Selective syngas conversion to olefins over bifunctional Zn2Al3O4/PLS-3 catalyst
Abstract
Designing zeolites with novel topologies and tunable acidity to construct metal oxide-zeolite bifunctional catalytic systems for targeted transformation of syngas into high-value olefins has aroused wide interest. PLS-3 aluminosilicates with the FER topology and unique nanorod crystal morphology, derived from layered precursors with a wide Si/Al ratio range of 50-300, were applied to combine with Zn2Al3O4 oxide, constructing bifunctional catalysts for selective syngas conversion reaction. The lower Al content in PLS-3 zeolite led to decreased acid amount and the preferred distribution of framework Al atoms in specific tetrahedral locations (T2 and T4), which promoted the formation of ethylene. High-silica PLS-3 (Si/Al = 250) combined with Zn2Al3O4 oxide showed high selectivity for C2-5= (78.5%), especially for ethylene (45%), and high ratios of ethylene to propylene (E/P, 7.0) and olefin to paraffin (O/P, 21.2). Furthermore, the in-situ infrared spectra evidenced that the syngas conversion over Zn2Al3O4/PLS-3 catalyst possibly followed the carbonylation route.
Keywords
INTRODUCTION
Syngas conversion is an effective process that converts non-petroleum carbon-based resources, such as coal, natural gas, and biomass, into high-value hydrocarbon chemicals[1-3]. The precise regulation of product distribution is a great challenge in traditional Fischer-Tropsch synthesis (FTS) process because the product distribution follows the Anderson-Schulz-Flory (ASF) model. Light olefins are important basic chemical raw materials, and thus the selective conversion of syngas to light olefins has become a research hotspot[4,5]. The Fe, Co, Ni, and Ru metals as main catalysts of FTS process have been extensively studied, but their light hydrocarbon selectivity did not exceed 58%. The supported iron nanoparticles and cobalt carbide nanoprisms were prepared by the regulation of metal morphology, and the high selectivity of light olefins up to 60% could be achieved, breaking the ASF distribution[6,7]. Furthermore, the bifunctional catalyst was also developed for the syngas conversion reaction by combining the FTS metal with zeolites, where zeolites with distinctive shape-selectivity and tunable acidity can shear the long-chain hydrocarbons to realize the high selectivity of light olefin[8-10]. However, the selectivity of light olefins still is low on traditional FTS process.
In 2016, the syngas direct conversion into light olefins was achieved by coupling the methanol synthesis and methanol to olefins (MTO) process using the bifunctional catalysts of metal oxides (ZnCrOx, ZnZrOx, and ZnAlOx) combined with the SAPO-34 zeolite[11-13]. The composited catalyst system of metal oxide–zeolite greatly broke the ASF limitation of syngas conversion, and the light olefin selectivity reached as high as 80%, far exceeding that of the conventional FTS process. The activation of CO and facilitation of C–C coupling is critical for syngas conversion, and the CH2CO (ketene) and/or CH3O* (methoxy) species have been confirmed as intermediates by activating CO* and H2 (or H*) species over metal oxide[11-13]. For the ketene intermediate route, the protonation of ketene leads to the formation of CH3CO species, which act as methylating agents toward hydrocarbon pool in SAPO-34 zeolite[14], and then higher olefins or the corresponding carbenium ions obtained by the methylation of olefins with ketene might be cracked into light olefins. In addition, for methanol as an intermediate in the syngas conversion, the hydrocarbon pool mechanism of MTO has been widely accepted[15]. Recently, the methanol carbonylation route to acetic acid (AA) followed by the selective conversion of AA to ethylene was proposed over bifunctional catalyst for the methanol conversion in syngas[16].
The metal oxide components result in the difference of intermediates, such as ketene and methanol, while zeolite is responsible for the intermediate conversion to obtain the target product for the oxide-zeolite catalyzed syngas conversion[11-13]. The topology, pore structure and acidity of zeolite synergistically affect the product distribution in the metal oxide-zeolite bifunctional catalyst system. The small-pore zeolites with the CHA (SAPO-34 and SSZ-13) and AEI (SAPO-18 and AIPO-18) topologies both gave the main hydrocarbon products of light olefins[17-21]. Wang et al. found that different product distributions in a range of light olefins were obtained using 8-ring (R) small-pore zeolites with different cavities and topologies, such as SAPO-17
Jiao et al. also found that the Ge-substituted AlPO-18 zeolite with ZnCrOx gave a C2-4= selectivity of 83% in syngas conversion reaction, which is due to the weaker acidity of Ge(OH)Al group in comparison to that of Si(OH)Al[24]. Recently, we combined Al-IDM-1 (-ION) zeolite with ZnaAlbOx oxide to catalyze the syngas conversion reaction, showing a C3-4= selectivity of 88% in light olefins and a high O/P ratio of 14[25]. We demonstrated that the Al-IDM-1 zeolite exhibited weak acidity, thus enhancing the olefin-based cycle and promoting C3-4= formation. These studies indicated that the weakened acidity has been proven to favor the formation of light olefins. Thus, the usage of zeolites with suitable pore structure and acidity to catalyze the conversion of syngas by combining with metal oxide is crucial to achieve specific olefins.
The FER zeolite consists of 8R (3.5 × 4.8 Å) and 10R (4.2 × 5.4 Å) pore channels along the b- and c-axes, respectively, which proves to be active in the reactions of 1-butene isomerization and carbonylation of dimethyl ether (DME)[26,27]. Similar to MOR zeolite, FER zeolite has the potential for selective conversion of syngas to olefins by making full use of its 8R pores. Herein, the PLS-3 zeolites with different Si/Al ratios, derived from a lamellar precursor with a structure similar to PREFER, were synthesized to catalyze the syngas conversion reaction by combining with Zn2Al3O4 oxide. The effect of zeolite acidity and reaction conditions on the catalytic performances was investigated in detail in the syngas conversion. In addition, the syngas conversion mechanism over the Zn2Al3O4/PLS-3 catalyst was also studied via the in-situ infrared (IR) spectra. PLS-3 with suitable acidity was highly selective to the production of olefins, especially ethylene.
EXPERIMENTAL
Synthesis of PLS-3 zeolite
PLS-3 zeolites were synthesized using tetraethylammonium hydroxide solution (TEAOH) and H-kanemite as the structure-directing agent (SDA) and silica source, respectively, according to a previously reported method (Experimental details in Supplementary Materials)[28]. Typically, H-kanemite, NaOH, and
Catalytic reactions
The syngas conversion reaction was tested using a high-pressure fixed-bed stainless steel reactor (inner diameter, 9 mm). For the preparation of the composite catalyst, the Zn2Al3O4 and PLS-3-x zeolite powders were pressed, crushed, and sieved to 20-40 mesh granules separately. Then, the granules of the two components were physically mixed. Typically, 0.25 g composite catalyst with a metal oxide to zeolite mass ratio of 3:2 was used. The catalyst was pretreated using N2 (30 mL·min-1) at a temperature of 673 K for 2 h, cooled to 623 K, and then the N2 gas was switched to syngas (volume ratio, 1/1; 6,000 mL·g-1·h-1) with the reaction pressure adjusted to 1 MPa. Subsequently, the reaction began after achieving the target temperature, and an online gas chromatograph, equipped with PLOT/Q capillary column (30 m × 0.32 mm × 20 μm) connected to a flame ionization detector (FID) detector and MS-13X column connected to a thermal conductivity detector (TCD) detector, was used to analyze the products. Notably, 4% Ar was used as an inner standard in the syngas flow. The CO conversion, along with CO2 and hydrocarbon selectivity (CnHm), with CO2 and DME excluded from the hydrocarbon selectivity calculation, was calculated.
The CO conversion [X(CO)] is given by
Where
The CO2 selectivity [S(CO2)] is given by
Where
The hydrocarbon selectivity is given by
Where Ai is peak area of specific product i determined via the FID, and fi is its calibration factor.
RESULTS AND DISCUSSION
Textural properties of PLS-3 zeolites
The as-made PLS-3 zeolite, synthesized with layered silicate H-kanemite as a silica source, showed the X-ray diffraction (XRD) patterns similar to a typical FER-type layered zeolite PREFER
Figure 1. The (A) XRD patterns and (B) N2 sorption isotherms of PLS-3 zeolites with different Al contents. XRD: X-ray diffraction.
Figure 2. SEM images of (A) PLS-3-50, (B) PLS-3-100, (C) PLS-3-200 and (D) PLS-3-250. SEM: Scanning electron microscope.
Textural and physicochemical properties of PLS-3 zeolites with different Si/Al ratios
Sample | Si/Al[a] | SBET[b] (m2·g-1) | Smicro[c] (m2·g-1) | Sext[d] (m2·g-1) | Vtotal[e] (cm3·g-1) | Vmicro[f] (cm3·g-1) | Vmeso[g] (cm3·g-1) |
PLS-3-50 | 40 | 425 | 309 | 116 | 0.60 | 0.14 | 0.46 |
PLS-3-100 | 82 | 409 | 297 | 112 | 0.66 | 0.12 | 0.54 |
PLS-3-200 | 95 | 406 | 314 | 92 | 0.62 | 0.13 | 0.49 |
PLS-3-250 | 140 | 403 | 308 | 95 | 0.67 | 0.13 | 0.54 |
PLS-3-300 | 190 | 404 | 303 | 101 | 0.67 | 0.12 | 0.55 |
FER | - | 390 | 370 | 20 | 0.27 | 0.14 | 0.13 |
Acidity of PLS-3 zeolites
The acidity of PLS-3 zeolites with different Si/Al ratios was analyzed via the 27Al magic angle spinning nuclear magnetic resonance (MAS NMR), hydroxyl infrared spectroscopy (OH-IR) and pyridine-adsorption infrared spectroscopy (Py-IR), and NH3-temperature-programmed desorption (TPD) techniques. The 27Al MAS NMR spectra of PLS-3-x samples are shown in Figure 3A. The resonance peaks at 53 and 0 ppm are related to the tetrahedrally coordinated framework Al (AlF) and the extra-framework Al (EFAl), respectively[29-32]. Nearly all the Al atoms were tetrahedrally coordinated in the framework, showing a strong peak centered at 53 ppm, and the intensity was significantly weakened with the decrease of Al content. In addition, the peak corresponding to AlF gradually shifted to a higher field with chemical shift changing from 53.7 to 52.7 ppm with decreasing Al amount, indicating that the chemical environment of AlF changed with the variation of Al content. There are four different tetrahedral (T) sites for the framework Al in FER topology. The T1/T2 sites are distributed in the intersection of 10R and 8R pores [Supplementary Scheme 1] while T3 sites are located in the 10R pores. T4 sites situated in 6R pockets can be accessed only through 8R pores[26]. Hence, the peak at 53 ppm was further deconvoluted into four peaks at 60.5, 51, 53.5, and 56.6 ppm to identify the different chemical environment of Al in PLS-3 [Supplementary Figure 4 and Table 2], which are related to framework Al sited in T1, T2, T3 and T4, respectively[26]. The AlF species in T2 sites gradually increased from 40.5% to 68.5% with increasing Si/Al ratio. The proportion of AlF in T4 sites rose from 15.1% to 22.5% with the Si/Al ratio of 50-200, and then slightly reduced to 18.7% when increasing the Si/Al ratio to 250. However, the percentage in T3 sites decreased from 38.2% to 11.5%. The results proved that the spatial distribution of AlF sites can be tuned by the regulation of the Si/Al ratio in PLS-3 zeolite, and AlF preferred to locate in T2 and T4 sites at relatively low Al content.
Figure 3. (A) 27Al MAS NMR spectra and (B) IR spectra in the hydroxyl-stretching region of PLS-3 zeolites with different Al contents. MAS NMR: Magic angle spinning nuclear magnetic resonance; IR: infrared.
Framework Al distributions in different PLS-3 zeolites achieved via the deconvolution of 27Al MAS NMR spectra
Zeolite | T1 (60.5 ppm) | T2 (51.0 ppm) | T3 (53.5 ppm) | T4 (56.6 ppm) |
PLS-3-50 | 6.2 | 40.5 | 38.2 | 15.1 |
PLS-3-100 | 2.5 | 53.7 | 26.0 | 17.8 |
PLS-3-200 | 3.2 | 61.8 | 12.5 | 22.5 |
PLS-3-250 | 1.5 | 68.3 | 11.5 | 18.7 |
The acidity in PLS-3 zeolites was further investigated by hydroxyl-stretching IR spectra [Figure 3B]. Two main absorption bands were observed at 3,743 and 3,602 cm-1, which are attributed to the terminal Si–OH and the bridging hydroxyl groups (Si–OH–Al), respectively. [25] The intensity of Si–OH–Al groups gradually decreased with increasing Si/Al ratio, in consistent with 27Al MAS NMR spectra. Furthermore, NH3-TPD curves give the acid amount in PLS-3 zeolites [Figure 4A and Table 3]. The NH3-TPD curves show two desorption peaks at relatively low (about 423 K) and high temperatures (about 573 K), which are generally attributed to the desorption of NH3 on weak acid sites (WAS) and strong acid sites (SAS), respectively. The amounts of WAS and SAS also dropped from 142 and 88 μmol·g-1 to 20 and 28 μmol·g-1, respectively, with the Si/Al ratio increased from 50 to 300. Moreover, the Py-IR spectra were used to analyze the content of Lewis acid site (LAS) and Brønsted acid site (BAS) after the desorption of physically adsorbed pyridine at 423 K [Figure 4B and Table 3]. The bands at 1,543 and 1,455 cm-1 correspond to BAS and LAS, respectively[33]. The band at ~1,490 cm-1 is attributed to both BAS and LAS, and the band at 1,445 cm-1 is due to hydrogen-bonded pyridine. The contents of BAS and LAS were dramatically reduced with increasing Si/Al ratio, consistent with NH3-TPD results.
Figure 4. (A) NH3-TPD curves and (B) Py-IR spectra of PLS-3 zeolites with different Si/Al ratios. Py-IR spectra were measured after desorption of pyridine at 423 K. TPD: Temperature-programmed desorption; Py-IR: pyridine-adsorption infrared spectroscopy.
The acid amounts of PLS-3 zeolites with different Si/Al ratios
Sample | WAS[a] (μmol·g-1) | SAS[b] (μmol·g-1) | BAS[c] (μmol·g-1) | LAS[c] (μmol·g-1) |
PLS-3-50 | 142 | 88 | 118 | 47 |
PLS-3-100 | 96 | 82 | 90 | 21 |
PLS-3-200 | 46 | 53 | 60 | 16 |
PLS-3-250 | 31 | 33 | 41 | 11 |
PLS-3-300 | 20 | 28 | 18 | 3 |
Catalytic performance of syngas conversion
The PLS-3-x zeolites were mixed with Zn2Al3O4 oxide to catalyze the syngas conversion reaction. The acidity of PLS-3 zeolites could be tuned by varying framework Al content, and the effect of acidity on the product selectivity was further studied by physically mixing PLS-3-x zeolites with Zn2Al3O4 under the reaction condition at a space velocity of 6,000 mL·g-1·min-1, 623 K, and 1.0 MPa. As shown in Figure 5 and Table 4, the Si/Al ratio of PLS-3 zeolite had little effect on the CO conversion and the CO2 selectivity, which remained almost unchanged. The selectivity of ethylene dramatically increased from 23.2% to 45.0%, but C6+ and C2-50 selectivity decreased with increasing the Si/Al ratio from 50 to 250, thus leading to evidently increased E/P and O/P ratios from 3.6 to 7.0 and from 4.5 to 21.2, respectively. However, the catalytic performance became worse for sample PLS-3-300, showing the high CH4 selectivity of 12.5% and DME selectivity of 3.1%, due to the difficulty in transforming intermediates over insufficient acid sites. Therefore, using PLS-3-250 as zeolite component combined with Zn2Al3O4 oxide gave the optimal catalytic performance in the syngas conversion reaction, that is high selectivity of C2-5= (78.5%) and ethylene (45%) and high E/P (7.0) and O/P (21.2) ratios, which breaks the ASF distribution [Supplementary Figure 5]. Moreover, the bifunctional Zn2Al3O4/PLS-3 catalysts for syngas conversion have a higher selectivity of ethylene, O/P and E/P ratio with comparison to the SAPO-type zeolite-based catalysts [Supplementary Table 1]. We speculated that the PLS-3 zeolite with fewer acid sites and more AlF sited in the T2/T4 sites at a lower Al content may suppress the hydrogen transfer and aromatization reactions, thus leading to the high C2-5= and ethylene. These results indicated that the acidity of PLS-3 zeolite significantly affected the product selectivity of syngas conversion reaction. Furthermore, the CO conversion and product selectivity were not significantly changed within 100 h over Zn2Al3O4/PLS-3-250 catalyst [Figure 6], indicating that this catalyst was durable. However, the catalytic performance gradually decreased after 100 h, accompanied by a significant increase in methane selectivity and a dramatic reduction in ethylene and C2-5= selectivity.
Figure 5. (A) Effect of Si/Al ratio of PLS-3 on the catalytic performance of Zn2Al3O4/PLS-3-x for syngas conversion; (B) Dependence of E/P and O/P ratios on the Si/Al ratio of PLS-3. Reaction conditions: catalyst, 0.25 g; metal oxide/zeolite mass ratio, 3:2; temperature, 623 K; pressure, 1 MPa; the WHSV of syngas (H2/CO volume ratio = 1:1), 6,000 mL·g-1·h-1; TOS, 10 h. E/P: Ethylene to propylene; O/P: olefin to paraffin; WHSV: weight hourly space velocity; TOS: time on stream.
Figure 6. CO conversion and product selectivity with TOS over the bifunctional composite catalyst of Zn2Al3O4/PLS-3-250. Reaction conditions: see Figure 5. TOS: Time on stream.
Catalytic activity of various Zn2Al3O4/PLS-3 catalysts in syngas conversion reaction
Sample | CO conv. (%) | CO2 sel. (%) | Product distribution (%) | DME (%) | E/P | O/P | ||||
CH4 | C2= | C2-5= | C2-50 | C6+ | ||||||
PLS-3-50 | 11.1 | 28.1 | 4.6 | 23.2 | 57.6 | 12.9 | 24.9 | - | 3.6 | 4.5 |
PLS-3-100 | 10.9 | 22.4 | 5.3 | 25.6 | 60.7 | 9.9 | 24.1 | - | 4.1 | 6.2 |
PLS-3-200 | 10.9 | 28.7 | 7.0 | 29.2 | 67.5 | 4.1 | 21.4 | - | 5.9 | 16.3 |
PLS-3-250 | 11.0 | 24.7 | 6.6 | 45.0 | 78.5 | 3.7 | 11.2 | - | 7.0 | 21.2 |
PLS-3-300 | 8.9 | 24.4 | 12.5 | 36.0 | 68.9 | 7.6 | 11.0 | 3.1 | 6.1 | 9.1 |
As shown in Supplementary Figures 6-8 and Supplementary Table 2, we investigated the effects of reaction conditions of weight hourly space velocity (WHSV), temperature, and proximity between two components on the syngas conversion performance using the bifunctional catalysts composed of Zn2Al3O4 and PLS-3-250. Increasing the WHSV of syngas from 3,000 to 7,200 mL·g-1·h-1 [Supplementary Figure 6 and Supplementary Table 2], the CO conversion was decreased (from 13.3% to ~11%) and the selectivity of ethylene (from 29% to >40%) dramatically increased, while the selectivity of C2-50 and C6+ was reduced. Among the hydrocarbon products, the E/P (from 5.4 to 7.1) and O/P (from 16.1 to 23.6) ratios also increased with WHSV. However, the selectivity of long-chain olefins (such as the butene and pentene) was reduced with increasing the WHSV [Supplementary Figure 6A], and they were produced from the ethylene oligomerization reaction. These results indicated that increasing the WHSV of syngas promoted the diffusion of olefin products, which depressed the further hydrogen transfer and ethylene oligomerization to form the alkanes and long-chain hydrocarbons. The reaction temperature showed a more significant effect on the catalytic performance of Zn2Al3O4/PLS-3-250 in the syngas conversion reaction [Supplementary Figure 7 and Supplementary Table 2]. The CO conversion and CO2 selectivity increased with temperature from 603 to 663 K. Furthermore, the higher methane (20.6%) and DME (1.2%) selectivity were observed at a lower temperature of 603 K. The result indicated that the lower temperature is unfavorable for the conversion of methanol intermediate. Meanwhile, the selectivity of C6+ exhibited a volcanic trend. At a lower temperature (603 K), the difficulty in converting methanol intermediates led to high CH4 selectivity but low C6+ selectivity, while at higher temperatures (663 K), the suppression of chain growth reactions resulted in low C6+ selectivity. In addition, when the temperature was increased from 623 to 663 K, the CH4 and C2-50 were promoted, but C2-5= and ethylene selectivity were reduced as a result of the enhancement of hydrogen transfer reactions at higher temperatures[3,5], resulting in the highest E/P (7.0) and O/P (21.2) ratios at 623 K. With comprehensive consideration of selectivity and conversion, the optimal reduction temperature was 623 K.
In addition, the proximity between two components of zeolite and metal oxide was reported to affect the product distribution in previous studies[5,25]. We thus compared the catalytic performance among three integration manners, including dual bed, granule mixing and mortar mixing [Supplementary Figure 8 and Supplementary Table 2]. Over a dual-bed configuration with PLS-3-250 zeolite downstream from Zn2Al3O4 oxide, a lower C2-5= (33.3%) and ethylene (11%) selectivity but a higher CO2 (~44%) and CH4 (~34%) selectivity were observed at low CO conversion (6.7%), and the E/P and O/P ratios were only 2.0 and 2.2, respectively. For the granule stacking mode, a higher C2-5= selectivity (78.5%), a higher ethylene selectivity (45%) in olefins, and a lower selectivity of CO2 (24%) were achieved at CO conversion of 11%. The powder of metal oxide and zeolite was mixed and then compressed into pellets to achieve the increased contact distance. The CH4 and C2-50 selectivity significantly increased, but the C2-5= selectivity decreased to 68%; thus, the O/P ratio dramatically dropped from 21.2 to 7.2. Meanwhile, the ethylene selectivity was slight increased (46%) and that of long-chain C6+ was decreased. These results indicated that too far spatial distance of the two components was unfavorable for the syngas conversion due to the difficulty in transferring intermediate, while the too close spatial distance may cause partial shielding of acid sites in PLS-3 zeolite[34]. Hence, the granule mixing of two components provided the most suitable spatial proximity to obtain high selectivity of C2-5= and ethylene.
Reaction mechanism of syngas conversion catalyzed by Zn2Al3O4/PLS-3
The investigation of coke precursors helps to understand the reaction mechanism in zeolite-catalyzed syngas conversion[25]. The carbonaceous species deposited on the spent PLS-3 catalysts were investigated by thermogravimetry (TG) [Figure 7A]. The percentage of coke deposition gradually decreased from 4.2% to 2.2% with the decrease of Al content in PLS-3 zeolite, suggesting that the weakened acidity of PLS-3 zeolite can suppress the generation of carbon deposition for the syngas conversion reaction. The soluble coke species confined in the pore channels of used PLS-3 zeolite were further analyzed by the GC-MS technique [Figure 7B]. Polymethylbenzene (PMB) species, including trimethylbenzene, tetramethylbenzene, pentamethylbenzene, hexamethylbenzene, naphthalenes, and phenanthrenes, were detected in spent catalysts, potentially serving as coke precursors that contribute to catalyst deactivation. These coke precursors were dramatically reduced over the PLS-3 zeolite with a higher Si/Al ratio, consistent with the TG result. Moreover, the oxygenate species, such as dimethylbenzaldehyde, were detected, probably related to the intermediate species of syngas conversion in PLS-3 zeolite[35], and their amounts were also decreased with increasing Si/Al ratios.
Figure 7. (A) TG curves and (B) GC-MS analysis of the hydrocarbons on the spent catalysts. *The internal standard. TG: Thermogravimetry; GC-MS: gas chromatography-mass spectrometry.
For the bifunctional catalyst of metal oxide and zeolite used in syngas conversion, the zeolite component is responsible for the transformation of intermediate into desired hydrocarbons. It is currently recognized in research that methanol is the main intermediate for the bifunctional catalytic system of ZnAlOx-zeolite[5,25]. Hence, the reaction mechanism of syngas conversion over Zn2Al3O4/PLS-3-250 was further studied by the probe reaction of methanol conversion using the in-situ IR spectra as shown in Figure 8. Methanol was first introduced into the wafer of PLS-3-250 zeolite under the He atmosphere at 623 K. The stretching vibration of C–H of surface methoxy species (2,980, 2,966 and 1,455 cm-1) and C–H bending vibration of -CH3 (2,952 and 2,923 cm-1) were observed, while the band at 2,891 cm-1 was related to the DME molecules hydrogen-boned to Si–OH[36]. Meanwhile, the symmetrical stretching vibration of =CH2 at 1,463 and 1,417 cm-1 and the C–H vibration of olefin species at 2,876, 2,855 and 2,817 cm-1 were observed[25,37]. The negative bands at 3,500-3,800 cm-1 were related to the interaction of hydrocarbon species with hydroxyl-stretching (-OH) groups[25]. We found that the intensity of signals corresponding to olefin species at 2,876, 2,855 and
Figure 8. In-situ FTIR spectra when gas-phase methanol was introduced to PLS-3-250 zeolite under the He or syngas flow at temperature of 623 K. Methanol was continuously brought into in-situ chamber under He flow for 10 min, and then under syngas flow for another 10 min. (A) Enlarge view of the region at 3,800-2,000 cm-1; (B) Enlarge view of the region at 1,800-1,400 cm-1. FTIR: Fourier transform infrared spectroscopy.
Based on the above results, we propose a possible formation mechanism of key intermediates in the syngas conversion over the bifunctional Zn2Al3O4/PLS-3 catalyst. As shown in Scheme 1, methanol carbonylation is the dominant route to form olefins (especially ethylene) for the PLS-3 zeolite with FER topology. The syngas is activated to generate the methanol/methoxy intermediate over Zn2Al3O4 oxide[5]. Subsequently, the acetyl group is converted into a methoxy group by insertion of CO over the acidic Si–OH–Al groups of PLS-3 zeolite. The formed MA and AA species are further converted into ethylene, and then longer-chain olefins (such as propylene, butene, and pentene) are formed by the oligomerization of ethylene. The previous study has indicated that the T2/T4 sites in FER are beneficial to the insertion of CO into the surface methoxy group to produce acetyl species for promoting the carbonylation reaction[26]. In this work, the proportion of Al in T2/T4 sites gradually raised with Si/Al ratios of PLS-3 zeolite as evidenced by 27Al MAS NMR investigation [Supplementary Figure 3 and Table 2]. Figure 9A showed that the ethylene selectivity and E/P ratio gradually increased with the proportion of T2 and T4 acid sites over the PLS-3 zeolite. Hence, we speculated that the T2 and T4 sites are the main active centers, contributing to the carbonylation route in Zn2Al3O4/PLS-3-catalyzed syngas conversion reaction. Moreover, the olefin selectivity and O/P ratio also gradually increased with the decreasing acid amount in PLS-3 as measured by Py-IR spectra and NH3-TPD curves [Figure 9B]. The result indicated that weakened acidity favors the formation of olefins by suppressing the hydrogen transfer reactions to form the alkanes. Hence, the high E/P and O/P ratios are presumably attributed to two main reasons: the suitable acid site distribution and acid density of PLS-3 zeolite, which are achieved by tuning the Si/Al ratio.
Figure 9. (A) Dependence of ethylene selectivity and E/P ratio on the proportion of (T2 + T4) sites; and (B) relationship between the olefin selectivity or O/P ratio and the acid amount. The (T2 + T4) site proportion was obtained from the 27Al MAS NMR spectra. The acid amount was measured by Py-IR and NH3-TPD techniques. E/P: Ethylene to propylene; O/P: olefin to paraffin; MAS NMR: magic angle spinning nuclear magnetic resonance; Py-IR: pyridine-adsorption infrared spectroscopy; TPD: temperature-programmed desorption.
CONCLUSIONS
FER-type high silica PLS-3 zeolites with nanorod crystals and tunable acidity are applied to construct bifunctional syngas conversion catalysts for selective production of olefins by combining with the Zn2Al3O4 oxide. The acid site distribution and acidity density of PLS-3 zeolite were achieved by tuning the Si/Al ratio from 50 to 300. The decreased acid amount of PLS-3 zeolite with lower Al content restrains the side reactions (such as hydrogen transfer and aromatization reactions) and the carbon deposition, while insufficient acid sites lead to decreased catalytic performance due to difficulty in transforming methanol intermediates. Furthermore, MA, AA and acetyl intermediates were observed in Zn2Al3O4/PLS-3-catalyzed syngas conversion reaction via the in-situ IR spectra, indicating the methanol carbonylation was identified as the dominant route. Meanwhile, a higher proportion of framework Al located in T2 and T4 sites of PLS-3 zeolite at low Al content favored the formation of ethylene by promoting the carbonylation route. Precisely controlling the acid property of PLS-3 zeolite allows the achievement of selective formation of olefins, providing the highest C2-5= selectivity of 78.5%, especially high ethylene selectivity of 45.0%, and high E/P (7.0) and O/P (21.2) ratios. This work provides a highly efficient zeolite catalyst for the conversion of syngas to olefins and proposes carbonylation mechanism for syngas conversion over oxide-zeolite bifunctional catalytic system.
DECLARATIONS
Authors’ contributions
Conception and design of the study: Xu, H.; Wu, P.
Data collection and analysis: Tuo, J.
Sample preparation: Tuo, J.; Gong, X.; Yang, Q.; Sheng, Z.; Guan, Y.
Paper writing and review: Tuo, J.; Xu, H.; Wu, P.
Availability of data and materials
The detailed materials and methods in the experiment were listed in the Supplementary Materials. Other raw data that support the findings of this study are available from the corresponding author upon reasonable request.
Financial support and sponsorship
We gratefully acknowledge the financial support from NSFC of China (Grant Nos. 22222201 and 22272054) and the National Key Research and Development Program of China (2023YFB3810602 and 2021YFA1501401).
Conflicts of interest
All authors declared that there are no conflicts of interest.
Ethical approval and consent to participate
Not applicable.
Consent for publication
Not applicable.
Copyright
© The Author(s) 2025.
Supplementary Materials
REFERENCES
1. Han, Q.; Gao, P.; Chen, K.; et al. Synergistic interplay of dual active sites on spinel ZnAl2O4 for syngas conversion. Chem 2023, 9, 721-38.
2. Lyu, S.; Wang, Y.; Qian, J.; et al. Role of residual CO molecules in OX–ZEO relay catalysis for syngas direct conversion. ACS. Catal. 2021, 11, 4278-87.
3. Wang, S.; Wang, P.; Shi, D.; et al. Direct conversion of syngas into light olefins with low CO2 emission. ACS. Catal. 2020, 10, 2046-59.
4. Torres Galvis, H. M.; de Jong, K. P. Catalysts for production of lower olefins from synthesis gas: a review. ACS. Catal. 2013, 3, 2130-49.
5. Ren, L.; Zhang, J.; Wang, B.; et al. Syngas to light olefins over ZnAlOx and high-silica CHA prepared by boron-assisted hydrothermal synthesis. Fuel 2022, 307, 121916.
6. Torres Galvis, H. M.; Bitter, J. H.; Khare, C. B.; Ruitenbeek, M.; Dugulan, A. I.; de Jong, K. P. Supported iron nanoparticles as catalysts for sustainable production of lower olefins. Science 2012, 335, 835-8.
7. Zhong, L.; Yu, F.; An, Y.; et al. Cobalt carbide nanoprisms for direct production of lower olefins from syngas. Nature 2016, 538, 84-7.
8. Sun, Q.; Wang, N.; Yu, J. Advances in catalytic applications of zeolite-supported metal catalysts. Adv. Mater. 2021, 33, e2104442.
9. Song, F.; Yong, X.; Wu, X.; et al. FeMn@HZSM-5 capsule catalyst for light olefins direct synthesis via Fischer-Tropsch synthesis: Studies on depressing the CO2 formation. Appl. Catal. B. Environ. 2022, 300, 120713.
10. Qiu, T.; Wang, L.; Lv, S.; et al. SAPO-34 zeolite encapsulated Fe3C nanoparticles as highly selective Fischer-Tropsch catalysts for the production of light olefins. Fuel 2017, 203, 811-6.
11. Ni, Y.; Liu, Y.; Chen, Z.; et al. Realizing and recognizing syngas-to-olefins reaction via a dual-bed catalyst. ACS. Catal. 2019, 9, 1026-32.
12. Jiao, F.; Li, J.; Pan, X.; et al. Selective conversion of syngas to light olefins. Science 2016, 351, 1065-8.
13. Cheng, K.; Gu, B.; Liu, X.; Kang, J.; Zhang, Q.; Wang, Y. Direct and highly selective conversion of synthesis gas into lower olefins: design of a bifunctional catalyst combining methanol synthesis and carbon–carbon coupling. Angew. Chem. Int. Ed. Engl. 2016, 55, 4725-8.
14. Wang, C.; Wang, Y.; Xie, Z. Methylation of olefins with ketene in zeotypes and its implications for the direct conversion of syngas to light olefins: a periodic DFT study. Catal. Sci. Technol. 2016, 6, 6644-9.
15. Pan, X.; Jiao, F.; Miao, D.; Bao, X. Oxide-zeolite-based composite catalyst concept that enables syngas chemistry beyond Fischer-Tropsch synthesis. Chem. Rev. 2021, 121, 6588-609.
16. Chen, K.; Wang, F.; Wang, Y.; et al. Relay catalysis for highly selective conversion of methanol to ethylene in syngas. JACS. Au. 2023, 3, 2894-904.
17. Zhu, Y.; Pan, X.; Jiao, F.; et al. Role of manganese oxide in syngas conversion to light olefins. ACS. Catal. 2017, 7, 2800-4.
18. Liu, X.; Zhou, W.; Yang, Y.; et al. Design of efficient bifunctional catalysts for direct conversion of syngas into lower olefins via methanol/dimethyl ether intermediates. Chem. Sci. 2018, 9, 4708-18.
19. Su, J.; Zhou, H.; Liu, S.; et al. Syngas to light olefins conversion with high olefin/paraffin ratio using ZnCrOx/AlPO-18 bifunctional catalysts. Nat. Commun. 2019, 10, 1297.
20. Li, G.; Jiao, F.; Pan, X.; et al. Role of SAPO-18 acidity in direct syngas conversion to light olefins. ACS. Catal. 2020, 10, 12370-5.
21. Su, J.; Zhang, L.; Zhou, H.; et al. Unveiling the anti-trap effect for bridging intermediates on ZnAlOx/AlPO-18 bifunctional catalysts to boost syngas to olefin conversion. ACS. Catal. 2023, 13, 2472-81.
22. Wang, M.; Kang, J.; Xiong, X.; et al. Effect of zeolite topology on the hydrocarbon distribution over bifunctional ZnAlO/SAPO catalysts in syngas conversion. Catal. Today. 2021, 371, 85-92.
23. Jiao, F.; Pan, X.; Gong, K.; Chen, Y.; Li, G.; Bao, X. Shape-selective zeolites promote ethylene formation from syngas via a ketene intermediate. Angew. Chem. Int. Ed. Engl. 2018, 57, 4692-6.
24. Jiao, F.; Bai, B.; Li, G.; et al. Disentangling the activity-selectivity trade-off in catalytic conversion of syngas to light olefins. Science 2023, 380, 727-30.
25. Tuo, J.; Fan, Y.; Wang, Y.; et al. Promoting syngas to olefins with isolated internal silanols-enriched Al-IDM-1 aluminosilicate nanosheets. Angew. Chem. Int. Ed. Engl. 2023, 62, e202313785.
26. Tuo, J.; Wang, J.; Gong, X.; et al. Ferrierite nanosheets with preferential Al locations as catalysts for carbonylation of dimethyl ether. Fuel 2024, 357, 130001.
27. Dai, W.; Ruaux, V.; Deng, X.; et al. Synthesis and catalytic application of nanorod-like FER-type zeolites. J. Mater. Chem. A. 2021, 9, 24922-31.
28. Yang, B.; Jiang, J.; Xu, H.; Liu, Y.; Peng, H.; Wu, P. Selective skeletal isomerization of 1-butene over FER-type zeolites derived from PLS-3 lamellar precursors. Appl. Catal. A. Gen. 2013, 455, 107-13.
29. Tuo, J.; Lv, J.; Fan, S.; et al. One-pot synthesis of [Mn,H]ZSM-5 and the role of Mn in methanol-to-propylene reaction. Fuel 2022, 308, 121995.
30. Tuo, J.; Fan, S.; Yang, N.; et al. Direct synthesis of [B,H]ZSM-5 by a solid-phase method: AlF siting and catalytic performance in the MTP reaction. Catal. Sci. Technol. 2020, 10, 7034-45.
31. Liu, R.; Zeng, S.; Sun, T.; et al. Selective removal of acid sites in mordenite zeolite by trimethylchlorosilane silylation to improve dimethyl ether carbonylation stability. ACS. Catal. 2022, 12, 4491-500.
32. Gong, Y.; Tuo, J.; Li, S.; et al. Direct synthesis of IDM-1 aluminosilicate nanosheets with improved MTP performance. Chem. Commun. 2023, 59, 724-7.
33. Li, S.; Peng, R.; Wan, Z.; et al. A nanostrips-assemble morphology of ZSM-5 zeolite for efficient propylene production from methanol conversion. ACS. Sustain. Chem. Eng. 2023, 11, 10274-83.
34. Ding, Y.; Jiao, F.; Pan, X.; et al. Effects of proximity-dependent metal migration on bifunctional composites catalyzed syngas to olefins. ACS. Catal. 2021, 11, 9729-37.
35. Xie, M.; Fang, X.; Liu, H.; et al. Cyclic oxygenate-based deactivation mechanism in dimethyl ether carbonylation reaction over a pyridine-modified H-MOR catalyst. ACS. Catal. 2023, 13, 14327-33.
36. Xiong, Z.; Zhan, E.; Li, M.; Shen, W. DME carbonylation over a HSUZ-4 zeolite. Chem. Commun. 2020, 56, 3401-4.
37. Ma, K.; Zhao, S.; Dou, M.; Ma, X.; Dai, C. Enhancing the stability of methanol-to-olefins reaction catalyzed by SAPO-34 zeolite in the presence of CO2 and oxygen-vacancy-rich ZnCeZrOx. ACS. Catal. 2024, 14, 594-607.
38. Liu, J.; Xue, H.; Huang, X.; et al. Stability enhancement of H-mordenite in dimethyl ether carbonylation to methyl acetate by pre-adsorption of pyridine. Chin. J. Catal. 2010, 31, 729-38.
39. Zhou, H.; Zhu, W.; Shi, L.; et al. In situ DRIFT study of dimethyl ether carbonylation to methyl acetate on H-mordenite. J. Mol. Catal. A. Chem. 2016, 417, 1-9.
40. Cheung, P.; Bhan, A.; Sunley, G.; Law, D.; Iglesia, E. Site requirements and elementary steps in dimethyl ether carbonylation catalyzed by acidic zeolites. J. Catal. 2007, 245, 110-23.
Cite This Article
How to Cite
Download Citation
Export Citation File:
Type of Import
Tips on Downloading Citation
Citation Manager File Format
Type of Import
Direct Import: When the Direct Import option is selected (the default state), a dialogue box will give you the option to Save or Open the downloaded citation data. Choosing Open will either launch your citation manager or give you a choice of applications with which to use the metadata. The Save option saves the file locally for later use.
Indirect Import: When the Indirect Import option is selected, the metadata is displayed and may be copied and pasted as needed.
About This Article
Copyright
Data & Comments
Data
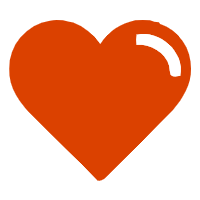
Comments
Comments must be written in English. Spam, offensive content, impersonation, and private information will not be permitted. If any comment is reported and identified as inappropriate content by OAE staff, the comment will be removed without notice. If you have any queries or need any help, please contact us at [email protected].