Bandgap engineering of Zn1-xCdxS for glycerol photo(electro)reforming into glyceric acid with hydrogen coproduction
Abstract
The surplus of glycerol generation from biodiesel fuel production has stimulated the development of efficient technology to realize the sustainability of biomass valorization. Herein, we demonstrate the glycerol valorization by mild photocatalytic and photoelectrocatalytic approaches. Glycerol photo(electro)reforming is realized on the well-designed Zn1-xCdxS solid solution photocatalysts. By continuously changing the ratio of Zn/Cd, Zn1-xCdxS is endowed with a regulatable bandgap structure, which finely controls the redox potential and light absorption. The spontaneous formation of homojunction by hexagonal wurtzite (WZ) and zinc-blende (ZB) facilitates spatial charge separation. As a result, Zn1-xCdxS exhibits the dual ability to simultaneously produce hydrogen and glyceric acid by the electrons and holes, respectively. The theoretical calculation and in-situ spectroscopy analysis reveal the prominent features of hydrogen evolution and glycerol oxidation into glyceric acid on the optimized Zn0.5Cd0.5S. This work provides a good example for glycerol valorization into sustainable fuels and chemicals by rationally designing dually functional photocatalysts.
Keywords
INTRODUCTION
Biomass valorization to produce value-added chemicals or fuels provides a sustainable alternative to release the pressure from the depletion of traditional fossil energy[1-5]. Biodiesel fuel production from biomass valorization is one of the most mature technologies and its market demand is increasing annually[6-8]. However, the production of biodiesel fuel is always accompanied by the coproduction of crude glycerol (~10% of glycerol) as a byproduct, and it is predicted that six million tons of glycerol will be produced in 2025[9,10]. Due to the limited market size of glycerol, the valorization of glycerol to value-added chemicals and gas fuels has been attracting extensive attention[11-13].
Steam reforming, pyrolysis and biological processes are the most common strategies for current glycerol conversion into gaseous and/or liquid products[14-18]. However, these processes always suffer from high energy input and/or low product selectivity. Photocatalytic and photoelectrocatalytic glycerol utilization with solar energy, as the driving force, holds outstanding features of mild reaction conditions and high product selectivity[19-23]. Currently, glycerol valorization by photo(electro)catalysis can be divided into two processes: photooxidation (with O2) and photoreforming (without O2). The former uses O2 or species derived from O2 as oxidants to convert glycerol, while the latter primarily uses glycerol as an electron donor to produce hydrogen[24-27]. By photocatalysis, glycerol can be converted into a vast range of value-added liquid and gaseous products such as dihydroxyacetone, glyceraldehyde, glyceric acid, glycolic acid, CO and H2 due to its highly functionalized nature from the three hydroxyl groups[28-31]. Glyceric acid, the important intermediate for light industries, is commonly produced via selective oxidation of glycerol under alkali conditions and in presence of noble metals[32-34]. Herein, rationally designing photocatalysts to achieve selective glyceric acid production from glycerol photo(electro)reforming along with sustainable H2 production holds promising perspectives from industrial and academic points of view.
The fundamental process of glycerol photo(electro)reforming is that the photogenerated electrons reduce protons from glycerol or water to produce hydrogen while the glycerol as an electron donor releases protons and timely consumes the photogenerated holes[35,36]. Improving the separation efficiency of photogenerated electrons and holes is apparently one of the approaches to boost glycerol conversion and hydrogen production. However, the selective glycerol conversion cannot be simultaneously guaranteed during the photo(electro)reforming process. As the redox ability of photocatalysts can be regulated by changing the band gap structure, it provides a possibility to selectively convert glycerol along with hydrogen coproduction by bandgap engineering strategy[37]. Fine regulation of the band gap structure of semiconductors is a kind of challenge. Until now, quantum size effect and doping have been revealed as strategies to slightly change the band gap structure[38,39]. However, these always involve complicated procedures and introduction of defects as recombination centers for electrons and holes[40]. Solid solutions stand out because their band gap structure can be continuously regulated by changing the ratio of solute atoms in the solvent lattice[41,42]. In particular, the cost-effective Zn1-xCdxS, a typical solid solution, has been widely investigated as a photocatalyst due to tunable band structure and compositions of exposed crystal facets[43-45]. Besides, the well-established homojunction structure by introducing twinning superlattice in Zn1-xCdxS nanocrystal also realizes efficient charge separation to boost the quantum yield[46,47]. Glycerol photoreforming has also been demonstrated over Zn1-xCdxS but only with hydrogen production[48]. The liquid or other gaseous products by photo(electro)reforming approach have not been systematically investigated to reveal the detailed reaction pathway.
Herein, to fill this gap, we design and fabricate a series of Zn1-xCdxS photocatalysts with twinning superlattice and homojunction structure, which benefit the separation efficiency of photogenerated electrons and holes. The redox ability and visible light absorption capability can be continuously regulated by changing the Cd/Zn ratio. Specifically, increasing the Cd/Zn ratio leads to enhanced visible light absorption, but the narrow bandgap structure is accompanied by limited redox ability. As a result, Zn1-xCdxS photocatalysts exhibit excellent H2 production, which strongly depends on the Cd/Zn ratio. Glyceric acid is selectively produced from glycerol oxidation by the photogenerated holes. Besides, the glycerol photoelectroreforming test is also performed and Zn0.5Cd0.5S exhibits good photoelectrocatalytic activity. This work demonstrates a good example for simultaneous production of gas fuels and value-added chemicals from glycerol photoreforming.
EXPERIMENTAL
Detailed experimental materials and methods were included in Supplementary Materials.
RESULTS AND DISCUSSION
Zn1-xCdxS solid solutions with different Zn/Cd ratios were prepared by a hydrothermal process with zinc acetate dihydrate, cadmium acetate dihydrate and thioacetamide as precursors. The obtained samples exhibited different colors from white (ZnS) gradually to orange (CdS), indicating the enhanced visible light absorbance with the increasing cadmium content [Figure 1A]. The obtained Zn1-xCdxS solid solutions exhibited crystalline particles [Figure 1B and Supplementary Figure 1]. The high-angle annular dark-field scanning transmission electron microscopy (HAADF-STEM) image and corresponding elemental mappings reveal the uniform distribution of Zn, Cd and S in the crystals [Figure 1C]. High-resolution transmission electron microscopy (HRTEM) was performed to check the fine crystalline structure at an atomic scale. The presence of both hexagonal wurtzite (WZ) structure and cubic zinc-blende (ZB) could be clearly observed in a single crystalline particle [Figure 1D]. The phase boundary could also be clearly identified. The crystal structures are further confirmed by the fast Fourier transform (FFT) patterns
Figure 1. (A) Schematic illustration of Zn1-xCdxS preparation; (B) a typical TEM image of Zn0.5Cd0.5S; (C) HAADF-STEM and corresponding elemental mappings of Zn0.5Cd0.5S; HR-TEM images of (D) WZ phase and ZB phase in a single crystal, (E) and (F) FFT patterns in the selected areas in (D); (G) ZB phase crystal and (H) WZ phase crystal; (I) HR-TEM image with the labeling of twin boundaries and elemental mappings at the atomic scale (inset). TEM: Transmission electron microscopy; HAADF-STEM: high-angle annular dark-field scanning transmission electron microscopy; HR-TEM: high-resolution transmission electron microscopy; WZ: wurtzite; ZB: zinc-blende; FFT: fast Fourier transform.
The change in crystal structure with different Zn/Cd ratios was tracked by X-ray diffraction [Figure 2A]. It is obvious that ZnS exhibits a typical cubic ZB crystal phase (JCPDS Card: 03-065-1691). With the introduction of Cd to gradually replace Zn in Zn1-xCdxS solid solutions, the crystal structure changes from cubic ZB phase to hexagonal WZ phase with a significant shift to a smaller diffraction angle of pristine ZnS (110) plane. Typical hexagonal WZ diffraction peaks at 24.8°, 26.4° and 28.2° are identified for pure CdS (100), (002) and (101) planes, respectively. Noticeably, the relative peak intensity of (002) of as-fabricated CdS is extremely higher than that of the reference pattern (JCPDS Card No. 01-077-2306), which is ascribed to the presence of cubic ZB crystal phase in CdS. The existence of a cubic phase in CdS could further be revealed by the diffraction peak at 30.5°, which corresponds to the (200) plane of cubic ZB CdS. The above results clearly demonstrate the formation of homojunction structure within Zn1-xCdxS solid solutions by random cubic ZB phase and hexagonal WZ phase. Another advantage of solid solution photocatalysts is their continuously adjustable bandgap structure with different constituents. All the photocatalysts exhibit direct semiconductor properties and pure ZnS shows the ability to only absorb ultraviolet (UV) light with an absorption edge at 332 nm [Figure 2B]. With the increasing loading of Cd to gradually replace Zn atoms, Zn1-xCdxS solid solutions show redshifts in light absorption edge, and pure CdS exhibits an absorption edge at 518 nm. According to Kubelka-Munk theory, the corresponding band gaps are 3.73, 3.10, 2.67, 2.53 and 2.39 eV for ZnS, Zn0.75Cd0.25S, Zn0.5Cd0.5S, Zn0.25Cd0.75S and CdS, respectively [Figure 2C].
Figure 2. (A) XRD patterns, (B) UV-vis diffuse reflectance spectra, (C) Tauc plots, (D) Mott-Schottky plots and (E) bandgap structures illustration of Zn1-xCdxS solid solutions; High-resolution XPS spectra of (F) Zn 2p, (G) Cd 3d and (H) S 2p of Zn1-xCdxS solid solutions. XRD: X-ray diffraction; UV-vis: ultraviolet-visible; XPS: X-ray photoelectron spectroscopy.
To clarify the exact positions of the bandgap structure of Zn1-xCdxS solid solutions, Mott-Schottky plots are recorded in a typical three-electrode system with photocatalyst on fluorine-doped tin oxide (FTO) as the working electrode, a Pt plate as the counter electrode and Ag/AgCl as the reference electrode. All the
The photocatalytic activity of as-prepared Zn1-xCdxS solid solutions was evaluated by glycerol photoreforming. Pristine Zn1-xCdxS solid solutions without any cocatalyst were first investigated for H2 evolution. All the samples exhibited almost linear relation of H2 amount with reaction time and the Zn/Cd ratio greatly affected the photocatalytic performance [Figure 3A]. Compared with bare CdS, with addition of Zn in crystal structure, samples exhibited significantly enhanced H2 production. This should be attributed to the formation of solid solution structure, which facilitates the spontaneously separation of photogenerated electrons and holes. This could be revealed by the enhanced photocurrent density and reduced electrochemical impedance of Zn0.5Cd0.5S compared to pristine CdS [Supplementary Figure 6]. Noticeably, a negligible amount of CO was also detected without any CO2 generation [Supplementary Figure 7]. The H2 evolution in the presence of Pt photodeposition still exhibited a linear relation with reaction time [Figure 3B]. The formation of a Schottky junction through in-situ introduction of Pt as a cocatalyst further boosted the photocatalytic H2 production for all the Zn1-xCdxS samples, except for ZnS, which is a UV-responsible material. Differently, Zn0.75Cd0.25S exhibited the highest H2 generation rate
Figure 3. Photocatalytic H2 generation of Zn1-xCdxS solid solutions from glycerol solution (A) without and (B) with Pt as cocatalyst, (C) corresponding H2 generation rates; (D) H2 generation of Zn0.5Cd0.5S with Pt from different glycerol concentration; (E) cycling test of
The photoelectrocatalytic performance was then investigated for Zn0.5Cd0.5S as it showed the compromised activity for both H2 production and glycerol oxidation. The film on FTO was endowed with a similar morphology of Zn0.5Cd0.5S compared to the powder but with significantly reduced crystal sizes. The thickness was measured to be around 1.08 μm [Supplementary Figure 11]. The variables of light irradiation and glycerol were applied for this test. The photocurrent densities were extremely low under dark conditions even with the addition of glycerol [Figure 4A]. Under the light irradiation, Zn0.5Cd0.5S exhibited an enhanced photocurrent density for water splitting and the photocurrent density was boosted to
Figure 4. (A) LSV curves of Zn0.5Cd0.5S in 0.1 M KOH solution with and without glycerol under dark and Xenon lamp illumination; (B) Transient response to the light irradiation at 1.2 V vs. RHE in 0.1 M KOH solution with 0.1 M glycerol; (C) Photocurrent density and calculated charge with increasing reaction time (insets are the photos of the working electrode before and after reaction; (D) Glycerol conversion and glyceric acid selectivity. LSV: Linear sweep voltammetry; RHE: reversible hydrogen electrode.
The theoretical calculation revealed that bandgap energies were 2.44, 1.76, 1.38, 1.11 and 1.05 eV for ZnS, Zn0.75Cd0.25S, Zn0.5Cd0.5S, Zn0.25Cd0.75S and CdS respectively [Figure 5A-E and Supplementary Figure 12]. The calculated bandgap energies were smaller than those obtained from UV-visible diffuse reflectance spectra but with a consistent trend of decline compared to the measured results. Subsequently, the hydrogen evolution reaction (HER) processes of five proportionally corresponding structures under the neutral condition were simulated [Figure 5F]. The effect of proton adsorption sites on free energy was also investigated on Zn0.75Cd0.25S, Zn0.5Cd0.5S and Zn0.25Cd0.75S. Pristine ZnS (ΔG = 0.46 eV) delivered smaller HER free energy than CdS (ΔG = 0.66 eV). However, the activity of the Cd site (ΔG = 0.21 eV) in Zn0.25Cd0.75S material was smaller than that of the Zn site (ΔG = 1.90 eV). In the comparison of Zn0.75Cd0.25S and
Figure 5. Band structure and atomic illustrations of Zn1-xCdxS: (A) ZnS, (B) Zn0.75Cd0.25S, (C) Zn0.5Cd0.5S, (D) Zn0.25Cd0.75S, (E) CdS, (F) the HER step-chart of Zn1-xCdxS with (111) facet, (G) oxidation of glycerol with Zn0.75Cd0.25S and Zn0.5Cd0.5S and corresponding structure inset; (H) and (I) Density of states graphs for first and second dehydrogenation step; (J) In situ DRIFTS spectra of glycerol oxidation on Zn0.5Cd0.5S surface. HER: Hydrogen evolution reaction; DRIFTS: diffuse reflectance infrared Fourier transform spectroscopy.
The density of states was further analyzed to confirm the prominent features of Zn0.5Cd0.5S in the whole oxidation process of glycerol into glyceric acid. For the first dehydrogenation step on the primary carbon atom of glycerol, the O2p orbital was a major part of bonding to the Zn3d orbital in Zn0.75Cd0.25S and
CONCLUSIONS
In summary, Zn1-xCdxS solid solutions have been successfully synthesized with bandgap engineering for glycerol photoreforming. The changeable bandgap structure regulates redox potential and light absorption. The spontaneous formation of homojunction by hexagonal WZ and ZB facilitates spatial charge separation. As a result, a considerable amount of H2 is produced by photogenerated electrons while glycerol is selectively oxidized into glyceric acid by the photogenerated holes. The as-fabricated material also exhibits excellent performance in glycerol photoelectroreforming process. This present work demonstrates an example for glycerol valorization into sustainable H2 along with value-added chemicals coproduction by mild photo(electro)catalytic technology.
DECLARATIONS
Authors’ contributions
Made substantial contributions to conception and design of the study, performed data analysis and interpretation, and wrote the draft of the manuscript: Yu, X.; Liu, D.; Zhu, B.
Performed DFT calculation: Fu, C.; Yong, X.
Discussed and revised the manuscript: Hu, J.; Zhao, H.; Chen, Z.
Availability of data and materials
Detailed experimental materials and methods were included in Supplementary Materials, and the data supporting the findings of this study are available within its Supplementary Materials.
Financial support and sponsorship
This work was financially supported by the Canada First Research Excellence Fund (CFREF) and research funding from the Ningbo Institute of Digital Twin, Eastern Institute of Technology, Ningbo.
Conflicts of interest
All authors declared that there are no conflicts of interest.
Ethical approval and consent to participate
Not applicable.
Consent for publication
Not applicable.
Copyright
© The Author(s) 2025.
REFERENCES
1. Liu, Z.; Wang, K.; Chen, Y.; Tan, T.; Nielsen, J. Third-generation biorefineries as the means to produce fuels and chemicals from CO2. Nat. Catal. 2020, 3, 274-88.
2. Song, B.; Lin, R.; Lam, C. H.; Wu, H.; Tsui, T.; Yu, Y. Recent advances and challenges of inter-disciplinary biomass valorization by integrating hydrothermal and biological techniques. Renew. Sustain. Energy. Rev. 2021, 135, 110370.
3. Sun, Z.; Zhao, H.; Yu, X.; Hu, J.; Chen, Z. Glucose photorefinery for sustainable hydrogen and value-added chemicals coproduction. Chem. Synth. 2024, 4, 4.
4. Ma, J.; Liu, K.; Yang, X.; et al. Recent advances and challenges in photoreforming of biomass-derived feedstocks into hydrogen, biofuels, or chemicals by using functional carbon nitride photocatalysts. ChemSusChem 2021, 14, 4903-22.
5. Shi, C.; Kang, F.; Zhu, Y.; et al. Photoreforming lignocellulosic biomass for hydrogen production: optimized design of photocatalyst and photocatalytic system. Chem. Eng. J. 2023, 452, 138980.
6. Yu, J.; Dappozze, F.; Martín-gomez, J.; et al. Glyceraldehyde production by photocatalytic oxidation of glycerol on WO3-based materials. Appl. Catal. B. Environ. 2021, 299, 120616.
7. Goh, B. H. H.; Chong, C. T.; Ge, Y.; et al. Progress in utilisation of waste cooking oil for sustainable biodiesel and biojet fuel production. Energy. Convers. Manag. 2020, 223, 113296.
8. Sun, C.; Hu, Y.; Sun, F.; et al. Comparison of biodiesel production using a novel porous Zn/Al/Co complex oxide prepared from different methods: physicochemical properties, reaction kinetic and thermodynamic studies. Renew. Energy. 2022, 181, 1419-30.
9. Stelmachowski, M.; Marchwicka, M.; Grabowska, E.; Diak, M. The photocatalytic conversion of (biodiesel derived) glycerol to hydrogen - a short review and preliminary experimental results part 1: a review. J. Adv. Oxid. Technol. 2014, 17, 167-78.
10. Ciriminna, R.; Pina, C. D.; Rossi, M.; Pagliaro, M. Understanding the glycerol market. Eur. J. Lipid. Sci. Technol. 2014, 116, 1432-9.
11. Estahbanati MR, Feilizadeh M, Attar F, Iliuta MC. Current developments and future trends in photocatalytic glycerol valorization: process analysis. React. Chem. Eng. 2021, 6, 197-219.
12. Luo, L.; Chen, W.; Xu, S. M.; et al. Selective photoelectrocatalytic glycerol oxidation to dihydroxyacetone via enhanced middle hydroxyl adsorption over a Bi2O3-incorporated catalyst. J. Am. Chem. Soc. 2022, 144, 7720-30.
13. Liu, D.; Liu, J. C.; Cai, W.; et al. Selective photoelectrochemical oxidation of glycerol to high value-added dihydroxyacetone. Nat. Commun. 2019, 10, 1779.
14. Saidi, M.; Moradi, P. Conversion of biodiesel synthesis waste to hydrogen in membrane reactor: theoretical study of glycerol steam reforming. Int. J. Hydrogen. Energy. 2020, 45, 8715-26.
15. Bivona, L. A.; Vivian, A.; Fusaro, L.; Fiorilli, S.; Aprile, C. Design and catalytic applications of 1D tubular nanostructures: improving efficiency in glycerol conversion. Appl. Catal. B. Environ. 2019, 247, 182-90.
16. Morales, D. M.; Jambrec, D.; Kazakova, M. A.; et al. Electrocatalytic conversion of glycerol to oxalate on Ni oxide nanoparticles-modified oxidized multiwalled carbon nanotubes. ACS. Catal. 2022, 12, 982-92.
17. Chen, J.; Yan, S.; Zhang, X.; Tyagi, R. D.; Surampalli, R. Y.; Valéro, J. R. Chemical and biological conversion of crude glycerol derived from waste cooking oil to biodiesel. Waste. Manag. 2018, 71, 164-75.
18. Tran, N. H.; Kannangara, G. S. Conversion of glycerol to hydrogen rich gas. Chem. Soc. Rev. 2013, 42, 9454-79.
19. Lakshmana Reddy, N.; Cheralathan, K. K.; Durga Kumari, V.; Neppolian, B.; Muthukonda Venkatakrishnan, S. Photocatalytic reforming of biomass derived crude glycerol in water: a sustainable approach for improved hydrogen generation using Ni(OH)2 decorated TiO2 nanotubes under solar light irradiation. ACS. Sustain. Chem. Eng. 2018, 6, 3754-64.
20. Zhao, S.; Dai, Z.; Guo, W.; Chen, F.; Liu, Y.; Chen, R. Highly selective oxidation of glycerol over Bi/Bi3.64Mo0.36O6.55 heterostructure: dual reaction pathways induced by photogenerated 1O2 and holes. Appl. Catal. B. Environ. 2019, 244, 206-14.
21. Shen, Y.; Mamakhel, A.; Liu, X.; et al. Promotion mechanisms of Au supported on TiO2 in thermal- and photocatalytic glycerol conversion. J. Phys. Chem. C. 2019, 123, 19734-41.
22. Zhang, Z.; Wang, M.; Zhou, H.; Wang, F. Surface sulfate ion on CdS catalyst enhances syngas generation from biopolyols. J. Am. Chem. Soc. 2021, 143, 6533-41.
23. Chang, J.; Song, F.; Hou, Y.; et al. Molybdenum, tungsten doped cobalt phosphides as efficient catalysts for coproduction of hydrogen and formate by glycerol electrolysis. J. Colloid. Interface. Sci. 2024, 665, 152-62.
24. Estahbanati, M. K.; Feilizadeh, M.; Iliuta, M. C. Photocatalytic valorization of glycerol to hydrogen: optimization of operating parameters by artificial neural network. Appl. Catal. B. Environ. 2017, 209, 483-92.
25. Guo, L.; Sun, Q.; Marcus, K.; et al. Photocatalytic glycerol oxidation on AuxCu–CuS@TiO2 plasmonic heterostructures. J. Mater. Chem. A. 2018, 6, 22005-12.
26. Xiao, Y.; Wang, M.; Liu, D.; et al. Selective photoelectrochemical oxidation of glycerol to glyceric acid on (002) facets exposed WO3 nanosheets. Angew. Chem. Int. Ed. Engl. 2024, 63, e202319685.
27. Yu, X.; Yu, Z.; Zhao, H.; Gates, I. D.; Hu, J. Photothermal catalytic H2 production over hierarchical porous CaTiO3 with plasmonic gold nanoparticles. Chem. Synth. 2023, 3, 3.
28. Ouyang, J.; Liu, X.; Wang, B. H.; et al. WO3 photoanode with predominant exposure of {202} facets for enhanced selective oxidation of glycerol to glyceraldehyde. ACS. Appl. Mater. Interfaces. , 2022, 23536-45.
29. Lu, Y.; Lee, B. G.; Lin, C.; et al. Solar-driven highly selective conversion of glycerol to dihydroxyacetone using surface atom engineered BiVO4 photoanodes. Nat. Commun. 2024, 15, 5475.
30. Yu, J.; González-Cobos, J.; Dappozze, F.; et al. WO3-based materials for photoelectrocatalytic glycerol upgrading into glyceraldehyde: unravelling the synergistic photo- and electro-catalytic effects. Appl. Catal. B. Environ. 2022, 318, 121843.
31. Kim, D.; Oh, L. S.; Tan, Y. C.; Song, H.; Kim, H. J.; Oh, J. Enhancing glycerol conversion and selectivity toward glycolic acid via precise nanostructuring of electrocatalysts. ACS. Catal. 2021, 11, 14926-31.
32. Carrettin, S.; McMorn, P.; Johnston, P.; Griffin, K.; Hutchings, G. J. Selective oxidation of glycerol to glyceric acid using a gold catalyst in aqueous sodium hydroxide. Chem. Commun. 2002, 696-7.
33. Zhang, X.; Zhou, D.; Wang, X.; et al. Overcoming the deactivation of Pt/CNT by introducing CeO2 for selective base-free glycerol-to-glyceric acid oxidation. ACS. Catal. 2020, 10, 3832-7.
34. Yan, H.; Yao, S.; Zhao, S.; et al. Insight into the basic strength-dependent catalytic performance in aqueous phase oxidation of glycerol to glyceric acid. Chem. Eng. Sci. 2021, 230, 116191.
35. Sanwald, K. E.; Berto, T. F.; Jentys, A.; Camaioni, D. M.; Gutiérrez, O. Y.; Lercher, J. A. Kinetic coupling of water splitting and photoreforming on SrTiO3-based photocatalysts. ACS. Catal. 2018, 8, 2902-13.
36. Li, Y.; Zhang, D.; Qiao, W.; et al. Nanostructured heterogeneous photocatalyst materials for green synthesis of valuable chemicals. Chem. Synth. 2022, 2, 9.
37. Lin, J.; Wu, X.; Xie, S.; et al. Visible-light-driven cleavage of C-O linkage for lignin valorization to functionalized aromatics. ChemSusChem 2019, 12, 5023-31.
38. Holmes, M. A.; Townsend, T. K.; Osterloh, F. E. Quantum confinement controlled photocatalytic water splitting by suspended CdSe nanocrystals. Chem. Commun. 2012, 48, 371-3.
39. Asahi, R.; Morikawa, T.; Irie, H.; Ohwaki, T. Nitrogen-doped titanium dioxide as visible-light-sensitive photocatalyst: designs, developments, and prospects. Chem. Rev. 2014, 114, 9824-52.
40. Choi, W.; Termin, A.; Hoffmann, M. R. The role of metal ion dopants in quantum-sized TiO2: correlation between photoreactivity and charge carrier recombination dynamics. J. Phys. Chem. 1994, 98, 13669-79.
41. Li, J.; Yang, W.; Wu, A.; Zhang, X.; Xu, T.; Liu, B. Band-gap tunable 2D hexagonal (GaN)1-x(ZnO)x solid-solution nanosheets for photocatalytic water splitting. ACS. Appl. Mater. Interfaces. 2020, 12, 8583-91.
42. Tsuji, I.; Kato, H.; Kobayashi, H.; Kudo, A. Photocatalytic H2 evolution reaction from aqueous solutions over band structure-controlled (AgIn)xZn2(1-x)S2 solid solution photocatalysts with visible-light response and their surface nanostructures. J. Am. Chem. Soc. 2004, 126, 13406-13.
43. Chen, J.; Chen, J.; Li, Y. Hollow ZnCdS dodecahedral cages for highly efficient visible-light-driven hydrogen generation. J. Mater. Chem. A. 2017, 5, 24116-25.
44. Song, J.; Zhao, H.; Sun, R.; Li, X.; Sun, D. An efficient hydrogen evolution catalyst composed of palladium phosphorous sulphide (PdP~0.33S~1.67) and twin nanocrystal Zn0.5Cd0.5S solid solution with both homo- and hetero-junctions. Energy. Environ. Sci. 2017, 10, 225-35.
45. Yu, G.; Qian, J.; Zhang, P.; et al. Collective excitation of plasmon-coupled Au-nanochain boosts photocatalytic hydrogen evolution of semiconductor. Nat. Commun. 2019, 10, 4912.
46. Zhao, H.; Li, C. F.; Yong, X.; et al. Coproduction of hydrogen and lactic acid from glucose photocatalysis on band-engineered Zn1-xCdxS homojunction. iScience 2021, 24, 102109.
47. Liu, M.; Jing, D.; Zhou, Z.; Guo, L. Twin-induced one-dimensional homojunctions yield high quantum efficiency for solar hydrogen generation. Nat. Commun. 2013, 4, 2278.
48. Gao, R.; Cheng, B.; Fan, J.; Yu, J.; Ho, W. ZnxCd1-xS quantum dot with enhanced photocatalytic H2-production performance. Chinese. J. Catal. 2021, 42, 15-24.
49. Copeland, J. R.; Santillan, I. A.; Schimming, S. M.; Ewbank, J. L.; Sievers, C. Surface interactions of glycerol with acidic and basic metal oxides. J. Phys. Chem. C. 2013, 117, 21413-25.
50. An, Z.; Zhang, Z.; Huang, Z.; et al. Pt1 enhanced C-H activation synergistic with Ptn catalysis for glycerol cascade oxidation to glyceric acid. Nat. Commun. 2022, 13, 5467.
Cite This Article

How to Cite
Download Citation
Export Citation File:
Type of Import
Tips on Downloading Citation
Citation Manager File Format
Type of Import
Direct Import: When the Direct Import option is selected (the default state), a dialogue box will give you the option to Save or Open the downloaded citation data. Choosing Open will either launch your citation manager or give you a choice of applications with which to use the metadata. The Save option saves the file locally for later use.
Indirect Import: When the Indirect Import option is selected, the metadata is displayed and may be copied and pasted as needed.
About This Article
Copyright
Data & Comments
Data
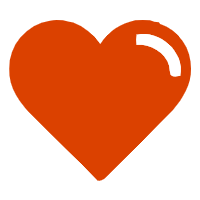
Comments
Comments must be written in English. Spam, offensive content, impersonation, and private information will not be permitted. If any comment is reported and identified as inappropriate content by OAE staff, the comment will be removed without notice. If you have any queries or need any help, please contact us at [email protected].