Macrocycle-based covalent-organic-polymer as efficient oxygen electrocatalysts for zinc-air flow batteries
Abstract
Covalent organic polymers (COPs), as emerging porous materials with well-defined architectures and high hydrothermal stability, have attracted extensive attention in the field of electrocatalysis. Herein, we report a rational design method for preparing oxygen reduction reaction electrocatalysts with the assistance of a predesigned macrocyclic COP model molecular. With the predesigned nitrogen position and structural features in macrocyclic chain-like COP-based materials, the obtained COPMCT-Co-900 catalyst provided excellent oxygen reduction performance, where the half-wave potential (E1/2) reaches 0.85 V (vs. RHE), comparable to commercial Pt/C. We also extended the strategy to similar macrocycle COPs and Fe-based and Ni-based metal sources and studied the oxygen reduction reaction performance of corresponding catalysts, proving the universality of the method. Interestingly, we assemble COPMCT-Co-900 catalyst as air electrode catalyst of the self-made rechargeable zinc-air flow batteries, which exhibit outstanding power density (155.6 mW·cm-2) and long cycle life (90 h, 270 cycles at 10 mA·cm-2). Our studies provide a new method for the development of high-performance oxygen electrodes applied in zinc-air flow battery devices.
Keywords
INTRODUCTION
Over the last decades, efficient design and research of non-precious metal catalysts (NPMC) have made significant progress in oxygen reduction reaction (ORR) electrocatalytic activity and stability[1-6]. The current NPMC systems mainly include transition metal (TM) nitrogen-doped carbon[7-13], supported TM oxides[14,15], TM carbides[16-18], heteroatom-doped carbon materials, etc.[19-21]. Among them, macrocyclic compounds have received widespread attention due to their unique 2D topology structure, high conjugation, and redox-rich chemical properties, exhibiting great potential to replace platinum-based catalysts[22-24]. Macrocyclic compounds are composed of various ligands, such as tetraazamacrocyclic porphyrins and phthalocyanines, and corrode with four nitrogen atoms, which form coordination sites with stabilized TM-N4 compounds, enhancing their ORR catalytic activity. One of the most attractive features for macrocyclic compounds is that their electronic and catalytic properties can be improved by rationally designing and adjusting their ligand characters. Metal macrocycles, such as porphyrins and phthalocyanines doped with TMs (Fe, Co or Ni), are one of the most widely used precursors for preparing ORR electrocatalysts. In 1964, Jasinski first observed that metal macrocycles could promote the ORR of fuel cells and conducted in-depth research on them as potential cathode catalysts in metal-air flow batteries [zinc-air flow batteries (ZAFBs)] and fuel cells[25]; the researchers found that metal atom occupies the center of the macrocycle cavity with maximum stability, which radically facilitates the catalytic activity of ORR electrocatalysts[26-31]. In addition, high ORR catalytic activity was found existing in macrocyclic containing TM-N4 centers (especially CoN4 or FeN4 moiety), such as porphyrins compounds (tetramethoxyphenyl-porphyrin, tetraphenyl-porphyrin, phthalocyanine compounds, and tetraazanthracene)[32,33]. Therefore, metal macrocycle-based catalysts have been extensively studied from synthetic routes to electrocatalytic mechanisms, especially for Fe-based or Co-based porphyrins[34]. However, relatively poor intrinsic conductivity of metallomacrocycles affects the electron transport during electrocatalysis and leads to their behindhand electrocatalytic performance.
The high-temperature pyrolysis for preparing carbon-based catalysts is crucial to improving the ORR catalytic activity of metal macrocyclic systems. The preparation of TM-doped nitrogen-carbon-based catalysts through carbonization of metallophthalocyanine/porphyrin macrocycles has been extensively studied in the field of electrocatalytic materials, and significant progress has been made in terms of oxygen reduction reactivity and lifetime[35]. For example, Choi et al. realized the direct construction of an ordered mesoporous three-dimensional iron-porphyrin catalyst by pyrolyzing the FeN4 complex (tetrapyridyl porphyrin iron) and SBA-15[36]. Compared with Fe-N-C catalysts prepared by carbonizing various precursors on carbon supports, porphyrin-like iron materials completely avoid the addition of additional carbon supports, and the Fe-Nx active sites are uniformly distributed in mesoporous graphite matrix with high specific surface areas. These structures effectively increase the density of effective catalytic sites and promote the transport property of oxygen in macropores and mesopores, accelerating the kinetic of ORR. These characteristics make them an excellent ORR catalytic material.
Recently, a series of new porous materials with covalently linked periodic frameworks and conjugated structures, i.e., macrocycle-based covalent organic polymers (COPs), have been developed[37-40]. They are equipped with superior tailorability in functional design and structure regulation[41-43], so that after pyrolysis, the riveting positions of doped heteroatoms and metal sites in COP precursors can be well predesigned[44]. For example, in recent studies, our group used the Yamamoto coupling reaction to controllably synthesize two-dimensional macrocycle-based COPs with quasi-phthalocyanine structures coordinating with Ni, Fe and Co metal ions and prepared non-noble metal-doped ORR catalysts by high-temperature pyrolysis towards COP precursors[45,46]. Due to the inherent rigid structural characteristics and flexible, adjustable structural design similar to the covalent organic framework (COF)[29], the predesigned catalytic site structure can be maintained to some extent during the high-temperature pyrolysis process, which guarantees the ideal ORR catalytic activity compared with the direct calcination towards carbon, nitrogen and metal-containing composites. Under alkaline conditions, the ORR catalytic activity of COP-based NPMCs achieved great performance improvement. Compared with Pt/C, the Co-doped catalyst showed a higher limiting current density, and its kinetic current is 1.4 times that of the Pt/C catalyst.
Inspired by the above studies, here, we utilized the molecular model of macrocyclic COP to design and prepare nitrogen-coordinated precursor structures and obtained COPMCT-Co-900 electrocatalysts with excellent oxygen reduction activity through subsequent high-temperature carbonization. We extended this strategy to two other COPs with different macrocycle structures and studied the effects of precursors, metal sources and carbonization temperature on the oxygen reduction performance of catalysts. The results show that the COPMCT-Co-900 catalyst exhibits the best oxygen reduction performance, and the half-wave potential (E1/2) reached 0.85 V [vs. reversible hydrogen electrode (RHE)] under alkaline test conditions, which is comparable to commercial Pt/C. After 50,000 s of chronoperometric testing, the current density of COPMCT-Co-900 decreased by 3.2%
EXPERIMENTAL SECTION
Chemicals
Ferric nitrate hexahydrate (99%) (Alfa Aesar), Cobal nitrate hexahydrate (99+%) (ACROS Organics), Nickel nitrate hexahydrate (Alfa Aesar), benzene-1,2,4,5-tetracarbonitrile (BTC) (99%) (Bide Medical),
Preparation of COPMCT-M
DTZ was employed as monomers to polymerize with BTC and generated the corresponding products, denoted as COPMCT-Co, respectively. Typically, BTC and corresponding monomers were added to 60 mL ethylene glycol under the molar ratio of 1:2.4, and then ultrasound for 5 min to obtain solution A. Additionally, 1.2 equivalent amounts of metal salt, such as iron, cobalt, and nickel salts, were added to
Preparation of COPMCT-M-900
COPMCT-M is ground into a powder, carbonized in a tube furnace for 2 h (900 °C), and naturally cooled to obtain COPMCT-M-900.
Physical characterization
The microstructure of the samples was obtained by scanning electron microscope (TESCAN, MAIA 3 XMU) and transmission electron microscope (Hitachi, HT7700). X-ray diffractometer (XRD) data were measured by XRD-7000. Sample compositions were measured by X-ray photoelectron spectroscopy (XPS) (Thermo Fischer, ESCALAB). The Fourier transform infrared spectroscopy (FT-IR) analysis was performed on a Nicolet 8700/Continuum XL. The valence structure of the sample was measured by an X-ray photoelectron spectrometer (THERMO VG, ESCALAB 250). The molecular structure information was obtained by a Raman spectrometer (HORIBA JOBIN YVON SAS, LabRAM HR Evolution), and the specific surface area and pore distribution were determined by BET-ASAP2460.
Electrochemical measurements
We used the CHI electrochemical instrument to perform electrochemical tests on the samples through a three-electrode system, in which the working electrode was coated with a glassy carbon electrode of the sample to be tested. Saturated calomel and graphite rod were used as the reference and counter electrodes, respectively. Furthermore, 5 mg catalyst is evenly dispersed in the mixed solution of ethanol (950 μL) and 5% Nafion (50 μL) by ultrasound, and the ink is coated on the surface of the glass carbon electrode. The working electrode with a load of 0.76 mg·cm-2 was obtained by natural drying (the load of Pt/C was
Kouteckye-Levich (K-L) Equations (3)-(5):
Where Ir, Id, J, N, Jk, B, JL, ω, n, F, C0, ν, and D0 represent respectively the ring current, the disk current, the measured current density, the ring collection efficiency, the angular velocity of the electrode (rpm), the reciprocal slope of equation, the transferred electron number, the kinematic viscosity of the electrolyte, the kinetic and diffusion-limiting current density, the Faraday constant, the bulk concentration of O2,
where ν: scan rate, CGC: double layer capacitance, j: measured current density.
Recharge ZAFBs assembly
The test steps of the rechargeable ZAFBs are as follows: (1) The electrolyte is configured. In addition, 6 M KOH and 0.2 M ZnO are dissolved in deionized water; (2) The air cathode is prepared and the sample is configured into a uniform ink and sprayed on carbon paper with a gas diffusion layer, and the sample load is 2mg·cm-2; (3) Anode preparation, polishing of 0.3 mm thick zinc sheet; (4) The above materials are assembled into ZAFBs, and the electrolyte flow rate is set at 5mL·min-1.
RESULTS AND DISCUSSION
As shown in Scheme 1, we utilize BTC and DTZ with high symmetric functionalities as reaction monomers to polycondense into chain hemiporphyrocyanogen COP rich in CoN4 active centers, denoted as
Scheme 1. Schematic diagram of the synthesis of COPMCT-Co-900 catalyst. COP: Covalent organic polymer.
The structural information of the COPMCT-Co precursor was confirmed by solid-state 13C nuclear magnetic resonance spectroscopy (NMR) [Figure 1A]. The characteristic peak at (a) 160.73 ppm is attributed to the carbon moiety on the five-membered ring connecting the nitrogen atom in the benzpyrole. The characteristic peak at (b) 153.7 ppm is ascribed to the carbon-containing moiety in the DTZ monomer; the characteristic peaks at (c) 140.74, (d) 126.0, (e) 115.47 ppm were attributed to the carbon atom of the benzene ring in the indole ring, respectively. We conducted high-resolution transmission electron microscopy (HRTEM) tests on the catalyst to further determine its composition and structure [Figure 1B and C]. The obvious cobalt nanoparticles were observed in Figure 1B. After further local magnification, we find that obvious lattice fringes with a lattice spacing of 0.20 nm [corresponding to the (111) crystal plane of cobalt nanoparticles] and 0.34 nm [corresponding to the (002) crystal plane of carbon matrix], respectively, indicating that Co-N-C catalyst loaded with Co nanoparticles has been successfully synthesized. Then, the elemental composition and chemical states of the COPMCT-Co-900 were tested by XPS [Figure 1D-F and Supplementary Figure 1]. COPMCT-Co is mainly composed of Co, N, O and C elements, of which C occupies the highest content, reaching 67.85%, followed by N, O and Co elements, accounting for 16.47%, 14.7% and 0.98%, respectively. The high-resolution Co 2p XPS spectra of COPMCT-Co-900 are deconvoluted into
Figure 1. (A) NMR spectra of COPMCT-Co; (B and C) HRTEM images of COPMCT-Co-900 at different scales; (D) High-resolution Co 2p XPS for COPMCT-Co-900; (E) High-resolution N 1s XPS for COPMCT-Co-900; (F) High-resolution C 1s XPS for COPMCT-Co-900. NMR: Nuclear magnetic resonance spectroscopy; COP: covalent organic polymer; HRTEM: high-resolution transmission electron microscopy; XPS: X-ray photoelectron spectroscopy.
We performed FT-IR tests on BTC and our as-synthesized COPMCT-Co polymer [Figure 2A]. The monomer BTC exhibits a strong characteristic peak at 2,245 cm-1, corresponding to the stretching vibration peak of
Figure 2. (A) FT-IR spectra of BTC and COPMCT-Co; (B) The corresponding pore distribution of COPMCT-Co-900; (C) N2 adsorption and desorption isotherm of COPMCT-Co-900; (D) Raman spectra of COPMCT-Co-900; XRD spectra of (E) COPMCT-Co and (F)
We conducted electrochemical tests on COPMCT-Co at different carbonized temperatures to explore the effect of carbonization temperature on catalyst performance. Figure 3A shows the oxygen reduction polarization curves of catalysts carbonized at 800-1,000 °C. The results show that the oxygen reduction performance of the catalyst obtained by carbonization at 900 °C is optimal. The COPMCT-Co-900 has the best electrochemical performance, with an initial potential of 0.97 V, a limited current density of 6.1 mA·cm-2, and a E1/2 of 0.85 V. Figure 3B shows the E1/2 of the catalyst at these five carbonization temperatures. In the range of 800~1000 °C, the E1/2 of the catalyst first increases and then decreases with rising temperature, indicating 900 °C as the optimal carbonization temperature. Having fast oxygen reduction kinetics is very important for the catalyst performance index. As shown in Figure 3C, the oxygen reduction polarization curves of catalysts carbonized at various carbonization temperatures were converted into the Tafel slope. The COPMCT-Co-900 delivers a Tafel slope of 79.5 mV·dec-1. Therefore, COPMCT-Co-900 maintains the best oxygen reduction kinetics, and 900 °C is the optimal temperature for catalyst carbonization. We first performed cyclic voltammetry (CV) tests on the catalyst in an alkaline electrolyte (0.1 M KOH aqueous solution) [Figure 3D]. An obvious oxygen reduction peak for the catalyst appears at ~0.8 V (vs. RHE). By contrast, we tested its CV test in nitrogen-saturated 0.1 mol·L-1 KOH solution, and no peak appears in the range of 0-1.2 V (vs. RHE), indicating the superior ORR catalytic activity of COPMCT-Co-900. In addition, we explored the effects of diverse doping metals on oxygen reduction activity and synthesized
Figure 3. (A) The LSV curves of COPMCT-Co-X (X represent pyrolysis temperature) catalysts at different temperatures; (B) E1/2 of COPMCT-Co-X catalysts at different temperatures; (C) Tafel plots of COPMCT-Co-X; (D) The CV curves of COPMCT-Co-900; (E) The LSV curves of COPMCT-Co-900, COPMCT-Ni-900, COPMCT- 900 and COPMCT-Fe-900; (F) Tafel plots of COPMCT-Co-900, COPMCT-Ni-900, COPMCT- 900 and COPMCT-Fe-900. LSV: Linear sweep voltammetry; COP: covalent organic polymer; CV: cyclic voltammetry.
In order to prove the universality and versatility of the method, we have successfully prepared two other Co-based chain COP precursors using DPD and TDZ monomers, denoted as COPMCT-Co, COPMCP-Co and COPMCZ-Co [Supplementary Figure 2]. Linear sweep voltammetry (LSV) curve results show that the ORR catalytic performance of COPMCT-Co is the highest compared with other catalysts. Through quantitative calculation analysis of the precursor, we found that the electrostatic potential of COPMCT-Co is between COPMCZ-Co and COPMCZ-Co, and the adsorption strength of oxygen intermediates is moderate, which is conducive to both the oxygen adsorption process and the oxygen desorption process. Therefore,
Figure 4. (A) ORR LSV curves of COPMCT-Co-900, COPMCP-Co-900 and COPMCZ-Co-900; (B) LSV of COPMCT-Co-900 at different rotating rates; (C) The corresponding K-L plots at various potentials, respectively; (D) I-t curves performed of COPMCT-Co-900 and Pt/C; (E) Hydrogen peroxide yield of COPMCT-Co, COPMCT-Co-900 and Pt/C; (F) Electron transfer numbers of COPMCT-Co,
Furthermore, we evaluated the performance of COPMCT-Co-900 in energy conversion devices ZAFBs, fabricated COPMCT-Co-900 as an air cathode catalyst of ZAFBs, and investigated its performance in ZAFBs [Figure 5A]. When COPMCT-Co-900 was used as the cathode catalyst for ZAFBs, the power density of
Figure 5. (A) A schematic configuration of the homemade Zn-air battery; (B) Comparison of polarization and power density curves using Pt/C and COPMCT-Co-900 as catalysts; (C) Open circuit plot of the Zn-air battery using COPMCT-Co-900 and Pt/C as catalysts; (D) Cycle discharge curves of COPMCT-Co-900-driven Zn-air batteries at periodically changed current density; (E) Discharge/charge cycling performance of Zn-air batteries with COPMCT-Co-900 as catalyst at a current density of 10 mA·cm-2. COP: Covalent organic polymer.
CONCLUSION
In conclusion, we demonstrate a rational design method for preparing ORR electrocatalysts with the assistance of a predesigned macrocyclic COP model molecular. Three kinds of metal macrocyclic COP materials with metal-N4 structures were prepared by a simple microwave-assisted method. The
DECLARATIONS
Authors’ contributions
Performed the synthesis, structural characterizations and electrochemical tests: Li X
Made substantial contributions to the conception and design of the study, performed data analysis and interpretation, and wrote the original draft: Leng Y
Supervised and led this project and revised the manuscript repeatedly: Xiang Z, Li X
Performed partial electrochemical tests and ZAFB device assembly: Chen T, Yin Y, Li J
All authors provided critical feedback and helped shape the research and paper. All authors commented on the paper.
Availability of data and materials
Supporting Information is available from the corresponding author upon reasonable request.
Financial support and sponsorship
This work was supported by the National Key Research and Development Program of China (2022YFB3807500); the NSF of China (22220102003); the Beijing Natural Science Foundation (JL23003); “Double-First-Class” construction projects (XK180301, XK1804-02); China Postdoctoral Science Foundation (2023TQ0020) and Postdoctoral Fellowship Program of CPSF (GZC20230199).
Conflicts of interest
All authors declared that there are no conflicts of interest.
Ethical approval and consent to participate
Not applicable.
Consent for publication
Not applicable.
Copyright
© The Author(s) 2024.
Supplementary Materials
REFERENCES
1. King LA, Hubert MA, Capuano C, et al. A non-precious metal hydrogen catalyst in a commercial polymer electrolyte membrane electrolyser. Nat Nanotechnol 2019;14:1071-4.
2. Varnell JA, Tse EC, Schulz CE, et al. Identification of carbon-encapsulated iron nanoparticles as active species in non-precious metal oxygen reduction catalysts. Nat Commun 2016;7:12582.
3. Malko D, Kucernak A, Lopes T. In situ electrochemical quantification of active sites in Fe-N/C non-precious metal catalysts. Nat Commun 2016;7:13285.
4. Bashyam R, Zelenay P. A class of non-precious metal composite catalysts for fuel cells. Nature 2006;443:63-6.
5. Leng Y, Yang B, Zhao Y, Xiang Z. Fluorinated bimetallic nanoparticles decorated carbon nanofibers as highly active and durable oxygen electrocatalyst for fuel cells. J Energy Chem 2022;73:549-55.
6. Li X, Liu Q, Yang B, Liao Z, Yan W, Xiang Z. An initial covalent organic polymer with closed-F edges directly for proton-exchange-membrane fuel cells. Adv Mater 2022;34:e2204570.
7. Bates JS, Johnson MR, Khamespanah F, Root TW, Stahl SS. Heterogeneous M-N-C catalysts for aerobic oxidation reactions: lessons from oxygen reduction electrocatalysts. Chem Rev 2023;123:6233-56.
8. Liu J, Wan X, Liu S, et al. Hydrogen passivation of M-N-C (M = Fe, Co) catalysts for storage stability and ORR activity improvements. Adv Mater 2021;33:2170300.
9. Patniboon T, Hansen HA. Acid-stable and active M-N-C catalysts for the oxygen reduction reaction: the role of local structure. ACS Catal 2021;11:13102-18.
10. Shi Q, He Y, Bai X, et al. Methanol tolerance of atomically dispersed single metal site catalysts: mechanistic understanding and high-performance direct methanol fuel cells. Energy Environ Sci 2020;13:3544-55.
11. Sun K, Dong J, Sun H, et al. Co(CN)3 catalysts with well-defined coordination structure for the oxygen reduction reaction. Nat Catal 2023;6:1164-73.
12. Sun Y, Silvioli L, Sahraie NR, et al. Activity-selectivity trends in the electrochemical production of hydrogen peroxide over single-site metal-nitrogen-carbon catalysts. J Am Chem Soc 2019;141:12372-81.
13. Zhao CX, Li BQ, Liu JN, Zhang Q. Intrinsic electrocatalytic activity regulation of M-N-C single-atom catalysts for the oxygen reduction reaction. Angew Chem Int Ed Engl 2021;60:4448-63.
14. Singh SK, Kashyap V, Manna N, et al. Efficient and durable oxygen reduction electrocatalyst based on CoMn alloy oxide nanoparticles supported over N-doped porous graphene. ACS Catal 2017;7:6700-10.
15. Chen Z, Higgins D, Yu A, Zhang L, Zhang J. A review on non-precious metal electrocatalysts for PEM fuel cells. Energy Environ Sci 2011;4:3167-92.
16. Ratso S, Kruusenberg I, Käärik M, et al. Highly efficient transition metal and nitrogen co-doped carbide-derived carbon electrocatalysts for anion exchange membrane fuel cells. J Power Sources 2018;375:233-43.
17. Yu Y, Zhou J, Sun Z. Novel 2D transition-metal carbides: ultrahigh performance electrocatalysts for overall water splitting and oxygen reduction. Adv Funct Mater 2020;30:2000570.
18. Das TK, Jesionek M, Çelik Y, Poater A. Catalytic polymer nanocomposites for environmental remediation of wastewater. Sci Total Environ 2023;901:165772.
19. Feng X, Bai Y, Liu M, et al. Untangling the respective effects of heteroatom-doped carbon materials in batteries, supercapacitors and the ORR to design high performance materials. Energy Environ Sci 2021;14:2036-89.
20. Cheon JY, Kim JH, Kim JH, Goddeti KC, Park JY, Joo SH. Intrinsic relationship between enhanced oxygen reduction reaction activity and nanoscale work function of doped carbons. J Am Chem Soc 2014;136:8875-8.
21. Liu M, Sun T, Peng T, et al. Fe-NC single-atom catalyst with hierarchical porous structure and P−O bond coordination for oxygen reduction. ACS Energy Lett 2023;8:4531-9.
22. Wang Y, Li K, Cheng R, et al. Enhanced electronic interaction between iron phthalocyanine and cobalt single atoms promoting oxygen reduction in alkaline and neutral aluminum-air batteries. Chem Eng J 2022;450:138213.
23. Madhavachary R, Abdelraheem EMM, Rossetti A, et al. Two-step synthesis of complex artificial macrocyclic compounds. Angew Chem Int Ed Engl 2017;56:10725-9.
24. Luo Y, Chen Y, Xue Y, et al. Electronic structure regulation of iron phthalocyanine induced by anchoring on heteroatom-doping carbon sphere for efficient oxygen reduction reaction and Al-Air battery. Small 2022;18:e2105594.
26. Shao M, Chang Q, Dodelet JP, Chenitz R. Recent advances in electrocatalysts for oxygen reduction reaction. Chem Rev 2016;116:3594-657.
27. Luo M, Zhao Z, Zhang Y, et al. PdMo bimetallene for oxygen reduction catalysis. Nature 2019;574:81-5.
28. Wang HF, Chen L, Pang H, Kaskel S, Xu Q. MOF-derived electrocatalysts for oxygen reduction, oxygen evolution and hydrogen evolution reactions. Chem Soc Rev 2020;49:1414-48.
29. Li X, Xiang Z. Identifying the impact of the covalent-bonded carbon matrix to FeN4 sites for acidic oxygen reduction. Nat Commun 2022;13:57.
30. Li X, Liu D, Liu Q, Xiang Z. A pyrolysis-free method toward large-scale synthesis of ultra-highly efficient bifunctional oxygen electrocatalyst for zinc-air flow batteries. Small 2022;18:e2201197.
31. Li X, Liu Y, Xiang Z. Dithiine bridged phthalocyanine-based covalent organic frameworks for highly efficient oxygen reduction reaction. J Phys Chem C 2022;126:4008-14.
32. Yang S, Yu Y, Gao X, Zhang Z, Wang F. Recent advances in electrocatalysis with phthalocyanines. Chem Soc Rev 2021;50:12985-3011.
33. Li X, Chen T, Yang B, Xiang Z. Fundamental understanding of electronic structure in FeN4 site on electrocatalytic activity via dz2-orbital-driven charge tuning for acidic oxygen reduction. Angew Chem Int Ed Engl 2023;62:e202215441.
34. Liang Z, Guo H, Zhou G, et al. Metal-organic-framework-supported molecular electrocatalysis for the oxygen reduction reaction. Angew Chem Int Ed Engl 2021;60:8472-6.
35. Wang X, Wang B, Zhong J, et al. Iron polyphthalocyanine sheathed multiwalled carbon nanotubes: a high-performance electrocatalyst for oxygen reduction reaction. Nano Res 2016;9:1497-506.
36. Choi J, Kim J, Wagner P, et al. Highly ordered mesoporous carbon/iron porphyrin nanoreactor for the electrochemical reduction of CO2. J Mater Chem A 2020;8:14966-74.
37. Côté AP, Benin AI, Ockwig NW, O’Keeffe M, Matzger AJ, Yaghi OM. Porous, crystalline, covalent organic frameworks. Science 2005;310:1166-70.
38. Liu Y, Diercks CS, Ma Y, et al. 3D covalent organic frameworks of interlocking 1D square ribbons. J Am Chem Soc 2019;141:677-83.
39. Lyle SJ, Osborn Popp TM, Waller PJ, Pei X, Reimer JA, Yaghi OM. Multistep solid-state organic synthesis of carbamate-linked covalent organic frameworks. J Am Chem Soc 2019;141:11253-8.
40. Lyu H, Diercks CS, Zhu C, Yaghi OM. Porous crystalline olefin-linked covalent organic frameworks. J Am Chem Soc 2019;141:6848-52.
41. Diercks CS, Lin S, Kornienko N, et al. Reticular electronic tuning of porphyrin active sites in covalent organic frameworks for electrocatalytic carbon dioxide reduction. J Am Chem Soc 2018;140:1116-22.
42. Nguyen HL, Hanikel N, Lyle SJ, Zhu C, Proserpio DM, Yaghi OM. A porous covalent organic framework with voided square grid topology for atmospheric water harvesting. J Am Chem Soc 2020;142:2218-21.
43. Zhang B, Wei M, Mao H, et al. Crystalline dioxin-linked covalent organic frameworks from irreversible reactions. J Am Chem Soc 2018;140:12715-9.
44. Gropp C, Ma T, Hanikel N, Yaghi OM. Design of higher valency in covalent organic frameworks. Science 2020;370:eabd6406.
45. Yamamoto T, Hayashi Y, Yamamoto A. A novel type of polycondensation utilizing transition metal-catalyzed C-C coupling. I. preparation of thermostable polyphenylene type polymers. Bull Chem Soc Jpn 1978;51:2091-7.
46. Zhou Z, Yamamoto T. Research on carbon - carbon coupling reactions of haloaromatic compounds mediated by zerovalent nickel complexes. Preparation of cyclic oligomers of thiophene and benzene and stable anthrylnickel(II) complexes. J Organomet Chem 1991;414:119-27.
Cite This Article

How to Cite
Download Citation
Export Citation File:
Type of Import
Tips on Downloading Citation
Citation Manager File Format
Type of Import
Direct Import: When the Direct Import option is selected (the default state), a dialogue box will give you the option to Save or Open the downloaded citation data. Choosing Open will either launch your citation manager or give you a choice of applications with which to use the metadata. The Save option saves the file locally for later use.
Indirect Import: When the Indirect Import option is selected, the metadata is displayed and may be copied and pasted as needed.
About This Article
Special Issue
Copyright
Author Biographies
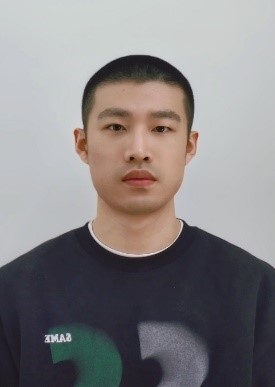
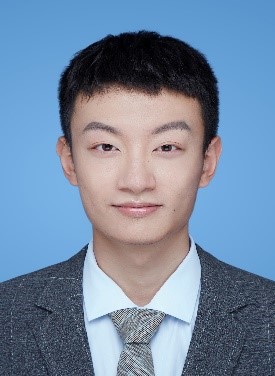
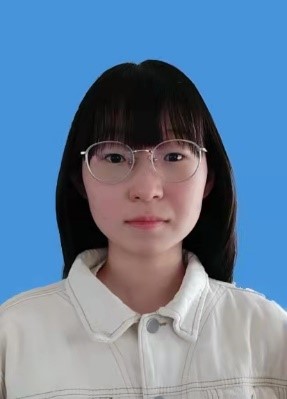
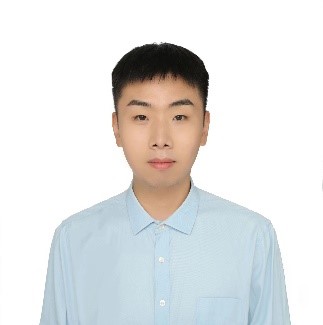
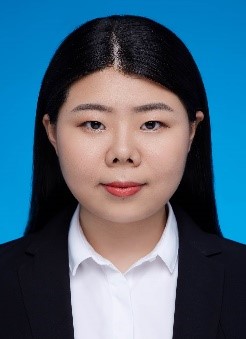
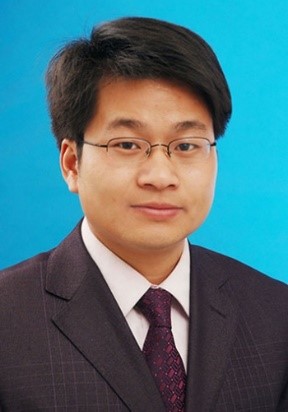
Comments
Comments must be written in English. Spam, offensive content, impersonation, and private information will not be permitted. If any comment is reported and identified as inappropriate content by OAE staff, the comment will be removed without notice. If you have any queries or need any help, please contact us at [email protected].