Integrating resilience in the multi-hazard sustainable design of buildings
Abstract
Recent natural disasters and climate change-induced extremes emphasize the urgent need to enhance the overall resilience of society by addressing the various hazards that buildings may face. Current design approaches recognize the need for integrated risk assessments, but studies primarily focus on existing buildings and single hazards, neglecting the impact of multiple hazards and resilience quantifications. However, it is crucial to consider multi-hazard scenarios and quantify economic, environmental, and resilience losses to pursue effective solutions from the early-stage design of both new buildings and retrofitting interventions. This paper presents a practical multi-criteria approach to support design decisions for enhanced safety, sustainability, and resilience of buildings against earthquakes and heatwaves. The proposed approach is applied to a commercial building with various seismic-resistant and energy-efficient facades. Non-linear seismic assessments are conducted to predict the potential impact concerning repair costs, carbon emissions, and the resilience loss at the design-level earthquake. Additionally, a whole life-cycle analysis and dynamic energy simulations are performed to calculate the financial and carbon losses resulting from power consumption and the ability of the building to maintain energy efficiency under extreme heat. Finally, the study employs a multi-matrix decision-making approach based on integrated economic, environmental, and resilience losses to guide the design selection. The results demonstrate that earthquake-resistant facades can significantly reduce financial losses by over 50%, with seismic resilience playing a crucial role in the final decision. This approach facilitates more effective investment decisions for building projects, enabling the quantification of the effectiveness of integrated strategies in reducing overall potential losses.
Keywords
INTRODUCTION
Recent earthquake disasters, such as the devastating 2023 Turkey and Syria earthquake, have once again highlighted the vulnerability of both existing and modern buildings and infrastructure, underscoring the significant impact of seismic events on the community. Such disasters can result in severe direct losses, including damage to buildings, injury, and loss of life, as well as indirect losses, i.e., reduced productivity and loss of income. These consequences on global economies and societies emphasize the pressing need for enhancing safety and resilience in structures and infrastructures. In addition, the post-earthquake damage has revealed a significant discrepancy between the societal expectations and the reality of the seismic behavior of modern structures[1,2]. It is not enough to focus solely on life-safety criteria when designing new buildings; instead, it is crucial to prioritize resilience and adopt innovative approaches and techniques for earthquake-resistant design and construction. Furthermore, non-structural components such as architectural elements, equipment, and building contents - typically not designed to withstand seismic loading - often contribute the most to economic losses, surpassing even structural losses[3,4]. Due to their poor dynamic performance and seismic detailing, these components can lose functionality even during low-intensity earthquakes and sustain serious damage or collapse during moderate-to-high earthquakes[5]. Consequently, the damage to non-structural components significantly has a profound impact on the functionality of buildings after earthquakes, causing prolonged periods of inactivity, overall losses, and potential life-safety risks for both occupants and pedestrians. As a result, there has been a rise in seismic risk consciousness among individuals, facility managers, and policymakers, who now are seeking high levels of earthquake protection not only for the main load-bearing structure but also for the entirety of the building envelope.
Climate-related extreme events are also increasingly affecting both the economy and society. Understanding their impact and associated risk on the built environment is one of the main challenges that the building construction sector is currently facing. The global annual temperature has increased at an average rate of 0.08 °C per decade since 1880, and the rate has more than doubled to 0.18 °C since 1981[6]. The resulting effects, such as more frequent and intense heatwaves[7], have significant consequences for the environment, human health, and the global economy. In urban areas, buildings and infrastructure are often ill-equipped to cope with high temperatures, leading to significant socio-economic losses. Therefore, it is critical to prioritize the development of structures that can withstand the impacts of climate change. Buildings serve as a critical interface between the external and internal environment, making them highly susceptible to the impacts of climate change, which can manifest in various ways, such as increased energy consumption for heating and cooling, building overheating, and higher annual carbon emissions. For example, in cold climates, buildings can achieve up to a 10% reduction in energy use, while in the tropics, energy use can increase by as much as 20%[8]. Additionally, buildings in temperate climates may experience a shift from heating to cooling energy. Specifically, residents of disadvantaged communities will be affected by extreme heat events, as they often live in older houses with poor insulation, single-pane windows, inefficient lighting, and outdated or inadequate air conditioning equipment, resulting in high operating costs and discomfort[9]. Building owners are thus becoming increasingly interested in the resilience of their existing stock, which should be assessed, accounting for climate variability and considering both gradual and extreme weather scenarios. On the other hand, clients wish to know how resilient the proposed designs may be before making an investment. As a result, it is important for new construction and refurbishment/renovations to prioritize adaptability and resilience throughout the lifespan of the building, with a focus on reducing greenhouse emissions and maintaining comfortable indoor conditions for occupants.
It is, therefore, crucial to consider the potential impact of multiple hazards on buildings and take appropriate measures in design and retrofitting choices to enhance their resilience. This would help to minimize the overall consequences of natural disasters, such as earthquakes and other extreme events such as climate-related heatwaves, thus reducing their devastating impact on the community in terms of economic losses and casualties (mostly due to heatwaves in Europe, especially in the last years - Figure 1).
In recent years, there has been a growing recognition of the need for integrated technical solutions. Research efforts have been dedicated to developing methodologies and cost-effective technologies that support holistic designs or interventions[12,13]. Researchers have been investigating the long-term value of integrated designs and proposing multi-functional interventions to enhance the performance of existing buildings. Some studies have analyzed existing local-to-global building level techniques, combining seismic retrofitting for the primary load-bearing structure with energy interventions for the building envelope[14], while others have explored innovative materials and solutions, such as the use of external double-skin exoskeletons or prefabricated textile capillary-tube panels[15-17]. However, challenges remain in implementing such integrated solutions, and further work is needed to validate them on large-scale buildings. Furthermore, the technical complexity and invasiveness of multi-functional interventions can discourage the adoption of an integrated strategy, causing building owners to opt for eco-only interventions. More research is also required to develop effective solutions applicable to various building types and locations. On the other hand, research endeavors have focused on developing and verifying multi-criteria evaluation frameworks and digital tools. These endeavors are aimed at assessing seismic safety and various other performance dimensions, especially environmental impacts and cost-benefit considerations. Consequently, these tools assist in identifying the most suitable integrated designs[18-21]. While these studies have primarily focused on retrofitting existing buildings, they have demonstrated the promising potential of an integrated approach for improving the safety and sustainability of buildings. However, current integrated design/assessment methods have some limitations. They often concentrate on typical weather scenarios and do not account for the impact of future climate changes or extreme heatwaves. Additionally, they tend to focus on economic and environmental indicators, with limited consideration for resilience quantifications.
Due to this urgent need for improving the resilience of the built environment, studies on resilience assessment are emerging recently. Focusing on seismic resilience, researchers have developed general frameworks for defining, assessing, managing, and enhancing the seismic resilience of buildings[22-25], and practical applications can be found in the literature[26,27]. These studies have centered on exploring solutions to enhance the resilience of existing buildings, which are designed following pre-seismic code regulations and further impacted by natural aging and potential degradation of material properties. Climate resilience is typically defined in terms of thermal resilience, which is assessed by quantifying the overheating risk through performance simulations under probable climate conditions expected during the lifetime of the building[28-30]. Recent years have seen a growing number of research efforts aimed at defining methods for quantifying resilience. For example, Sharifi et al. proposed a set of indicators to assess the thermal resilience of residential buildings, taking into account their ability to maintain comfortable indoor temperatures during heatwaves, energy efficiency, and adaptive capacity[31]. Homaei and Handy[32] defined a multi-phase metric that assesses thermal resilience based on building characteristics (such as envelope and systems) and occupancy. This metric penalizes deviations from thermal performance targets depending on the phase, hazard level, and exposure time of the event. However, when compared to seismic resilience assessment, methods for climate resilience assessment are still in their infancy.
While design methods are continuously developing and research efforts are growing in different disciplines, there is still a need for integrated procedures for building resilience to multiple hazards. Currently, common design practices often focus on addressing single hazards, not considering real-world local scenarios. On the other hand, research involving multiple hazards often overlooks resilience quantification. When dealing with earthquakes vs. climate-related extremes, existing approaches may not even account for heatwave scenarios. This paper aims to address these research gaps by proposing a practical multi-matrix decision-making procedure that quantifies the expected economic and environmental losses, as well as the resilience of buildings to earthquakes and extreme heatwaves. By offering a simplified approach, this procedure can effectively support the selection of optimal design solutions from the very early-stage design, where most critical design decisions are made. The integrated design approach proposed in this study is comprehensively outlined in Section "RESEARCH METHODOLOGY", while Section "RESULTS" discusses the results of applying this methodology to a case study. Finally, Section "CONCLUSIONS" presents the implications of the research findings and offers recommendations for improved building design and decision-making processes.
RESEARCH METHODOLOGY
This study presents a practical multi-criteria analysis to support early-stage design for increased structural safety, sustainability, and resilience of buildings against earthquakes and heatwaves. An overview of the overall approach is shown in Figure 2 and described in detail below. In line with a Sustainable Structural Design (SSD) approach[33], this study aims to optimize the structural design while minimizing the ecological footprint of the building across its entire lifespan. The analysis evaluates key performance criteria, including financial loss [expected annual losses (EAL)], environmental impact (operational and embodied energy and the impact of post-earthquake damage/repairing during the life cycle of the building), and resilience quantification. These criteria are relevant to stakeholders for managing decisions related to investments and risk mitigation to multiple hazards. Social factors, such as casualties and injuries, are not considered in this study. Although models for assessing the earthquake-related social losses exist, no relevant models can be found that correlate the indoor air temperature of a building to mortality rates/probabilities during heatwaves.
When dealing with the seismic analysis, the Performance-Based Earthquake Engineering (PBEE) procedure[34-36] is employed to quantify the post-earthquake consequences of design solutions. Inputs to the PBEE methodology include building knowledge and location, which are used to perform structural, damage, and loss analyses. The decision variables, such as economic losses, damage-related carbon losses, and business interruption (downtime), can be directly evaluated as a result. These variables are quantified using a time-based approach that involves identifying annualized data to assess the overall environmental-economic loss or potential savings expected in the life cycle of the building. Finally, the assessment of seismic resilience involves further elaboration of the loss results obtained through the PBEE methodology. Fragility functions are used to determine the probability of an asset exceeding defined damage states, thereby facilitating the determination of the extent of functionality drop when a seismic event occurs. The recovery stage of a building following an earthquake disaster is connected to the downtime, which indicates the duration required to attain a specified recovery condition, such as re-occupancy, restoration of pre-earthquake functionality, or complete recovery. By defining the functionality vs. time curve, the seismic resilience loss (RL) resulting from a specific seismic event can be defined[22].
A similar approach can be outlined for the environmental sustainability analysis. Economic losses associated with the annual energy consumptions, accounting for lighting, equipment, heating, and cooling, can be assessed by conducting energy simulations of building systems under typical or extreme weather scenarios. Results from the energy analysis are further elaborated to assess the resilience of the building to the heatwave scenario. In this investigation, RL and resilience indices are evaluated by considering the functionality drop, defined as the capacity to maintain energy efficiency during a heatwave. A whole life-cycle analysis is also conducted to evaluate the expected environmental losses. This enables us to assess the total negative impact (operational energy + embodied energy) of a design solution on the environment, which further increases during extreme climate-related events such as heatwaves.
It is emphasized that an uncertainty-based investigation for the energy/environmental analysis, encompassing uncertainties related to weather, building properties, and design requirements, could be employed to develop a fully integrated probabilistic approach for loss assessment. As demonstrated by Bianchi et al., this approach could provide valuable insights into the reliability and risks associated with specific design solutions, thereby avoiding potentially unconservative loss outcomes that could influence the final investment decision[37]. However, the primary focus of this paper is on the approaches currently used in building design to assess potential losses. Therefore, the typical semi-deterministic approach was employed for the energy analysis.
Integrating seismic, energy, and environmental results can finally offer a comprehensive assessment of the benefits of design solutions or retrofitting interventions. To compare alternative building technologies, both single (hazard-related) and cumulative (multi-hazard) values for economic, environmental, and resilience losses are assessed. This comprehensive approach enables a thorough evaluation of various design options and helps make informed decisions to minimize the overall impact of the building in terms of safety, resilience, and sustainability during its life cycle. A multi-criteria decision-making procedure based on integrated performance-based matrices is proposed to support the comparison. As schematically depicted in Figure 2, the methodology involves the creation of three different matrices based on the simulation outcomes: (i) a cost-based matrix, which integrates seismic repair costs for building components with heatwave-related costs, i.e., the expected energy consumption; (ii) a carbon-based matrix, combining the carbon emissions due to the seismic damage with the overall operation and embodied impact of the building system; and (iii) a resilience-based matrix, assessing seismic and climate resilience by considering the expected functionality drop (impact of damage and energy efficiency for earthquakes and heatwaves, respectively) and recovery time to the pre-event equilibrium state. For each matrix, specific integrated ranges of values and labeling can be determined based on existing classifications for seismic and climate performance. Lastly, an integrated loss matrix can be derived from the single matrix outcomes by applying weighting factors to the single losses.
The proposed approach takes into account that individual mitigation strategies might not prove effective across multiple hazards. Instead, integrated solutions have the potential to generate compounded and greater savings over the building lifespan. To achieve a specific resilience index based on stakeholder requirements or desired recovery states, a multi-objective optimization process may be employed to identify the solution that minimizes economic/environmental/resilience loss. It is worth noting that research on multi-criteria procedures has been developed, particularly in façade engineering. However, existing studies have primarily focused on construction and operational energy efficiency, such as the choice of technology, design of control strategies, and occupant comfort[38,39], with little or no consideration for resilience quantification.
Description of the case study
This research involves the validation of the proposed methodology on a seven-story building. The structural configuration and overall dimensions of the building were extrapolated from a prior investigation conducted by Bianchi et al.[40]. However, the current building is located in Messina and has different façade properties. As a result, the seismic design and loss modeling (and further elaborations for resilience quantification) were implemented for the new case-study scenario, and detailed outcomes are provided in the following section.
The building features a rectangular floor plan measuring 32 meters in length and 18 meters in width, with a total height of 26.6 meters and an inter-story height of 3.8 meters [Figure 3]. The building is used for commercial purposes, and the roof is not accessible. The seismic mass of this building is 729 tons per floor, while the roof has a mass of 564 tons. The main load-bearing structure comprises reinforced concrete (RC) frames (external beams: 0.4 m × 0.7 m; internal beams: 0.3 m × 0.6 m; columns: 0.7 m × 0.7 m), RC walls
The study aims to select the optimal façade system for the building structure by considering different alternatives. Facades (or building envelopes) indeed represent a significant portion of the building investment cost, ranging from 15 to 30% of the total cost[41]. They also have a crucial role in mitigating the effects of climate change and can contribute up to 31% of the total embodied carbon of a building[42]. Moreover, they have a notable influence on the operational energy efficiency of buildings and can highly affect post-earthquake losses[43]. To this end, the study considers alternative façade systems with details shown in Figure 3, including (a) 300 mm brick cavity walls (CWs); (b) modular prefabricated steel-stud panel system with external 100 mm thick concrete claddings connected to the main structure using bearing and tie-back connections; and (c) double-skin façade with an external glazing and an internal infilled wall. All façade systems have double-pane windows (U = 2.4 W/m2K) with a 0.3 Window-to-Wall Ratio, and solar blinds are applied to the glazed surfaces. To improve seismic performance and/or environmental sustainability, three additional façade systems are considered: (a) ventilated facades with internal rocking masonry panels (i.e., consisting of vertical sub-panels and internal gaps) and external timber claddings; (b) modular wood-stud panels with an external sandwich panel (SP) connected by dissipative U-shaped connections[44]; and (c) double-skin façade with an internal rocking wall system and integrated movable shading systems. These facades comprise double-pane windows with low-e coating (U = 1.2 W/m2K).
The structural/seismic design of the building is based on the building layout, geometry, and pertinent vertical loads, and it considers the seismic hazard in Messina (Italy). Messina is classified as a high-seismicity zone (Zone 1) according to the Italian risk map. In this region, earthquakes with ground acceleration exceeding 0.25 g may occur. From a climate perspective, the city experiences a warm temperate Mediterranean climate characterized by dry/warm summers and moderate/wet winters (Köppen-Geiger Climate Classification: “Csa”: Mediterranean Climate). The city has experienced several heatwaves in the past, with one notable extreme scenario occurring in 2021. This particular heatwave (Figure 4, with a maximum recorded temperature of 38.2 °C) lasted for 60 days and is being considered in this study. Weather data for this specific heatwave can be obtained from the Copernicus online services.
Figure 4. Heatwave scenario for the city of Messina, with highlighted (in red) the maximum and minimum dry-bulb temperatures.
The seismic design of the lateral load resisting systems (frames/walls) was implemented at the Ultimate Limit State (ULS) level (C soil category, T1 topographic zone, peak ground acceleration ag of
Finally, design criteria are established to ensure the internal comfort of occupants. The set point temperature is maintained at 24 °C for cooling while set to 19 °C for heating. As for the internal heat gain, the equipment load is 5 W/m2, the artificial lighting load is 10 W/m2, and the occupancy density is 0.01 persons per square meter. Additionally, the targeted rate of outdoor air infiltration stands at 0.0003 m3/s per square meter of floor area. The HVAC is considered as an Ideal Load System, thus not accounting for any inefficiencies of the system.
RESULTS
Seismic analysis
The case-study building was numerically modeled using Ruaumoko2D[49]. A lumped plasticity approach was used to represent the beam-column and column/wall-foundation connections where the inelastic behavior is expected. The joint panel zones were modeled as rigid elements, while the structural elements were represented through one-dimensional elastic elements incorporating plastic hinges at the end sections. This numerical approach has been extensively validated in the literature[50,51]. Following the code-compliant procedure in the Italian code[45], the structural connections were designed to develop a strong column-weak beam mechanism within the frame system according to capacity design principles. The plastic hinges of the beam/column/wall end-section(s) were described by moment-curvature functions and hysteresis rules that account for stiffness degradation (Takeda). Fixed-base connections were implemented without considering the influence of soil-structure interaction. Apart from the primary frame model, a secondary numerical representation is constructed for the case studies featuring external masonry facades in order to consider the influence of these heavy walls on the overall seismic response. Indeed, these facade systems have the potential to augment the stiffness and strength of the building, potentially resulting in unanticipated collapse mechanisms at both component and structure levels[52]. In these numerical models, the masonry walls are simulated by means of diagonal axial springs calibrated based on the formulations introduced by Bertoldi et al. and incorporating the hysteresis rule developed by Crisafulli[53,54].
Non-linear push-over analyses were carried out to identify capacity curves of both bare frames and infilled structures, and these were transformed into acceleration-displacement curves [Figure 5]. It is important to note that non-linear static/push-over analyses are considered a favorable trade-off between precision and simplicity, especially when compared to simplified analytical methodologies or time-intensive non-linear dynamic analyses. While non-linear static procedures might potentially result in slightly conservative predictions of seismic costs[55], this approach still serves as a valuable tool for practical engineering applications. It aids in decision-making during the initial stages of building design, where the objective is to determine the optimal alternative among various design options.
Figure 5. Push-over curves and performance points (PP) of the bare structure vs. infilled structure: frame (A) and wall (B) directions.
In accordance with the local seismic regulation[45], four earthquake intensity levels are taken into account (denoted as Immediate Operational - SLO, Damage Control - SLD, Life Safety - SLV, and Collapse Prevention - SLC). The capacity spectrum methodology[56] was employed to determine the Performance Points (PPs) of the buildings at each earthquake intensity. The PPs facilitate the computation of floor accelerations and inter-story drift levels, which serve as the input data for the loss estimation. The findings underscore that the inclusion of masonry walls results in heightened stiffness and strength, particularly noticeable in the direction of the frame that is characterized by a greater number of infills. Consequently, a rise in acceleration demand and a reduction in displacements are observed at the PPs when compared to the equivalent configurations without infills.
To conduct the loss modeling, the building replacement costs were estimated considering the labor expenses and encompassing quantities of concrete and steel, formwork costs, safety measures, foundation costs, and necessary surveys. Moreover, fragility curves, along with corresponding consequence functions (repair cost/time), were established for all building components. The fragility data of the concrete structural members and the internal building components (including lightweight partitions, ceilings, equipment, and contents) were defined based on the FEMA P-58[36]. For the façade systems, the fragility curves were defined as follows. For the masonry infill walls, fragility data are provided by Cardone and Perrone[57], while the damage states of the masonry rocking system were determined based on the outcomes from experimental testing[58,59]. The experimental data establish the median drift/displacement values of the various damage mechanisms, with a dispersion/beta value of 0.5 adopted to consider uncertainties in the fragility definition. Figure 6A shows a comparison of the fragility curves for the monolithic vs. rocking masonry infill wall (monolithic wall: DS1 = light diagonal cracking, DS2 = extensive diagonal cracking, DS3 = corner crushing and sliding of mortar joints, DS4 = global in-plane collapse; low-damage wall: DS1 = minor mortar cracking, DS2 = light mortar cracking, minor toe-crushing). For the traditional (with tie-back) vs. low-damage (with U-shaped dissipater) precast cladding systems, the fragility data were defined by referring to the data collection provided by Bianchi and Pampanin[5]. In order to assess the environmental impact associated with the potential earthquake damage, the global warming potential and primary energy use of the repair actions of all the building components were assumed, referring to the data provided by Simonen et al. (e.g., Figure 6B shows the contribution to the carbon emissions for the RC members of the building, where DS1, DS2, and DS3 indicate slight, moderate, and major damage, respectively)[60].
Figure 6. (A) Fragility curves of the monolithic vs. low-damage masonry infill (with no openings). (B) Embodied carbon associated with different damage states of RC components (data elaborated from Simonen et al.[60]).
Table 1 and Figure 7 present the results of the investigation into the losses associated with the different façade systems. Using probabilistic-based simulations, the repair costs (EAL - expressed in relation to the replacement cost), carbon emissions, and embodied energy associated with repairing each system were calculated. These annualized losses enable a comprehensive understanding of the long-term implications and facilitate informed decision-making regarding safer and sustainable facade solutions. The analysis highlights the effectiveness of low-damage solutions for building envelopes, such as those adopted in the SP system (UFP connections), as well as in the ventilated wall (VW) and double skin rocking (DSR) configurations (low-damage rocking masonry wall). Compared to conventional systems [CWs, concrete panes (CPs), double skin monolithic (DSM)], these facade solutions resulted in reductions of more than 50% for both financial and environmental losses. This finding emphasizes the importance of adopting cost-affordable sustainable facade solutions to mitigate the overall impact of seismic repairs. Notably, replacing tie-back connections of CPs with dissipative connections led to a significant reduction in the damage-related carbon emissions, approximately 70%. The UFP connections are indeed capable of greatly reducing the seismic demand on the cladding panel, thereby minimizing damage. Moreover, these connections are designed to fail due to fatigue rather than achieving a specific inter-story drift level (typical range of
Figure 7. (A) Seismic-related Expected Annual Loss (EAL), defined as a percentage of the Replacement Cost. (B) Expected Carbon Loss (ECL).
Annualized seismic-related losses
Facade system | Seismic cost [€] | Carbon emission [kg/CO2eq] |
Cavity wall - CW | 17,267.2 | 10,095.7 |
Ventilated wall - VW | 9,897.8 | 4,417.7 |
Concrete panel - CP | 17,458.8 | 15,731.3 |
Sandwich panel - SP | 10,041.8 | 4,414.3 |
Double skin monolithic - DSM | 17,187.4 | 10,095.1 |
Double skin rocking - DSR | 9,652.4 | 4,523.6 |
To assess the impact of earthquakes on building functionality, the loss results were elaborated to compute the expected downtime associated with repairs. This included repair duration, post-earthquake inspections, mobilization of engineering and contracting services, and financing and permitting. Following the procedure outlined in Almufti et al., the sequence of repairing actions and the impeding factors were defined[23]. The recovery time, estimated from the expected downtime, was then combined with the damage-related loss of functionality to define resilience curves and expected loss of resilience for all case study configurations. Figure 8 and Table 2 present the differences in RL among the different case studies. The highest loss of resilience was associated with configurations that included both monolithic masonry panels, which required longer recovery time (537 days), and the CP system, which suffered a higher loss of functionality at the specific seismic intensity level (SLV). However, the final design decision is based on the total seismic resilience value, computed according to Bruneau et al.[22]. This index considers the complete loss of resilience expected for the component, encompassing the period from the occurrence of the earthquake (t = 0) until the conclusion of the recovery phase. The objective of the decision-making process is to maximize this resilience value, ensuring the chosen solution can effectively withstand seismic events and recover efficiently. As shown in Table 2, among the available facade options, the application of the ventilated facade stands out as the most favorable choice for this specific application. The ventilated facade solution is distinguished by a reduced functionality drop and recovery time, showcasing its ability to absorb seismic forces/displacements and swiftly return to its pre-event state with minimal disruption.
Resilience indices for the earthquake scenario
Facade system | Resilience index | Resilience loss [%] |
Cavity wall - CW | 0.66 | 34 |
Ventilated wall - VW | 0.76 | 24 |
Concrete panel - CP | 0.58 | 42 |
Sandwich panel - SP | 0.73 | 27 |
Double skin monolithic - DSM | 0.69 | 31 |
Double skin rocking - DSR | 0.75 | 25 |
Finally, it is highlighted that this study focused on comparing different façade systems covering the same earthquake-resistant structural system. The latter contributed to 70% of the overall seismic damage and RL for the case studies with low-damage façade configurations. Therefore, applying advanced structural systems to the main structure could result in even higher reductions in RL. For instance, low-damage (rocking-dissipative) structural members could be used to further enhance the seismic resilience of buildings and minimize damage[40].
Energy and environmental analysis
A full life-cycle assessment was conducted to evaluate the sustainability level of the façade design strategies. This assessment aimed to measure the overall environmental impacts (operational and embodied energy) of the building with the alternative facade systems, accounting for all stages of their life cycle.
To determine the operational energy consumption and cost of the building, dynamic energy simulations were conducted in EnergyPlus software for both the typical meteorological year and the heatwave scenario. The thermal properties of buildings were precisely represented using a combination of geometry, material properties, and system specifications within the Grasshopper environment. This included modeling the walls (with a Window-to-Wall ratio of 0.4), roofs, floors, windows (with double-pane glass), and doors and assigning values for thermal conductivity, specific heat, density, and emissivity to each building element. The thermal zones of the building were divided based on occupancy and thermal characteristics, which helped capture the dynamic interactions between different building elements and the HVAC system. As discussed in the case study description, design requirements were set to ensure the internal comfort of occupants. The model also considered internal heat gain from equipment and artificial lighting, as well as the density of occupation and rate of outdoor air infiltration. To model the HVAC system, an Ideal Load System was assumed. Although this did not account for system inefficiencies, it allowed for an accurate simulation of the operational energy consumption of a building. The overall modeling approach has been validated in a previous study that included uncertainty analysis[37]. This validation was conducted to assess the overall reliability of the energy simulation by evaluating the impact of uncertainties in input parameters on the simulation outcomes. Furthermore, for the specific location in Messina, a primary energy factor of 2.5 was considered, representing the ratio of primary energy to final consumption and incorporating losses that occur during energy generation, transmission, and distribution.
The embodied energy of the buildings was assessed from cradle to grave, including all stages from production to disposal (production, transportation, construction, use, maintenance, and end-of-life phases). The embodied impact was quantified by means of the Bombyx plugin[61] within the same Grasshopper environment, which includes a material and component database and enables users to select different materials and building systems for assessment. The analysis accounted for all building components, including the structure, external walls, internal systems, services, and the transport of people and materials.
Table 3 and Figure 9 summarize the results of the energy and environmental analyses. To assess the energy cost, the energy consumption for lighting, equipment, heating, and cooling was converted into Euros per square meter using the electricity and gas prices, along with taxes and levies provided by Eurostat for the Italian territory.
Figure 9. (A) Weather-related Expected Annual Loss (EAL), defined as a percentage of the Replacement Cost. (B) Expected Carbon Loss (ECL).
Annualized weather-related losses
Facade system | Energy cost [€] | Carbon emission [kg/CO2eq] |
Cavity wall - CW | 105,127.3 | 60,511.5 |
Ventilated wall - VW | 99,126.7 | 59,871.2 |
Concrete panel - CP | 104,716.5 | 59,290.6 |
Sandwich panel - SP | 99,135.8 | 59,464.2 |
Double skin monolithic - DSM | 104,697.8 | 60,217.6 |
Double skin rocking - DSR | 99,134.2 | 60,819.7 |
The comparison of the various facade solutions (designed for a new building) shows that the energy cost is similar for all options, with a slight reduction observed for the configurations with lower transmittance (VW, SP, DSR). Regarding the carbon emissions, the differences between the systems are minimal, but precast CPs exhibit lower emissions compared to other facade types involving masonry walls and glazed systems. This can be attributed to the manufacturing processes involved in the production of such facade systems.
Finally, the results can be elaborated to determine resilience curves in terms of loss of energy efficiency during the heatwave scenario. The daily loss of functionality and (cooling) energy performance during the heatwave can be assessed by examining energy consumption data. The resilience curves [Figure 10] are now characterized by fluctuations due to yearly temperature variations and the capacity of the heating/cooling system (in winter/summer) to maintain their setpoint temperatures.
The maximum cooling capacity of each thermal zone was determined by calculating the cooling demand during the design day for Messina. Therefore, the energy performance during the heatwave scenario is already influenced by reduced functionality compared to a 100% functionality level. When comparing the resilience indices for all alternative facades [Table 4], computed as the area underneath the curve in the
Resilience indices for the heatwave scenario
Facade system | Resilience index | Resilience loss [%] |
Cavity wall - CW | 0.82 | 18 |
Ventilated wall - VW | 0.83 | 17 |
Concrete panel - CP | 0.83 | 17 |
Sandwich panel - SP | 0.84 | 16 |
Double skin monolithic - DSM | 0.84 | 16 |
Double skin rocking - DSR | 0.85 | 15 |
Multi-criteria analysis
To compare the performance of the different façade systems, the results from seismic and energy/environmental analyses can be combined by using overall loss maps. These maps integrate key decision variables that are crucial when selecting new building systems or retrofitting/rehabilitating existing ones, such as economic, carbon, and resilience losses. Figure 11A-C presents the results for all the case studies, along with their classifications for seismic and energy variables. The EAL categories are established in accordance with established national codes, specifically the DM 65[62], commonly referred to as the "Sisma Bonus" guidelines for seismic classifications (ranging from A+ to G, where A+ indicates superior seismic performance), and the DM 26[63] for energy classifications (ranging from A4-1 to G, with A4 indicating higher energy efficiency). The embodied carbon targets are defined from existing rating systems developed for office buildings, such as the LETI and RIBA targets[64] (from A++ to G, where A++ identifies reduced upfront embodied carbon, less than 100 kg CO2eq/m2, and life-cycle embodied carbon, less than
Figure 11. Comparison of design alternatives in terms of integrated seismic and energy/environmental (A) Expected Annual Losses - EAL, (B) Carbon Emissions - CO2, (C) Resilience Loss - RL, and (D) the overall weighted loss.
The results of the integrated assessment further highlight that: (1) applying low-damage solutions for the building envelope can significantly reduce the economic losses (by more than 50%), leading to an integrated Class A+, even without using advanced solutions for the structural system; (2) the life-cycle embodied carbon increases (by less than 20%) with high-performance systems, enabling the achievement of an integrated Class A, which represents the 2030 target for office buildings[64]; and (3) the integrated reduction of RL is highly affected by seismic resilience for this specific application: the solutions in Class A show a higher seismic RL (twice the energy RL) associated with the CP system, affected by a higher functionality drop at the earthquake intensity level under consideration (SLV). It is worth noting that this study only investigated the effect of façade systems, and higher loss reductions could have been achieved by considering earthquake-proof solutions for the overall building system (structure + envelope).
Finally, the study employs a multi-criteria approach to compare results in the same graph [Figure 11D]. A pairwise comparison (based on the Analytic Hierarchy Process[65]) is used to compare the seismic/energy decision variables and determine the priority value (criteria weight, w) to assign to each parameter. The weighted loss (= EAL × wEAL + CO2 × wCO2 + RL × wRL) is thus used to compare the integrated seismic and energy/environmental results. In this specific study, more importance was assigned to the CO2 and EAL values, while equal importance was given to the RL. By keeping the seismic and energy results separate, the map (where both façade outcomes and classification values were defined as weighted values) shows the domain of the overall loss associated with the six different façade alternatives. The results indicate that the seismic performance has a more significant influence on the overall loss than the energetic behavior.
The proposed multi-criteria approach offers a practical method that can be integrated into common practice and existing decision-making processes to facilitate the selection of design strategies. By adopting this approach, designers can identify potential multi-hazard resistant design solutions from the very early stages of the design process. This empowers them to make informed choices based on crucial decision variables, including expected financial, environmental, and resilience losses in the building life-cycle. Through this approach, designers can work towards minimizing these losses, thus identifying optimal design scenarios based on the specific multi-hazard local scenario. Furthermore, the flexibility of the approach allows stakeholders to assign specific targeted values to the cumulative or single losses based on their interests. This customization enables the development of “tailored” ad-hoc solutions for building projects, aligning them with the unique needs and objectives of each stakeholder.
It is finally highlighted that while the proposed method offers a simplified yet effective multi-criteria approach to quantify the efficacy of integrated multi-hazard resistant strategies, a more comprehensive and robust framework should be developed to consider all uncertainties and hazard scenarios that may affect the final outcome. Following a similar approach as currently adopted in seismic engineering, this procedure would consider both aleatoric (weather-related) and epistemic (material properties, design variations) uncertainties to define probable maximum losses, such as the 90th percentile values. The work by
CONCLUSIONS
The vulnerability of our built environment to natural disasters, such as earthquakes and climate change-induced weather events, has resulted in significant losses. Therefore, building a resilient society is increasingly recognized as a priority for risk reduction and management policies. However, current design approaches focus on single hazards and economic and environmental indicators, with limited or no consideration for resilience. This paper proposes a practical multi-criteria approach based on loss-based matrices to aid early-stage design decisions. The primary objective is to enhance the safety, resilience, and sustainability of buildings against earthquakes and heatwaves. The research underscores the crucial role of integrating resilience considerations with economic and environmental losses, emphasizing the importance of using the proposed approach to improve investment decisions and support early-stage decision-making processes for both new buildings and retrofit interventions. The approach is applied to a commercial building with various seismic-resistant and energy-efficient facades. The study carries out seismic analyses, life-cycle analysis, and dynamic energy simulations to estimate overall annual losses and resilience associated with design-level earthquake and extreme heatwave scenarios. Results show that low-damage facades (rocking masonry walls and dissipative connections) can reduce financial loss by over 50%, and seismic resilience has a significant influence on the final decision. More advanced “earthquake-proof” solutions for the structural system and low-carbon strategies could reduce the overall losses further.
While the proposed method provides a simplified multi-criteria approach to quantify the efficacy of multi-hazard resistant strategies in reducing the expected losses, a more comprehensive uncertainty-based integrated framework is needed. In this study, a probabilistic approach was employed for the seismic analysis only, as it aligns with the current performance-based design method. However, an uncertainty-based approach should also be adopted for the energy/environmental analysis. Additionally, the effect of alternative hazard scenarios (the paper focused solely on the design-level earthquake and a recorded heatwave scenario) and their long-term impact on the service life of a building should be considered. Furthermore, while the study evaluated energy resilience based on the capacity of a building to maintain energy efficiency, it is essential to include the quantification of thermal resilience to consider discomfort hours and the impact of heatwaves on human health, as they can significantly affect the overall risk of the building.
Overall, the proposed approach provides valuable insights for informing decisions related to building design and disaster preparedness by quantifying the impact of extreme events on the functionality of buildings. However, the study highlights the need for further research to effectively evaluate the cumulative impact of earthquakes and heatwaves on buildings and infrastructure. An integrated mathematical approach that considers the probability of both events (different intensities and frequencies) occurring within a life-cycle framework is crucial for assessing the expected cumulative losses. Developing new risk assessment methodologies, also accounting for potential interdependence between earthquakes and heatwaves, is essential to promote more robust policies and regulations to enforce resilient building codes. By adopting such methodologies, policymakers, building designers, and engineers can make more informed decisions and prioritize interventions to develop more resilient buildings and infrastructure that can withstand multiple hazards, ultimately contributing to a more sustainable and safer built environment.
DECLARATIONS
Authors’ contributions
The author contributed solely to the article.
Availability of data and materials
Not applicable.
Financial support and sponsorship
This study has received funding from the European Union’s Horizon 2020 research and innovation program under the Marie Skłodowska-Curie grant agreement No. 101029605 (H2020-MSCA-IF-2020 - SAFE-FACE - Seismic SAFety and Energy efficiency: Integrated technologies and multi-criteria performance-based design for building FACadEs) for Simona Bianchi.
Conflicts of interest
The author declared that there are no conflicts of interest.
Ethical approval and consent to participate
Not applicable.
Consent for publication
Not applicable.
Copyright
© The Author(s) 2023.
REFERENCES
1. Kam WY, Pampanin S, Elwood K. Seismic performance of reinforced concrete buildings in the 22 February Christchurch (Lyttelton) earthquake. Bull NZ Soc Earthq Eng 2011;44:239-78.
2. Pampanin S. Reality-check and renewed challenges in earthquake engineering: implementing low-damage systems - from theory to practice. Bull NZ Soc Earthq Eng 2012;45:137-60.
3. O’reilly GJ, Perrone D, Fox M, Monteiro R, Filiatrault A. Seismic assessment and loss estimation of existing school buildings in Italy. Eng Struct 2018;168:142-62.
4. Sousa L, Monteiro R. Seismic retrofit options for non-structural building partition walls: Impact on loss estimation and cost-benefit analysis. Eng Struct 2018;161:8-27.
5. Bianchi S, Pampanin S. Fragility functions for architectural nonstructural components. J Struct Eng 2022;148:03122005.
6. National Centers for Environmental Information. Monthly global climate report for annual 2021. Available from: https://www.ncei.noaa.gov/access/monitoring/monthly-report/global/202113/supplemental/page-1 [Last accessed on 15 August 2023].
7. Meehl GA, Tebaldi C. More intense, more frequent, and longer lasting heat waves in the 21st century. Science 2004;305:994-7.
8. Crawley DB. Estimating the impacts of climate change and urbanization on building performance. J Build Perform Simul 2008;1:91-115.
9. Sun K, Zhang W, Zeng Z, Levinson R, Wei M, Hong T. Passive cooling designs to improve heat resilience of homes in underserved and vulnerable communities. Energy Build 2021;252:111383.
10. EEA. Economic losses from climate-related extremes in Europe. Copenhagen, Denmark: European Environmental Agency, 2019.
11. Ritchie H, Rosado P, Roser M. Natural disasters. Available from: https://ourworldindata.org/natural-disasters [Last accessed on 15 August 2023].
12. Pohoryles D, Bournas D, Da Porto F, Caprino A, Santarsiero G, Triantafillou T. Integrated seismic and energy retrofitting of existing buildings: A state-of-the-art review. J Build Eng 2022;61:105274.
13. Menna C, Felicioni L, Negro P, et al. Review of methods for the combined assessment of seismic resilience and energy efficiency towards sustainable retrofitting of existing European buildings. Sustain Cities Soc 2022;77:103556.
14. Menna C, Del Vecchio C, Di Ludovico M, Mauro GM, Ascione F, Prota A. Conceptual design of integrated seismic and energy retrofit interventions. J Build Eng 2021;38:102190.
15. Bournas DA. Concurrent seismic and energy retrofitting of RC and masonry building envelopes using inorganic textile-based composites combined with insulation materials: A new concept. Composites Part B Eng 2018;148:166-79.
16. Zanni J, Cademartori S, Marini A, et al. Integrated deep renovation of existing buildings with prefabricated shell exoskeleton. Sustainability 2021;13:11287.
17. Baek E, Pohoryles D, Kallioras S, Bournas D, Choi H, Kim T. Innovative seismic and energy retrofitting of wall envelopes using prefabricated textile-reinforced concrete panels with an embedded capillary tube system. Eng Struct 2022;265:114453.
18. Caruso M, Pinho R, Bianchi F, Cavalieri F, Lemmo MT. Integrated economic and environmental building classification and optimal seismic vulnerability/energy efficiency retrofitting. Bull Earthq Eng 2021;19:3627-70.
19. Calvi GM, Sousa L, Ruggeri C. Energy efficiency and seismic resilience: a common approach. In: Multi-hazard approaches to civil infrastructure engineering. Berlin: Springer; 2016. pp. 165-208.
20. Pohoryles D, Maduta C, Bournas D, Kouris L. Energy performance of existing residential buildings in Europe: A novel approach combining energy with seismic retrofitting. Energy Build 2020;223:110024.
21. Marini A, Passoni C, Belleri A, et al. Combining seismic retrofit with energy refurbishment for the sustainable renovation of RC buildings: a proof of concept. Eur J Environ Civ Eng 2022;26:2475-95.
22. Bruneau M, Chang SE, Eguchi RT, et al. A framework to quantitatively assess and enhance the seismic resilience of communities. Earthq Spectra 2003;19:733-52.
23. Almufti I, Willford M. REDi Rating system resilience-based earthquake design initiative for the next generation of buildings. Arup, San Francisco, United States, 2013.
24. FEMA. Recommended options for improving the built environment for post-earthquake reoccupancy and functional recovery time, FEMA P-2090/ NIST SP-1254. Washington, United States, 2021.
25. Marasco S, Kammouh O, Cimellaro GP. Disaster resilience quantification of communities: a risk-based approach. Int J Disaster Risk Reduct 2022;70:102778.
26. Samadian D, Ghafory-ashtiany M, Naderpour H, Eghbali M. Seismic resilience evaluation based on vulnerability curves for existing and retrofitted typical RC school buildings. Soil Dyn Earthq Eng 2019;127:105844.
27. Hu J, Wen W, Zhai C, Pei S, Ji D. Seismic resilience assessment of buildings considering the effects of mainshock and multiple aftershocks. J Build Eng 2023;68:106110.
28. Rodrigues L, Gillott M. Building for future climate resilience. In: Hakansson A, Höjer M, Howlett R, Jain L, eds. Sustainability in energy and buildings. smart innovation, systems and technologies. Berlin: Springer; 2013. pp. 453-62.
29. Zeng Z, Zhang W, Sun K, Wei M, Hong T. Investigation of pre-cooling as a recommended measure to improve residential buildings’ thermal resilience during heat waves. Build Environ 2022;210:108694.
30. O’Donovan A, Murphy MD, O’sullivan PD. Passive control strategies for cooling a non-residential nearly zero energy office: simulated comfort resilience now and in the future. Energy Build 2021;231:110607.
31. Sharifi A, Pathak M, Joshi C, He B. A systematic review of the health co-benefits of urban climate change adaptation. Sustain Cities Soc 2021;74:103190.
32. Homaei S, Hamdy M. Thermal resilient buildings: how to be quantified? A novel benchmarking framework and labelling metric. Build Environ 2021;201:108022.
33. Pongiglione M, Calderini C. Sustainable structural design: comprehensive literature review. J Struct Eng 2016;142:04016139.
34. Moehle J, Deierlein G. A framework methodology for performance-based earthquake engineering. In Proceedings of the 13th World Conference on Earthquake Engineering; 1-6 August 2003; Vancouver, United States.
35. Porter KA. An overview of PEER’s performance-based earthquake engineering methodology. In Proceedings of the 9th International Conference on Applications of Statistics and Probability in Civil Engineering; 6-9 July 2003; San Francisco, United States.
36. FEMA. Seismic performance assessment of buildings, volume 1 - methodology. technical report FEMA-P-58-1. Washington, USA: Federal Emergency Management Agency; 2018.
37. Bianchi S, Ciurlanti J, Overend M, Pampanin S. A probabilistic-based framework for the integrated assessment of seismic and energy economic losses of buildings. Eng Struct 2022;269:114852.
38. Jin Q, Overend M. A prototype whole-life value optimization tool for façade design. J Build Perform Simul 2014;7:217-32.
39. Montali J, Sauchelli M, Jin Q, Overend M. Knowledge-rich optimisation of prefabricated façades to support conceptual design. Autom Costr 2019;97:192-204.
40. Bianchi S, Ciurlanti J, Pampanin S. Comparison of traditional vs low-damage structural and non-structural building systems through a cost/performance-based evaluation. Earthq Spectra 2021;37:366-85.
41. Klein T. Integral facade construction - towards a new product architecture for curtain walls. Delft: Delft University of Technology; 2013.
42. Arup. Net-zero buildings Where do we stand? Technical report, 2021. Available from: https://globalabc.org/sites/default/files/2021-07/Net_zero_buildings_where_do_we_stand.pdf [Last accessed on 16 August 2023].
43. Baird A, Palermo A, Pampanin S. Façade damage assessment of concrete buildings in the 2011 Christchurch earthquake. Struct Concrete 2012;13:3-13.
44. Kelly JM, Skinner RI, Heine AJ. Mechanisms of energy absorption in special devices for use in earthquake resistant structures. Bull NZ Soc Earthq Eng 1972;5:63-88.
45. NTC. Aggiornamento delle Norme Tecniche per le Costruzioni, Supplemento ordinario n°8 alle G.U. n° 42 del 20/02/2018. Rome, Italy, 2018. Available from: https://www.gazzettaufficiale.it/eli/gu/2018/02/20/42/so/8/sg/pdf [Last accessed on 16 August 2023].
46. Priestley NMJ, Calvi GM, Kowalsky MJ. Direct displacement-based seismic design of structures. Pavia: IUSS Press; 2007.
47. Pampanin S, Marriott D, Palermo A, New Zealand concrete society. PRESSS design handbook. Auckland: New Zealand Concrete Society; 2010.
48. Ciurlanti J, Bianchi S, Pampanin S. Raising the bar in seismic design: cost-benefit analysis of alternative design methodologies and earthquake-resistant technologies. Bull Earthq Eng 2023;21:2723-57.
49. Carr Athol J. Ruaumoko program for inelastic dynamic analysis - user manual. Christchurch: University of Canterbury; 2003.
50. Zendaoui A, Kadid A, Yahiaoui D. Comparison of different numerical models of RC elements for predicting the seismic performance of structures. Int J Concr Struct Mater 2016;10:461-78.
51. Carvalho G, Bento R, Bhatt C. Nonlinear static and dynamic analyses of reinforced concrete buildings - comparison of different modelling approaches. Earthq Struct 2013;4:451-70.
52. Magenes G, Pampanin S. Seismic response of gravity-load design frames with masonry infills. In Proceedings of the 13th World Conference on Earthquake Engineering; 1-6 August 2003; Vancouver, United States.
53. Bertoldi SH, Decanini LD, Gavarini C. Telai tamponati soggetti ad azione sismica, un modello semplificato: confronto sperimentale e numeric. In Proceedings of the 6th Convegno Nazionale ANIDIS; 13-15 October 1993; Perugia, Italy.
54. Crisafulli FJ. Seismic behaviour of reinforced concrete structures with masonry infills. PhD Thesis, Christchurch: University of Canterbury, 1997.
55. Bianchi S, Ciurlanti J, Pampanin S. A SLaMA-based analytical procedure for the cost/performance-based evaluation of buildings. In Proceedings of the 7th International Conference on Computational Methods in Structural Dynamics and Earthquake Engineering; 24-26 June 2019; Crete, Greece.
56. Freeman SA. Review of the development of the capacity spectrum method. ISET J Earthq Technol 2004;41:1-13. Available from: https://engineering.purdue.edu/~ce573/Documents/SAF_Review%20of%20Capacity%20Spectrum%20Method.pdf [Last accessed on 16 August 2023]
57. Cardone D, Perrone G. Developing fragility curves and loss functions for masonry infill walls. Earthq Struct 2015;9:257-79.
58. Tasligedik AS, Pampanin S. Rocking cantilever clay brick infill wall panels: a novel low damage infill wall system. J Earthq Eng 2017;21:1023-49.
59. Bianchi S, Ciurlanti J, Perrone D, et al. Shake-table tests of innovative drift sensitive nonstructural elements in a low-damage structural system. Earthquake Engng Struct Dyn 2021;50:2398-420.
60. Simonen K, Huang M, Aicher C, Morris P. Embodied carbon as a proxy for the environmental impact of earthquake damage repair. Energy Build 2018;164:131-9.
61. Basic S, Hollberg A, Galimshina A, Habert G. A design integrated parametric tool for real-time life cycle assessment - bombyx project. IOP Conf Ser Earth Environ Sci 2019;323:012112.
62. Ministero delle Infrastrutture. Linee guida per la classificazione del rischio sismico delle costruzioni, Allegato A. Decreto Ministeriale 65 of 07/03/2017, Rome, Italy, 2017. Available from: https://www.logical.it/pdf/sismabonus/DM_65_del_07-03-2017_All_A.pdf [Last accessed on 16 August 2023].
63. Ministero dello Sviluppo Economico. Linee guida nazionali per la certificazione energetica degli edifici, Allegato 1. Decreto interministeriale 26 Giugno 2015, Rome, Italy, 2015. Available from: https://www.mimit.gov.it/images/stories/normativa/DM_Linee_guida_APE_allegato1.pdf [Last accessed on 16 August 2023].
64. LETI. Embodied carbon target alignment. London Energy Transformation Initiative, 2020. Available from: https://www.leti.uk/_files/ugd/252d09_25fc266f7fe44a24b55cce95a92a3878.pdf [Last accessed on 16 August 2023].
65. Saaty TL. The analytic hierarchy process. New York: McGraw-Hill; 1980. p. 287.
Cite This Article
How to Cite
Download Citation
Export Citation File:
Type of Import
Tips on Downloading Citation
Citation Manager File Format
Type of Import
Direct Import: When the Direct Import option is selected (the default state), a dialogue box will give you the option to Save or Open the downloaded citation data. Choosing Open will either launch your citation manager or give you a choice of applications with which to use the metadata. The Save option saves the file locally for later use.
Indirect Import: When the Indirect Import option is selected, the metadata is displayed and may be copied and pasted as needed.
About This Article
Copyright
Data & Comments
Data
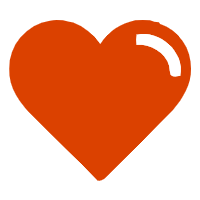
Comments
Comments must be written in English. Spam, offensive content, impersonation, and private information will not be permitted. If any comment is reported and identified as inappropriate content by OAE staff, the comment will be removed without notice. If you have any queries or need any help, please contact us at [email protected].