to automated infrared analysis of count, size, shape, and elemental composition using the Agilent 8700 LDIR[59,60] and the supplied Microplastics Starter spectral library[61] using Clarify™ software. The library contained a default set of > 50 polymer IR spectra plus spectra of biogenic particles such as coal, chitin, and rubber, and spectra of other particle types that might typically be present in an environmental sample, e.g., silica. Counts of MP in these paired samples were analyzed to determine concurrence of the two methods.
Surface water and bulk sample MP (HSE and GME)
Manta trawl samples were wet sieved through stacked 5 and 0.3 (GME) or 0.1 mm (HSE) stainless-steel mesh sieves using diH2O. Particles remaining on the 5 mm sieve were discarded. Solids collected on the smallest sieve were transferred to a pre-weighed clean glass beaker, covered with aluminum foil, and placed in a drying oven set to 60 °C until completely dry. Due to high amounts of organic matter, samples with more than 15% settled visual organic matter were sub-sampled prior to sieving. Once dry, organic material was eliminated using 30% hydrogen peroxide (30% H2O2, LabChem, USA) at 60 °C[46]. Following digestion, samples with sand or sediment were density separated using a ZnCl2 solution (1.5 g·cm-1; Fisher Scientific, USA)[62]. Following digestion and density separation, the remaining sample was vacuum filtered onto 5 µm PTFE filters. GME samples were visually assessed using light microscopy while HSE samples were stained for confocal microscopy. Bulk surface-water samples were immediately filtered onto 5 µm PTFE filters and peroxide digested (op. cit.). Subsampling and density separation was not performed for bulk grabs as sand and sediment levels did not require this step.
Samples from HSE were subjected to confocal imaging following the same Nile Red staining process described for GBE, after which MP in surface water and bulk samples were immediately incubated with DAPI (1 mg·mL-1, Sigma-Aldrich, USA)[63] for another 30 min in the dark to counter-stain. This procedure identified putative MP as those reflecting strongly for Nile Red (mean reflectance < 850 nm) but not reflecting strongly for DAPI (max reflectance < 170 nm). Identified particles were counted, measured, and reflectance noted. Samples representing sites and MP count ranges were selected and processed for LDIR analysis as described for the GBE water samples. The LDIR spectra were compared to a library containing 8 different polymer spectra. Samples from GME were analyzed using light microscopy only to identify MP by type and color, and were not subject to confocal or LDIR analysis.
Sediment core processing
Sediment cores were separated into 2 cm segments, dried, homogenized[10], and 1 µm sieved using filtered diH2O. Filtrates were again dried, digested over two days using wet peroxide at 60 °C[46], and vacuum filtered onto a 5 µm PTFE filter, after which particles were manually identified using light microscopy. Samples containing a large amount of material following digestion were density separated using 25 mL of ZnCl2 solution (1.5 g·cm-1; Fisher Scientific, USA)[62]. Floating solids were collected by vacuum filtration onto a
Statistical treatments
Normal distribution of data was tested by Shapiro-Wilk normality test. Kruskal-Wallis test was performed to investigate ambient MP concentration in all sample types and the P-values were adjusted by Bonferroni correction. Data derived from confocal and LDIR analyses were compared using paired two-sample t-test for means. All tests were performed in R.
RESULTS
Across the 179 GBE water column samples analyzed, the range of numbers of MP detected was
Figure 2. Overall variability in MP counts in water column samples from Great Bay Estuary shown by (A) location sampled; (B) year sampled; and (C) month. Significance level indicated by “*”. MP: Microplastics.
Figure 3. (A) Temporal and (B) spatial variation in MP counts from water column samples collected in GBE. Significance level indicated by “*”. GBE: Great Bay Estuary; MP: microplastics.
Figure 4. Spectrally determined (LDIR) elemental composition of MP detected in representative water samples from Great Bay Estuary. Panels show data for (A) Nannie Island August 2020; (B) Nannie Island April 2021; (C) Oyster River July 2020; and (D) Adams Point October 2020. LDIR: Laser direct infrared spectrometry; MP: microplastics.
Across the 14 manta water samples from HSE, the range of MP counts was 0.45-30 MP particles m-3, with a mean of 11 ± 9 (median 8) MP particles m-3. For the 72 bulk water samples from HSE, the range of numbers of MP detected was 0-143,763 MP particles m-3, with a mean of 27,690 ± 29,860 (median 10,927) MP particles m-3. As observed in GBE, the majority of particles observed in HSE waters were very small, around 50 μm [Figure 5]. Elemental morphology of MP in these water samples also was highly variable generally showing representatives of at least eight polymer types. Bulk grabs in HSE contained significantly more MP per volume than those collected with a manta trawl net. Similar findings have been noted across a range of MP studies comparing discrete grabs with manta trawls[47,66-69]. This outcome can be explained by differences in methodology; 300 mm trawl mesh captures only a subset of MP present, whereas bulk grabs of water capture the full size-range of MP[47,70]. When comparing particle abundance derived from nets only (manta trawl in HSE versus plankton net in GBE), far fewer MP were observed in HSE than in GBE waters, very nearly 1,000× fewer particles. Those differences were most likely due to the hydrology of HSE, which has greater ocean influence and flow in/out. It also is possible that differences in particle density could account for lower MP abundance in the HSE surface sampling approach (top 0-0.2 m of the water column), vs. the slightly deeper water column GBE sampling (~0.3 m below the surface). Minor differences could be due to differences in spectral libraries used for LDIR, with the library used for the HSE samples having a smaller number of polymer types (8 vs. > 50 used for the GBE samples). The GME water samples, being analyzed only via light microscopy, yielded many fewer MP than the samples from other studies that were subjected to confocal fluorescent analysis. Across the 42 GME surface water trawl samples, the range of MP detected was 0.05-5 MP particles m-3, with a mean of 1 ± 0.2 (median 0.6) MP particles m-3. Significant variation was observed across GME sites (P = 0.002).
Figure 5. Physical parameters of MP collected in different estuarine water systems of New England using three types of water samplers, all estimated using a confocal microscopic analysis. Manta and bulk sample types were from Hampton-Seabrook Estuary only. Net samples were from Great Bay Estuary. MP: Microplastics.
The numbers of MP vary considerably among each of these New England habitats. This is not surprising given the differences in methodologies, hydrology, and particular geographies[71]. The MP counts also are well-within the ranges reported for many other estuaries around the globe [Table 2].
Microplastic counts in selected estuaries
Sediment location | MP count (max particles m-3) | Ref. |
Hampton-Seabrook Estuary (USA) | 143,763 | This study |
Great Bay Estuary (USA) | 4,528 | This study |
Great Marsh Estuary (USA) | 5 | This study |
Chesapeake Bay (USA) | 0.2 | Bikker et al. 2020[72] |
Delaware Bay (USA) | 1 | Cohen et al. 2019[73] |
Estuary (India) | 752 | Suresh et al. 2020[74] |
Estuary (China) | 4,137 | Zhao et al. 2014[75] |
Estuary (Australia) | 172 | Hitchcock et al. 2019[76] |
Estuary (Mauritius) | 412,000 | Ragoobur et al. 2023[77] |
As the study of MP progresses and expands, so do the methods utilized. The fortunate application of both confocal and LDIR analysis for many of the samples accessed in this survey provided for comparison of MP counts derived from both methodologies at two sites and using three sampling methods. In every case, LDIR microscopy yielded more particles than was obtained by confocal microscopy. For samples collected with plankton nets, MP were underestimated by a factor 5× when analyzed by confocal. For bulk samples, MP were undercounted by confocal microscopy by a factor of 6×. Samples collected by manta trawl were undercounted by a factor of 10×.
Across the eight salt marsh locations tested for MP sequestration, there were significant differences in the counts of MP·kg-1 (χ2 = 6.8, df = 7, P = 3 × 10-6). The majority of MP (60%-70%) found in HSE sediments were in the top 4 cm [low marsh (19,800 ± 6,900 kg-1), high marsh (13,900 ± 2,800 kg-1)]. More MP particles were found in HSE low marsh sediment samples (16,400 ± 3,900 kg-1 dry weight) than were found in high marsh samples (10,600 ± 1,500 kg-1), which floods less frequently than the low marsh on a lunar tidal cycle. The difference translated to 60%-70% more MP particles, but due to high variation among samples this difference was not significant (P = 0.45) [Figure 6]. The absolute numbers of MP detected in HSE marsh sediments were within the typical ranges of many other estuarine MP burdens [Table 3] and were an order of magnitude less than the numbers of MP found in GBE sediments[10].
Figure 6. MP found at eight locations in coastal marsh sediments of Hampton-Seabrook Estuary, each subsampled at high and low marsh elevations. MP: Microplastics.
Selected studies of microplastic counts in some coastal sediments
Sediment location | MP count (max particles kg-1) | Ref. |
Hampton-Seabrook Estuary (USA) | 20,000 | This study |
Great Bay Estuary (USA) | 675,000 | Cheng et al. 2021[10] |
Narragansett Bay (USA) | 13,000 | Fulfer and Walsh 2023[78] |
Tampa Bay (USA) | 290 | McEachern et al. 2019[66] |
Coastal (Belgium) | 213 | Claessens et al. 2011[79] |
Estuary (China) | 340 | Peng et al. 2017[1] |
Salt marsh (Portugal) | 23 | Cozzolino et al. 2020[80] |
Mangrove (Singapore) | 63 | Nor et al. 2014[81] |
Lagoon (Italy) | 2,175 | Vianello et al. 2013[82] |
Estuary (Mauritius) | 135 | Ragoobur et al. 2023[77] |
Beach (Germany) | 62,100 | Liebezeit et al 2012[83] |
DISCUSSION
Use of fluorescent staining is popular for MP studies because it is inexpensive and can be accomplished with less sophisticated microscopes. This study illustrates the ease with which hundreds of samples can be analyzed by that method but by no means is an endorsement of one approach over another. A caveat is that the numbers of particles can be greatly underestimated by staining for reasons that are well-documented, namely variations in handling (time and temperature of incubation with the stain[84]) and also the lower staining intensity of many polymers. Infrared spectral analysis of representative samples that previously were analyzed with Nile Red confocal fluorescence analysis provided a precise and accurate means to confirm size and shape analyses from the less stringent procedure and provided estimates of the proportional elemental composition of MP from waters and sediments.
Many of the data recounted here were made possible because samples for other (non-MP) studies were archived long ago and still were available. Because the studies took place over multiple years and by investigators in different laboratories, there was variation in the methodologies used to assess MP. Many water samples were collected using a plankton net whereas some other samples were collected using a manta trawl, also constructed of gradient mesh material. It is important to note that net sampling in estuarine waters is nearly always affected to some degree by the ambient levels of organic material, which can vary rapidly and greatly. Mesh can clog with phytoplankton chains, resuspended sediments, and gelatinous creatures such as ctenophonres, cnidaria, and organic debris (i.e., plant material and wrack, especially in estuarine waters). When nets become clogged, particles smaller than the actual mesh size are entrained and maintained in samples. For this reason, grab samples also were utilized in the recent HSE sampling. Furthermore, the increasing accessibility of high-throughput infrared analysis allowed improvement over time in the analysis of plastic type. This allowed the finding that although confocal analysis of Nile Red stained particles is a reasonable method to begin a survey, the LDIR is consistently a more valuable method because it identifies particles that are not effectively stained and the LDIR provides a definitive morphology. In the future, as sampling continues to evolve with apparatus such as the MuMi[85], more precise data can be obtained. Despite the limitations of net mesh sampling, this set of studies provides a remarkably consistent baseline for the presence and types of MP in these New England estuaries.
There are a variety of environmental and anthropogenic factors that may influence how MP enter, circulate, and alternatively are sequestered or exit an estuarine system. Variation in watershed size, number of tributaries, water depth, geomorphology, hydrology and tidal energy, and presence intertidal and subtidal coastal habitats can influence MP presence, as can human population densities, amounts and proximity of impervious surface, and the abundance of associated infrastructure. Precipitation events have been tied to increased surface-water MP abundance[86-88], with higher numbers in nearshore waters compared to offshore waters likely due to land-based runoff[87]. Tides have also been found to impact the distribution of MP, with greater amounts of plastic litter found on the outgoing tidal phase[89] and larger MP particles correlated to the spring and flood tidal cycle[90]. Coastal flooding may have a similar impact as precipitation on transporting land-based MP into estuarine environments[86-88]. With increasing storm events and associated surge, flooding events may resuspend and force stranded near-shore marine MP deeper into the estuary[91].
GBE is surrounded by rural, agricultural, and forested areas and is fed by seven tributaries that typically are low-discharge providing limited freshwater[44]. GBE is unique from other estuaries in that it is well-mixed due to strong currents that introduce oceanic water into the Bay, but flushing rates and residence times are slower/longer than HSE and GME. Although the extent to which the tributaries are the primary source of MPs in GBE is unclear, water resource recovery facilities from more than 50 rural communities in New Hampshire and Maine discharge into GBE. Thus, the MP and other point and non-point source pollutants may be higher in GBE. Despite being well-mixed overall, most of the stations in GBE that were sampled are a considerable distance from the estuary’s outlet at the mouth of the Piscataqua River. Various authors suggest the flushing time for Great Bay is between 2 and 30 days[64,92,93], depending on the tide and river discharge states involved. The most recent estimate of residence time for Great Bay is between 5 and
To our knowledge, quantitative estimates of flushing time have not been published for the HSE, nor were any calculated estimates found in grey literature. However, recognizing that 88% of the water in the estuary is exchanged on each tide and 6% of the ebb tide plume returns to the estuary on the next tidal cycle based on estimates from Public Systems of New Hampshire[95], the flushing time is estimated to be less than one day[96], which is considerably faster than GBE. Although there are no published residence time estimates for HSE and GME, both estuaries have a more direct connection to coastal waters and have higher flushing rates despite the considerable watershed areas and associated population densities. GBE is the smallest of the three estuarine systems studied here at an estimated area of 200 ha with relatively low population density, yet it had orders of magnitude greater MP per unit volume than the significantly larger HSE
The uniquely different geomorphology of GBE as a post-glacial drowned river valley landform may play an important role. GBE lacks the extent of broad fringing salt marsh that buffer the waterways in HSE and GME. Unlike the shallow barrier-beach systems of HSE and GME that are flanked by hundreds of hectares of salt marsh[97,98], terrestrial runoff may be washing directly into the waters of GBE with little to no natural filtration through high and low marsh habitats along much of its extent. The salt marshes in GBE are limited to relatively small, narrow and disparate patches that do not extend far seaward from upland shores and many are struggling to keep pace with sea level rise[99]. As a result, GBE is expected to have far less capacity to eliminate MP through sequestration in vegetated wetlands given significantly less acreage of fringing saltmarsh and riparian buffer zones than the barrier beach marsh complexes of HSE and GME. In fact, the high levels of MP in GBE waters have little destiny other than to settle. This corroborates the higher sediment levels of MP in GBE[10] than in GME (current data).
The HSE sediment MP were dramatically lower than found for GBE sediments[10]. Although higher MP concentrations have been correlated with population density[86,100], their presence also can be influenced by point sources such as water resource recovery facilities[101,102]. These facilities are located on two of the seven tributaries to GBE, just upstream of several sampling areas. It is possible that these potential MP point sources, coupled with slower (and lower) tidal flushing rates and diminished ability to sequester MP in fringing salt marsh sediments, could be collectively contributing to the greater abundance of MP in GBE waters as compared to HSE and GME. Similarly, the greater MP in GBE subtidal sediment (100 ± 50 particles g-1)[10] as compared to HSE intertidal marsh peat cores (2.3 ± 0.3 particles g-1) may be attributed to lower flush rates and longer residence times allowing denser MP to settle. Together, the detection and comparisons of MP in these New England estuarine waters and sediments illustrate that baseline MP will vary based on anthropogenic impacts (e.g., water treatment methods), hydrology (e.g., estuary type and circulation), and habitat type (e.g., subtidal mudflats vs. intertidal salt marshes).
CONCLUSIONS
This work illustrates that the occurrence and fate of MP in the New England estuarine habitats studied vary widely and are generally comparable to the broad ranges of MP identified by other published studies of estuarine waters and sediments. These water and intertidal sediment data complement the only other published study on MP in this geographic area, a study of MP in bottom sediments of GBE[10]. The data are essential to populate new hydrological models that are expected to help trace the sources of MP and other pollutants in GBE. The results provided here are of immediate importance and value to resource users, harvesters, aquaculturists, and managers to inform and evaluate contaminant risks, to choose low MP sites for their activities, and provide information on the time of year with the highest or lowest MP concentrations.
DECLARATIONS
Acknowledgments
Thanks are due to other members of the Brown Lab Team: Kelsey Meyer, Alyssa Stasse, Emily Williams, Kennedy McGrath, and Grant Milne for helping to collect, preserve, and analyze samples. Alyssa Stasse created the maps. Thanks are also due to members of the Moore Lab Team: Kayla Tozier, Grant McKown, Creightanya Brewley, and Emily Dutton who helped collect field samples in HSE. Peter Phippen and Geoff Walker collected GME trawl samples. Kariana Ebel assisted in sample processing. David Shay provided assistance with vessels, nets, and flow meter calibration. Krystin Ward allowed access to one of the sites.
Authors’ contributions
Conceptualization: Brown B, Moore G
Methodology: Brown B, Mogensen H, Sims-Harper T, Gibson J, Jarrett G, Wardinski C
Data analysis: Lee BY, Brown B, Mogensen H, Gibson J
Investigation: Sims-Harper T, Brown B, Jarrett G, Wardinski C
Original manuscript preparation: Brown B, Moore G, Gibson J
Authors have read and agreed to the submitted version of the manuscript.
Availability of data and materials
Not applicable.
Financial support and sponsorship
UNH School of Marine Sciences and Ocean Engineering provided fellowship funding to Sims-Harper T and Mogensen H. The College of Life Sciences and Agriculture and NHAES Hatch 1023564 (nh00697) provided funding to Brown B. New Hampshire Sea Grant research project R/RCE-3, pursuant to National Oceanic and Atmospheric Administration Award No. NA18OAR4170090, and The Merrimack Valley Planning Commission provided funding to Moore G.
Conflicts of interest
All authors declared that there are no conflicts of interest.
Ethical approval and consent to participate
Not applicable.
Consent for publication
Not applicable.
Copyright
© The Author(s) 2023.
REFERENCES
1. Peng J, Wang J, Cai L. Current understanding of microplastics in the environment: occurrence, fate, risks, and what we should do. Integr Environ Assess Manag 2017;13:476-82.
3. Carpenter EJ, Anderson SJ, Harvey GR, Miklas HP, Peck BB. Polystyrene spherules in coastal waters. Science 1972;178:749-50.
4. Gregory MR. Accumulation and distribution of virgin plastic granules on New Zealand beaches. N Z J Mar Freshwater Res 1978;12:399-414.
5. Gregory MR. Virgin plastic granules on some beaches of Eastern Canada and Bermuda. Mar Environ Res 1983;10:73-92.
6. Winston JE. Drift plastic - an expanding niche for a marine invertebrate? Mar Pollut Bull 1982;13:348-51.
7. Cheng L. Marine insects. 1976. Available from: https://escholarship.org/uc/item/1pm1485b. [Last accessed on 29 Nov 2023].
8. Barboza LGA, Dick Vethaak A, Lavorante BRBO, Lundebye AK, Guilhermino L. Marine microplastic debris: an emerging issue for food security, food safety and human health. Mar Pollut Bull 2018;133:336-48.
9. Dodhia MS, Rogers KL, Fernández-juárez V, et al. Microbe-mineral interactions in the Plastisphere: coastal biogeochemistry and consequences for degradation of plastics. Front Mar Sci 2023;10:1134815.
10. Cheng MLH, Lippmann TC, Dijkstra JA, et al. A baseline for microplastic particle occurrence and distribution in Great Bay Estuary. Mar Pollut Bull 2021;170:112653.
11. Seeley ME, Song B, Passie R, Hale RC. Microplastics affect sedimentary microbial communities and nitrogen cycling. Nat Commun 2020;11:2372.
12. Li J, Huang W, Xu Y, Jin A, Zhang D, Zhang C. Microplastics in sediment cores as indicators of temporal trends in microplastic pollution in Andong salt marsh, Hangzhou Bay, China. Reg Stud Mar Scie 2020;35:101149.
13. Lloret J, Pedrosa-Pamies R, Vandal N, et al. Salt marsh sediment act as sinks for microplastics and reveal effects of current and historical land use changes. Environ Adv 2021;4:100060.
14. Carson HS, Nerheim MS, Carroll KA, Eriksen M. The plastic-associated microorganisms of the North Pacific Gyre. Mar Pollut Bull 2013;75:126-32.
15. Jokiel PL. Long-distance dispersal by rafting: reemergence of an old hypothesis. Endeavour 1990;14:66-73.
17. Zettler ER, Mincer TJ, Amaral-Zettler LA. Life in the “plastisphere”: microbial communities on plastic marine debris. Environ Sci Technol 2013;47:7137-46.
18. Barnes D, Fraser K. Rafting by five phyla on man-made flotsam in the Southern Ocean. Mar Ecol Prog Ser 2003;262:289-91.
19. Barnes DK. Natural and plastic flotsam stranding in the Indian Ocean. In: Davenport J, Davenport JL, editors. The effects of human transport on ecosystems: cars and planes, boats and trains. Dublin: Royal Irish Academy; 2004. p. 193-205. Available from: https://nora.nerc.ac.uk/id/eprint/16343/. [Last accessed on 29 Nov 2023].
20. Masó M, Garcés E, Pagès F, Camp J. Drifting plastic debris as a potential vector for dispersing Harmful Algal Bloom (HAB) species. Sci Mar 2003;67:107-11.
21. Reisser J, Shaw J, Hallegraeff G, et al. Millimeter-sized marine plastics: a new pelagic habitat for microorganisms and invertebrates. PLoS One 2014;9:e100289.
22. Thompson RC, Olsen Y, Mitchell RP, et al. Lost at sea: where is all the plastic? Science 2004;304:838.
23. Plastic rafting: the invasive species hitching, a ride on ocean litter. Available from: https://www.theguardian.com/environment/2021/jun/14/plastic-rafting-the-invasive-species-hitching-a-ride-on-ocean-litter. [Last accessed on 29 Nov 2023].
24. McCormick A, Hoellein TJ, Mason SA, Schluep J, Kelly JJ. Microplastic is an abundant and distinct microbial habitat in an urban river. Environ Sci Technol 2014;48:11863-71.
25. Urbanek AK, Rymowicz W, Mirończuk AM. Degradation of plastics and plastic-degrading bacteria in cold marine habitats. Appl Microbiol Biotechnol 2018;102:7669-78.
26. Rogers KL, Carreres-calabuig JA, Gorokhova E, Posth NR. Micro-by-micro interactions: how microorganisms influence the fate of marine microplastics. Limnol Oceanogr Lett 2020;5:18-36.
27. Gregory MR. Environmental implications of plastic debris in marine settings--entanglement, ingestion, smothering, hangers-on, hitch-hiking and alien invasions. Philos Trans R Soc Lond B Biol Sci 2009;364:2013-25.
28. Browne MA, Dissanayake A, Galloway TS, Lowe DM, Thompson RC. Ingested microscopic plastic translocates to the circulatory system of the mussel, mytilus edulis (L.). Environ Sci Technol 2008;42:5026-31.
29. Cole M, Lindeque P, Fileman E, Halsband C, Galloway TS. The impact of polystyrene microplastics on feeding, function and fecundity in the marine copepod calanus helgolandicus. Environ Sci Technol 2015;49:1130-7.
30. Al-Sid-Cheikh M, Rowland SJ, Stevenson K, Rouleau C, Henry TB, Thompson RC. Uptake, whole-body distribution, and depuration of nanoplastics by the scallop pecten maximus at environmentally realistic concentrations. Environ Sci Technol 2018;52:14480-6.
31. Watts AJ, Lewis C, Goodhead RM, et al. Uptake and retention of microplastics by the shore crab carcinus maenas. Environ Sci Technol 2014;48:8823-30.
32. Farthing J, Laing I, Dore WJ. Depuration conditions for great scallops (Pecten maximus). J Shellfish Res 2003;22:409-14. Available from: https://api.semanticscholar.org/CorpusID:82533626. [Last accessed on 29 Nov 2023]
33. Canniff PM, Hoang TC. Microplastic ingestion by Daphnia magna and its enhancement on algal growth. Sci Total Environ 2018;633:500-7.
34. Sussarellu R, Suquet M, Thomas Y, et al. Oyster reproduction is affected by exposure to polystyrene microplastics. Proc Natl Acad Sci U S A 2016;113:2430-5.
35. Markham E, Brault EK, Khairy M, et al. Time trends of polybrominated diphenyl ethers (PBDEs) in Antarctic biota. ACS Omega 2018;3:6595-604.
36. Dawson AL, Kawaguchi S, King CK, et al. Turning microplastics into nanoplastics through digestive fragmentation by Antarctic krill. Nat Commun 2018;9:1001.
37. Suckling CC. Responses to environmentally relevant microplastics are species-specific with dietary habit as a potential sensitivity indicator. Sci Total Environ 2021;751:142341.
38. Biamis C, Driscoll KO, Hardiman G. Microplastic toxicity: a review of the role of marine sentinel species in assessing the environmental and public health impacts. Case Stud Chem Environ Eng 2021;3:100073.
39. Smith M, Love DC, Rochman CM, Neff RA. Microplastics in seafood and the implications for human health. Curr Environ Health Rep 2018;5:375-86.
40. Dehaut A, Cassone AL, Frère L, et al. Microplastics in seafood: benchmark protocol for their extraction and characterization. Environ Pollut 2016;215:223-33.
41. Rochman CM, Tahir A, Williams SL, et al. Anthropogenic debris in seafood: Plastic debris and fibers from textiles in fish and bivalves sold for human consumption. Sci Rep 2015;5:14340.
42. Kroon FJ, Motti CE, Jensen LH, Berry KLE. Classification of marine microdebris: a review and case study on fish from the Great Barrier Reef, Australia. Sci Rep 2018;8:16422.
43. Laursen SN, Fruergaard M, Dodhia MS, et al. Settling of buoyant microplastic in estuaries: the importance of flocculation. Sci Total Environ 2023;886:163976.
44. Short FT. The ecology of the Great Bay Estuary, New Hampshire and Maine: an estuarine profile and bibliography. PREP Reports & Publications; 1992. p. 222. Available from: https://scholars.unh.edu/prep/376/. [Last accessed on 29 Nov 2023].
45. Stasse A, MLH Cheng, K Meyer, et al. Temporal dynamics of eastern oyster, larval abundance in Great Bay Estuary, New Hampshire. J Shellfish Res 2022;40:471-8.
46. Masura J, Baker J, Foster G, Arthur C. Laboratory methods for the analysis of microplastics in the marine environment: recommendations for quantifying synthetic particles in waters and sediments. Available from: https://repository.oceanbestpractices.org/handle/11329/1076. [Last accessed on 29 Nov 2023].
47. Hung C, Klasios N, Zhu X, Sedlak M, Sutton R, Rochman CM. Methods matter: methods for sampling microplastic and other anthropogenic particles and their implications for monitoring and ecological risk assessment. Integr Environ Assess Manag 2021;17:282-91.
48. Brander SM, Renick VC, Foley MM, et al. Sampling and quality assurance and quality control: a guide for scientists investigating the occurrence of microplastics across matrices. Appl Spectrosc 2020;74:1099-125.
49. Miller E, Sedlak M, Lin D, et al. Recommended best practices for collecting, analyzing, and reporting microplastics in environmental media: lessons learned from comprehensive monitoring of San Francisco Bay. J Hazard Mater 2021;409:124770.
50. Zhu X, Munno K, Grbic J, et al. Holistic assessment of microplastics and other anthropogenic microdebris in an urban bay sheds light on their sources and fate. ACS EST Water 2021;1:1401-10.
51. Pasquier G, Doyen P, Kazour M, et al. Manta net: the golden method for sampling surface water microplastics in aquatic environments. Front Environ Sci 2022;10:811112.
52. Payne AR, Burdick DM, Moore GE, Wigand C. Short-term effects of thin-layer sand placement on salt marsh grasses: a marsh organ field experiment. J Coast Res 2021;37:771-8.
53. Enders K, Lenz R, Beer S, Stedmon CA, Browman H. Extraction of microplastic from biota: recommended acidic digestion destroys common plastic polymers. ICES J Mar Sci 2017;74:326-31.
54. Maes T, Jessop R, Wellner N, Haupt K, Mayes AG. A rapid-screening approach to detect and quantify microplastics based on fluorescent tagging with Nile Red. Sci Rep 2017;7:44501.
55. Shim WJ, Song YK, Hong SH, Jang M. Identification and quantification of microplastics using Nile Red staining. Mar Pollut Bull 2016;113:469-76.
56. Erni-Cassola G, Gibson MI, Thompson RC, Christie-Oleza JA. Lost, but found with nile red: a novel method for detecting and quantifying small microplastics (1 mm to 20 μm) in environmental samples. Environ Sci Technol 2017;51:13641-8.
57. Prata JC, da Costa JP, Duarte AC, Rocha-santos T. Methods for sampling and detection of microplastics in water and sediment: a critical review. TrAC Trends Analyt Chem 2019;110:150-9.
58. Scircle A, Cizdziel JV, Tisinger L, Anumol T, Robey D. Occurrence of microplastic pollution at oyster reefs and other coastal sites in the Mississippi sound, USA: impacts of freshwater inflows from flooding. Toxics 2020;8:35.
59. Bäuerlein PS, Pieke EN, Oesterholt FIHM, Ter Laak T, Kools SAE. Microplastic discharge from a wastewater treatment plant: long term monitoring to compare two analytical techniques, LDIR and optical microscopy while also assessing the removal efficiency of a bubble curtain. Water Sci Technol 2023;87:39-56.
60. Nizamali J, Mintenig SM, Koelmans AA. Assessing microplastic characteristics in bottled drinking water and air deposition samples using laser direct infrared imaging. J Hazard Mater 2023;441:129942.
61. Whiting QT, O'Connor KF, Potter PM, Al-Abed SR. A high-throughput, automated technique for microplastics detection, quantification, and characterization in surface waters using laser direct infrared spectroscopy. Anal Bioanal Chem 2022;414:8353-64.
62. Coppock RL, Cole M, Lindeque PK, Queirós AM, Galloway TS. A small-scale, portable method for extracting microplastics from marine sediments. Environ Pollut 2017;230:829-37.
63. Stanton T, Johnson M, Nathanail P, Gomes RL, Needham T, Burson A. Exploring the efficacy of nile red in microplastic quantification: a costaining approach. Environ Sci Technol Lett 2019;6:606-11.
64. Brown WS, Arellano E. The application of a segmented tidal mixing model to the Great Bay Estuary, N. H. Estuaries 1980;3:248-57.
65. Neto JA, Gaylarde C, Beech I, Bastos AC, da Silva Quaresma V, de Carvalho DG. Microplastics and attached microorganisms in sediments of the Vitória bay estuarine system in SE Brazil. Ocean Coast Manag 2019;169:247-53.
66. McEachern K, Alegria H, Kalagher AL, Hansen C, Morrison S, Hastings D. Microplastics in Tampa Bay, Florida: abundance and variability in estuarine waters and sediments. Mar Pollut Bull 2019;148:97-106.
67. Song YK, Hong SH, Jang M, et al. A comparison of microscopic and spectroscopic identification methods for analysis of microplastics in environmental samples. Mar Pollut Bull 2015;93:202-9.
68. Barrows APW, Cathey SE, Petersen CW. Marine environment microfiber contamination: global patterns and the diversity of microparticle origins. Environ Pollut 2018;237:275-84.
69. Covernton GA, Pearce CM, Gurney-Smith HJ, et al. Size and shape matter: a preliminary analysis of microplastic sampling technique in seawater studies with implications for ecological risk assessment. Sci Total Environ 2019;667:124-32.
70. Cutroneo L, Reboa A, Besio G, et al. Microplastics in seawater: sampling strategies, laboratory methodologies, and identification techniques applied to port environment. Environ Sci Pollut Res Int 2020;27:8938-52.
71. Biltcliff-ward A, Stead JL, Hudson MD. The estuarine plastics budget: a conceptual model and meta-analysis of microplastic abundance in estuarine systems. Estuar Coast Shelf Sci 2022;275:107963.
72. Bikker J, Lawson J, Wilson S, Rochman CM. Microplastics and other anthropogenic particles in the surface waters of the Chesapeake Bay. Mar Pollut Bull 2020;156:111257.
73. Cohen JH, Internicola AM, Mason RA, Kukulka T. Observations and simulations of microplastic debris in a tide, wind, and freshwater-driven estuarine environment: the Delaware Bay. Environ Sci Technol 2019;53:14204-11.
74. Suresh A, Vijayaraghavan G, Saranya KS, Neethu KV, Aneesh B, Bijoy Nandan S. Microplastics distribution and contamination from the Cochin coastal zone, India. Reg Stud Mar Sci 2020;40:101533.
75. Zhao S, Zhu L, Wang T, Li D. Suspended microplastics in the surface water of the Yangtze Estuary System, China: first observations on occurrence, distribution. Mar Pollut Bull 2014;86:562-8.
76. Hitchcock JN, Mitrovic SM. Microplastic pollution in estuaries across a gradient of human impact. Environ Pollut 2019;247:457-66.
77. Ragoobur D, Amode NS, Somaroo GD, Nazurally N. Microplastics in estuarine water and sediment in Mauritius. Reg Stud Mar Sci 2023;57:102766.
78. Fulfer VM, Walsh JP. Extensive estuarine sedimentary storage of plastics from city to sea: Narragansett Bay, Rhode Island, USA. Sci Rep 2023;13:10195.
79. Claessens M, De Meester S, Van Landuyt L, De Clerck K, Janssen CR. Occurrence and distribution of microplastics in marine sediments along the Belgian coast. Mar Pollut Bull 2011;62:2199-204.
80. Cozzolino L, Nicastro KR, Zardi GI, de Los Santos CB. Species-specific plastic accumulation in the sediment and canopy of coastal vegetated habitats. Sci Total Environ 2020;723:138018.
81. Nor NH, Obbard JP. Microplastics in Singapore's coastal mangrove ecosystems. Mar Pollut Bull 2014;79:278-83.
82. Vianello A, Boldrin A, Guerriero P, et al. Microplastic particles in sediments of Lagoon of Venice, Italy: first observations on occurrence, spatial patterns and identification. Estuar Coast Shelf Sci 2013;130:54-61.
83. Liebezeit G, Dubaish F. Microplastics in beaches of the East Frisian islands Spiekeroog and Kachelotplate. Bull Environ Contam Toxicol 2012;89:213-7.
84. Lv L, Qu J, Yu Z, et al. A simple method for detecting and quantifying microplastics utilizing fluorescent dyes - Safranine T, fluorescein isophosphate, Nile red based on thermal expansion and contraction property. Environ Pollut 2019;255:113283.
85. Montoto-Martínez T, Meléndez-Díez C, Melián-Ramírez A, Hernández-Brito JJ, Gelado-Caballero MD. Comparison between the traditional Manta net and an innovative device for microplastic sampling in surface marine waters. Mar Pollut Bull 2022;185:114237.
86. Yonkos LT, Friedel EA, Perez-Reyes AC, Ghosal S, Arthur CD. Microplastics in four estuarine rivers in the Chesapeake Bay, U.S.A. Environ Sci Technol 2014;48:14195-202.
87. Lattin GL, Moore CJ, Zellers AF, Moore SL, Weisberg SB. A comparison of neustonic plastic and zooplankton at different depths near the southern California shore. Mar Pollut Bull 2004;49:291-4.
88. Moore CJ, Moore SL, Weisberg SB, Lattin GL, Zellers AF. A comparison of neustonic plastic and zooplankton abundance in southern California's coastal waters. Mar Pollut Bull 2002;44:1035-8.
89. Manickavasagam S, Kumar S, Kumar K, Rathi Bhuvaneswari G, Paul T, Shukla S. Quantitative assessment of influx and efflux of marine debris in a water channel of South Juhu creek, Mumbai, India. Reg Stud Mar Sci 2020;34:101095.
90. Sadri SS, Thompson RC. On the quantity and composition of floating plastic debris entering and leaving the Tamar Estuary, Southwest England. Mar Pollut Bull 2014;81:55-60.
91. Fok L, Cheung PK, Tang G, Li WC. Size distribution of stranded small plastic debris on the coast of Guangdong, South China. Environ Pollut 2017;220:407-12.
92. Bilgili A, Proehl JA, Lynch DR, Smith KW, Swift MR. Estuary/ocean exchange and tidal mixing in a Gulf of Maine Estuary: a Lagrangian modeling study. Estuar Coast Shelf Sci 2005;65:607-24.
93. Gallagher TW, Mancilla C. MEMORANDUM: calibration of Great Bay Estuary hydrodynamic model and incremental nitrogen estimation. Available from: https://scholars.unh.edu/prep/411/. [Last accessed on 29 Nov 2023].
94. Matso K. Flushing time versus residence time for the Great Bay Estuary. Available from: https://scholars.unh.edu/prep/413/. [Last accessed on 29 Nov 2023].
95. Seabrook station environmental report, vol.1 - construction permit. Available from: https://www.nrc.gov/docs/ML1028/ML102880614.html. [Last accessed on 29 Nov 2023].
96. Trowbridge P. Hydrological parameters for New Hampshire’s Estuaries. Available from: https://scholars.unh.edu/prep/130/. [Last accessed on 29 Nov 2023].
97. Eberhardt AL, Burdick DM. Hampton-seabrook estuary restoration compendium. Available from: https://scholars.unh.edu/prep/76/. [Last accessed on 29 Nov 2023].
98. Piscataqua Region Estuaries Partnership. Piscataqua region environmental planning assessment 2015. Available from: https://scholars.unh.edu/prep/358/. [Last accessed on 29 Nov 2023].
99. Payne AR, Burdick DM, Moore GE. Potential effects of sea-level rise on salt marsh elevation dynamics in a New Hampshire Estuary. Estuaries Coast 2019;42:1405-18.
100. Barnes DKA. Remote islands reveal rapid rise of southern hemisphere, sea debris. Sci World J 2005;5:915-21.
101. Browne MA, Crump P, Niven SJ, et al. Accumulation of microplastic on shorelines woldwide: sources and sinks. Environ Sci Technol 2011;45:9175-9.
Cite This Article

How to Cite
Download Citation
Export Citation File:
Type of Import
Tips on Downloading Citation
Citation Manager File Format
Type of Import
Direct Import: When the Direct Import option is selected (the default state), a dialogue box will give you the option to Save or Open the downloaded citation data. Choosing Open will either launch your citation manager or give you a choice of applications with which to use the metadata. The Save option saves the file locally for later use.
Indirect Import: When the Indirect Import option is selected, the metadata is displayed and may be copied and pasted as needed.
About This Article
Special Issue
Copyright
Data & Comments
Data
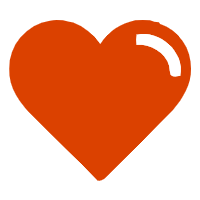
Comments
Comments must be written in English. Spam, offensive content, impersonation, and private information will not be permitted. If any comment is reported and identified as inappropriate content by OAE staff, the comment will be removed without notice. If you have any queries or need any help, please contact us at [email protected].