Zwitterionic hydrogels and their biomedical applications: a review
Abstract
The coexistence of anions and cations in zwitterionic hydrogels results in electrostatic interactions between the polymer chains. This structure endows zwitterionic hydrogels with higher ion sensitivity and promising properties, such as anti-polyelectrolyte and thermosensitive effects. Hydrophilic groups on the molecular backbone give zwitterionic hydrogels good biocompatibility, and they effectively resist the non-specific adsorption of proteins. The abundant functional groups on the molecular skeleton also facilitate the chemical modification of zwitterionic hydrogels. In recent years, these excellent properties have made zwitterionic hydrogels broadly interesting and they have been heavily studied for medical applications. A comprehensive review will help researchers have a deeper understanding of zwitterionic hydrogels and their potential applications. In this review, the types, functional characteristics, and applications in the biomedicine of zwitterionic hydrogels are summarized in detail. In addition, the challenges and opportunities for using zwitterionic hydrogels for biomedical applications are discussed.
Keywords
INTRODUCTION
With the rapid developments of biomedical science, there is an increasing demand for efficient, safe, and sustainable treatment and repair methods. In this context, hydrogels have emerged as a significant material garnering widespread attention[1]. They are high molecular weight polymers with three-dimensional (3D) network structures, which can retain a large amount of water in the swollen state; that is, the 3D network structures swell in water but do not dissolve in a short period[2,3]. According to the charged properties of polymer network structures, hydrogels can be considered ionic or non-ionic. Ionic hydrogels can be further divided into cationic, anionic, and zwitterionic varieties. Zwitterionic polymers include charged zwitterionic groups with uniform arrangements of anions and cations, which make the polymers electrically neutral in the atmosphere[4]. The study of zwitterionic hydrogels, which are crosslinked by zwitterionic polymers, has been popular in recent years due to their good biocompatibility, super-hydrophilicity, resistance to non-specific adsorption of proteins[5], high ion density and the ion-sensitive property[6,7]. Based on these properties, zwitterionic hydrogels are promising medical materials, having a wide range of applications in the biomedical field, including biosensors, flexible wearable devices, tissue engineering, and drug release[8-11].
By combining biosensors with zwitterionic hydrogels, innovative solutions for disease management and personalized medicine can be achieved. As far back as 2008, it was demonstrated that zwitterionic polymers could effectively counteract the non-specific adsorption of proteins[12]. This finding contributed to the design and synthesis of biomimetic antifouling materials, which are important for improving the long-term resistance of implantable biosensors to fibrous capsule formation caused by foreign body reactions. As carriers of biosensor sensing elements, zwitterionic hydrogels can effectively block the penetration of external interfering substances and protect the sensing elements from inactivation or degradation[13], enhancing the sensitivity, long-term stability, and dependability of biosensors. In addition, zwitterionic hydrogels possess excellent adhesiveness and biocompatibility[14], allowing for the firm attachments of biosensors to tissues or organs while maintaining compatibility with the surrounding cells and tissues within the organism[15]. This ensures effective interactions of the biosensors with the targets of analysis. Coupled with the antibacterial properties of zwitterionic hydrogels, the use of zwitterionic hydrogel-modified glucose sensors in diabetic ulcers can achieve the dual purpose of stable monitoring of human blood glucose levels and facilitating wound healing[16]. Furthermore, the excellent mechanical properties and electrical conductivity of zwitterionic hydrogels make wearable electronic devices in intimate contact with the skin, improving the sensitivity and applicability of wearable devices. In the field of tissue engineering, zwitterionic hydrogels are used as scaffolds and filling materials which can provide 3D structures that support and guide cell growth and tissue regeneration[17]. As a kind of biomaterial, they can meet the specific growth needs of various tissues without changing the chemical environment[18], providing necessary support for cell attachment, proliferation, and differentiation[19,20], creating a suitable physical and biochemical environment for tissue regeneration[21]. Additionally, based on the sensitivity of zwitterionic hydrogels to temperature, ion concentration, pH, etc.[22], it is possible to combine zwitterionic hydrogels with other functional components such as nanoparticles, bioactive molecules, or target molecules to form multifunctional drug carriers[23], which can achieve the precise release of drugs in different biological environments and achieve the purpose of treatment of diseases.
The applications of zwitterionic hydrogels in the biomedical field have yielded significant results and displayed considerable potential. However, despite some promising progress, numerous challenges and unresolved issues persist. This comprehensive review offers an in-depth analysis and summary of the applications of zwitterionic hydrogels in biomedicine, aiming to enhance understanding of this material and facilitate its broad implementation in the biomedical field. As shown in Scheme 1[24-28], we present an overview of the structures of zwitterionic hydrogels, followed by a detailed discussion of their key properties. Subsequently, our focus shifts towards exploring the utilization of zwitterionic hydrogels in diverse biomedical applications such as biosensor fabrication, flexible energy storage devices, tissue engineering, and drug delivery. By summarizing and analyzing the existing research, we address the prevailing challenges and outline future development directions, thereby providing valuable references for further research and application endeavors.
Scheme 1. Schematic illustration of the properties and biomedical applications of zwitterionic hydrogels. Reproduced with permission from[24-28]. Copyright 2020, The Royal Society of Chemistry. Copyright 2019, American Chemical Society. Copyright 2022, American Chemical Society. Copyright 2020, Wiley-VCH GmbH. Copyright 2020, Wiley-VCH GmbH.
TYPES AND PROPERTIES OF ZWITTERIONIC HYDROGELS
Types of zwitterionic hydrogels
As shown in Figure 1A, zwitterionic polyelectrolytes are classified into category (i) and category (ii)[29]. Category (i) polyampholytes are prepared by the copolymerization of anionic monomers and cationic monomers. This kind of zwitterionic polymer has oppositely charged groups located on different monomer units[30,31]. The uniformity of the charge distribution of the polyampholyte is mostly achieved by uniform mixing of a 1:1 ratio of the oppositely charged comonomers used in the copolymerization reaction[32]. These opposite charges are uniformly distributed throughout the polymer chains in the polyampholytes, making the polyampholyte molecules electrically neutral. The main chains of these polymers are usually polymerized by monomers of methacrylate, methacrylamide, acrylamide (AM), vinylbenzyl chloride, or other derivatives that contain carbon double bonds[33-35]. Category (ii) polyampholytes are obtained by the homopolymerization of zwitterionic monomers composed of an alkenyl moiety with polymerization activity and a side moiety capable of electrically neutral zwitterionization[36,37]. An alternative method for synthesizing category (ii) polyzwitterionic electrolytes involves grafting zwitterionic functional groups onto existing polymer long chains[38]. As shown in Figure 1B, the common cationic groups in zwitterionic polymers are quaternary ammonium cations, amino cations, quaternary phosphonium cations, pyridinium ions, and imidazolium ions. The main types of anionic groups, sulfonate anions, carboxylate anions, and phosphoric acid root anions [Figure 1C]. The biologically good electrically neutral structures sulfobetaine (SB), carboxybetaine (CB), and phosphorylcholine (PC) shown in Figure 1D are obtained by combining quaternary ammonium salt cations with different types of anions[39]. The structures of SB, CB, and PC are similar to natural molecules, and they can be considered biomimetic molecules. The structure of CB is similar to betaine, a natural compound associated with osmotic regulation in the human body[40]. The structure of SB is similar to taurine, a sulfur-containing non-protein amino acid found in many animals[41,42]. The structure of PC is similar to the hydrophilic part of a phospholipid, the main component of cell membranes[43]. The α-amino acids depicted in Figure 1E represent a class of natural zwitterions that can be employed in the side chains of zwitterionic polymers[44]. Furthermore, the sulfonate anion structure and carboxylate anion structure can typically combine with the cations shown in Figure 1B to generate a diverse range of derived zwitterionic polymers[45]. An illustration of partially derived zwitterionic hydrogels is presented in Figure 1F. These zwitterionic polymers can be cross-linked to form zwitterionic hydrogels which have a wide range of applications.
Figure 1. (A) Classification of zwitterionic electrolytes: Category (i) and Category (ii); (B) Cationic groups; (C) Anionic groups. Molecular structures of (D) polybetaines; (E) α-amino acid; and (F) other derived zwitterionic polymers. SB: Sulfobetaine; CB: carboxybetaine; PMPC: poly (2-methacryloyloxyethyl phosphorylcholine); TMAO: trimethylamine N-oxide derived polymer; VBIPS: 3-(1-(4-vinylbenzyl)-1H-imidazol-3-ium-3-yl) propane-1-sulfonate; PVPB: polyzwitterions based on poly (4-vinylpiridine).
Properties of zwitterionic hydrogels
The ionic conductivity performance
Conductive materials can be divided into electronic and ionic conductive materials[46]. In electronically conductive materials, electrons have the ability to move freely within the lattice, allowing the formation of an electric current. Ionic conductivity refers to the ability of ions in a medium to move in response to an electric field, resulting in the transfer of charge and, thus, the flow of current[47]. Generally, ionic conduction is predominantly found in electrolyte solutions[48] and electrolyte solids[49]. Due to the unique structure of zwitterionic materials, the anions and cations in the zwitterionic ion chains are readily separated under the influence of an external electric field. Simultaneously, the strong electrostatic interactions between oppositely charged ions facilitate ion transport in the electrolyte system, leading to charge transfer[50]. Therefore, zwitterionic hydrogels have excellent ionic conductivity. A novel antifreeze hydrogel electrolyte (polySH) was designed and fabricated by random copolymerization of a zwitterionic monomer sulfobetaine methacrylate (SBMA) and 2-hydroxyethyl acrylate (HEA) [Figure 2A]. The anionic and cationic counterions on the zwitterionic chains contribute to the dissociation of the LiCl [Figure 2B]. The polySH electrolyte exhibits the high ionic conductivity due to the electrostatic interaction between the SO3- group and hydration structure of Li+ [Figure 2C][51]. It has also been shown that the conductivity increases with the amount of SBMA in the zwitterionic nanocomposite hydrogel [Figure 2D][52]. The zwitterionic hydrogels exhibit excellent transparency compared to most electronically conducting hydrogels, thus providing a superior visual advantage[53,54]. The transparency of conductive hydrogels is crucial for the visual monitoring of wearable biosensors. A zwitterionic-clay nanocomposite hydrogel with light transmittance of 98.8% and a conductivity of 0.14 ± 0.01 S·m-1 by in situ polymerization of 3-[dimethyl-[2-(2-methylprop-2-enoyloxy) ethyl]azaniumyl]propane-1-sulfonate (DMAPS) has been synthesized in the aqueous nanoclay solution. This zwitterionic hydrogel was utilized as a self-healing human-computer interaction trackpad with pressure-sensitive adhesion[55]. The presence of equal amounts of cation and anion groups promotes the migration and dispersion of the nanoclay and enhances the mechanical properties of the hydrogel. Moreover, the interactions between polar and charged groups bound to the zwitterionic groups improve the interfacial adhesion between the matrix and the gel electrolyte. Therefore, the hydrogel touchpad can be firmly attached to the curved or flat insulator surface. This observation also suggests that zwitterionic hydrogels can be used as polymer binders.
Figure 2. (A) Schematic diagram of anti-freezing polySH hydrogel and its network structure; (B) Li+ migration mechanism in the polySH electrolyte, where hydrated Li+ hopping through SO3- sites; (C) Ionic conductivity of polySH, polyHEA, and PVA electrolyte. Reproduced with permission from[51]. Copyright 2021, Wiley-VCH. GmbH; (D) Conductivity of zwitterionic hydrogels with different SBMA contents. Reproduced with permission from[52]. Copyright 2019, American Chemical Society. SH: SBMA-co-HEA (SBMA: sulfobetaine methacrylate; HEA: 2-hydroxyethyl acrylate); HEA: 2-hydroxyethyl acrylate; PVA: poly (vinyl alcohol); SBMA: sulfobetaine methacrylate.
The non-fouling performance
The strong interactions between surface charges and water molecules at the zwitterionic polymer surface lead to an ordered and strongly hydrogen-bonded water layer. It has been demonstrated that strong surface hydration plays a significant role in determining the excellent non-fouling performance of zwitterionic hydrogels [Figure 3A)[56,57]. Previous studies have shown that the adsorption capacity of sulfobetaine vinylimidazole (polySBVI) hydrogel for non-specific proteins is extremely low, even lower than the detection line of surface plasmon resonance (< 0.3 ng·cm-2)[58]. The anti-pollution performance of various zwitterionic polymers varies and is influenced by the carbon spacer length between zwitterionic groups and the nature of the anionic groups. Research indicates that an increase in the carbon chain between the cation and anion enhances the hydrophobic/hydrophilic ratio of zwitterionic chains. For instance, the anti-protein adsorption capability of zwitterionic poly(carboxybetaineacrylamide)s with several carbon atoms between the cation and anion weakens when the carbon atom count exceeds two, as its hydrophilicity diminishes[59]. Additionally, the hydrophilicity of CO2- and PO4- is significantly greater than that of SO3-[60]. Consequently, the anti-fouling ranking is poly[carboxybetaine methacrylate (CBMA)] > poly[2-methacryloyloxyethyl phosphorylcholine (MPC)] > polySBMA.
Figure 3. (A) The hemoadsobent PCB-PAC hydrogel for detoxification of biological fluids. Reproduced with permission from[56]. Copyright 2017, Elsevier; (B) Accelerating wound healing by the antibacterial and nonfouling hydrogel. Reproduced with permission from[63]. Copyright 2020, American Chemical Society. PCB: Poly-carboxybetaine; PAC: powdered activated carbon.
The PC group is an important part of phospholipid molecules, the main component of biological membranes. Zwitterionic polymers containing these groups have properties similar to those of phospholipid molecules, and they can be used as high molecular weight materials to mimic biological membranes[61]. The PC group mimics the outer cell membrane. The phospholipid head group is presented on the surface, leading to the hydration of the polymer coating, considered the primary mechanism for reducing protein and bacterial adhesion[62]. This creates a favorable environment for wound healing and helps prevent wound infection, contributing to the overall healing process. Simultaneously, the zwitterionic hydrogel is loaded with curcumin, which is then released to eliminate bacteria [Figure 3B][63]. The MPC is the most classic PC-type zwitterionic monomer, and polyMPC has been widely used because of its good biocompatibility[64,65]. In addition, choline phosphate (CP) groups with anti-fouling properties obtained by exchanging the anion and cation groups of PC can also be applied to zwitterionic polymers to create complementarity and functional diversity with the PC[66,67].
The structure of polyCBMA is similar to glycine betaine, a compound naturally present in the human body[68], and its anionic group, -COO-, serves as a means to immobilize biomolecules. Research has demonstrated that polyCBMA can effectively immobilize specific antibodies, enabling the detection of 100% of antigens in plasma without the adsorption of non-specific proteins[69]. Other materials, including polyethylene glycol (PEG), cannot achieve this. The -COO- anionic group is protonated at low pH, making the copolymer positively charged, which makes the polyCBMA hydrogel have protein adsorption behavior at low pH.
The polySBMA hydrogels are completely deprotonated over the wide pH range, and their anti-protein adsorption behavior is not easily affected by pH[70]. The structure of SB exists in high concentration in nature. A 70 kg human contains up to 70 g of taurine which is structurally similar to SB, making polySBMA a promising biocompatibility material[71]. The mechanically responsive drug controlled drug release, antibacterial, and anti-sewage hydrogel has been synthesized by the SBMA monomer[72]. However, due to the anti-polyelectrolyte effect of polySBMA, the protein adsorption capacity of polySBMA hydrogels was slightly increased at high ion concentrations[73].
The thermosensitivity and pH-responsive property
Zwitterionic hydrogels possess unique properties attributed to their distinct structures. The anions and cations within zwitterionic hydrogels exhibit both electrostatic association and repulsion. These electrostatic interactions undergo changes when subjected to temperature variations, leading to a transition between a dispersed micellar state and a dense 3D network structure. This phenomenon is known as the thermosensitivity of zwitterionic hydrogels. Two types of thermosensitive effects are observed: lower critical solution temperature (LCST) and upper critical solution temperature (UCST). In the case of LCST-type thermosensitive polymers, zwitterionic hydrogels are soluble at lower temperatures. However, upon heating above the LCST, the polymer molecules precipitate out of the solution and undergo a sol-gel phase transition. Conversely, UCST-type thermosensitive polymers cannot be dissolved below a specific temperature (UCST temperature). These polymers dissolve when heated above the UCST temperature[74]. The poly (3-dimethyl (methacryloyloxyethyl) ammonium propane sulfonate (PDMAP) containing the SB structure is one of the extensively studied temperature-responsive polyzwitterions, exhibiting a high UCST in aqueous solutions. The UCST of PDMAP is strongly influenced by several factors, including concentration, molar mass, and branching coefficient[75]. Various approaches can be employed to alter the UCST of PDMAP. For example, increasing the length of a linear alkyl substituent on the nitrogen atom gradually can shift the UCST toward the LCST. A series of zwitterionic poly(sulfobetaine methacrylate)s with different substituents, including n-alkyl, cyclohexyl, 2-hydroxyethyl, and phenyl groups, were synthesized. When the length of two symmetric n-alkyl substituents is simultaneously increased, polySBMAs transition from the UCST to the soluble LCST, and finally to insolubility in water. This transition is thought to arise from the competition between the decrease in electrostatic zwitterionic interactions and the increase in hydrophobic effects associated with longer alkyl chains[76]. Additionally, incorporating hydrophilic spacer groups into the betaine moiety can effectively separate the cationic and anionic moieties, elevating the cloud point[77]. Co-polymerization of polySB with another temperature-responsive monomer N-vinylcaprolactam (VCL) allows the synthesis of dual-temperature-responsive low surface-contaminated zwitterionic microgels [Figure 4A]. The microgels showed excellent antifouling properties, and they can be potentially used for temperature-triggered drug delivery and anti-bioadhesion coating material[78]. Modification of polyamide amine (PAMAM) dendrimers with phenylalanine (Phe) and succinic anhydride (Suc) to produce a carboxy-terminal Phe-modified zwitterionic dendrimer called PAMAM-Phe-Suc[79]. This amphoteric substance exhibits UCST-type thermal sensitivity. Interestingly, PAMAM-Phe-Suc displayed LCST-type thermal sensitivity at lower pH values [Figure 4B]. This is due to the protonation of the terminal carboxyl and tertiary amino groups of the polymer.
Figure 4. (A) Microstructures of PVCL-co-PGMA-g-PSB microgels at different transition temperatures. Reproduced with permission from[78]. Copyright 2020, American Chemical Society; (B) Relationship between the solubility and the protonation state of the ionic groups in PAMAM-Phe-Suc. Reproduced with permission from[79]. Copyright 2020, Royal Society of Chemistry. LCST: Lower critical solution temperature; PSB: poly(sulfobetaine); PVCL: poly(N-vinylcaprolactam); UCST: upper critical solution temperature; PGMA: poly(glycidylmethacrylate); PAMAM: polyamide amine.
Protonation leads to the positively charged state of zwitterionic hydrogels. The relationship between the degree of protonation (α) and the pH can be calculated using equation (1):
The pKa represents the acid dissociation constant of the hydrogen ion[80]. It can be deduced from equation (1) that as the pH decreases, the degree of protonation increases. Functional groups on the zwitterionic polymer chains become protonated, transforming into cationic entities capable of interacting with negatively charged substances. Conversely, as the environmental pH increases, the extent of deprotonation rises. The functional groups on the zwitterionic polymer chains undergo deprotonation, turning into anionic entities that can readily interact with cationic substances. This reflects the pH responsiveness of the zwitterionic polymers. The pH responsiveness changes the internal charge state of hydrogels through protonation and deprotonation, subsequently affecting the structures and properties of hydrogels. This property can be utilized in drug delivery systems to achieve controlled drug release by modulating the swelling and deswelling of hydrogels[81]. Moreover, it can also be employed in the design and fabrication of smart materials, such as zwitterionic hydrogels with pH-responsive shape memory behavior[82].
The anti-polyelectrolyte effect
The presence of ions will weaken the self-association effect of the polymer networks in zwitterionic hydrogels; thus, the networks exhibit special ion sensitivity. The polymer chains segment is relaxed, and the volume of the hydrogel expands when the zwitterionic hydrogels are placed in a certain concentration of electrolyte solution after they have reached swelling equilibrium in pure water. This is because ions in the electrolytes can screen for interchain/intrachain electrostatic associations, leading to cross-link dissociation and zwitterionic chain expansion, exposing more hydratable ionic groups and increasing water flux. This phenomenon is different from the behavior of traditional polyelectrolyte hydrogels, and it is called the “anti-polyelectrolyte effect”[83]. As early as 2002, the anti-polyelectrolyte effect of zwitterionic hydrogels was reported[84]. Additionally, the polySBMA is more susceptible to salt concentration than the polyCBMA, because the proper hydrophobicity and strong intrinsic electrostatic binding strength of the SBMA improve its ability to bind ions in the water[85]. To further investigate the anti-polyelectrolyte effect of different zwitterionic hydrogels, the electrostatic binding strength of different zwitterionic pairs was verified in detail and compared: MPC < CBMA < SBAA < SBMA. This order is positively correlated with the degree of anti-polyelectrolyte effect[86]. Despite the fact that salts are known to significantly affect the association of ionic bonds in zwitterionic hydrogels, chemical cross-links in the hydrogel network structure are not disrupted [Figure 5A]. Not only does it provide guidance for the biological applications of zwitterionic hydrogels in the saline environment, but it also provides important insights into the mechanism of hydrogel toughening[87]. For example, an instant network of the polySBMA hydrogel with anti-pollution ability was created based on ion concentration. The ion dissociation degree within the polySBMA hydrogel was adjusted by varying the ion concentration, thereby making the polySBMA hydrogel a candidate for preventing peritoneal adhesions after surgery[88]. The introduction of the hydrophobic benzsulfamide group in (2-((2-(methacryloyloxy)ethyl)dimethylammonio)acetyl)(phenylsulfonyl)amide (MEDAPA) has simultaneously exhibited the UCST and the anti-polyelectrolyte effect[89]. Their friction coefficients decrease with increasing salt concentration and temperature, and the polymer chains extend, resulting in a larger hydrodynamic size of the hydrogel [Figure 5B and C]. This shows great potential as a zwitterionic regenerative interface and has potential applications in smart separation membranes or responsive drug delivery systems. However, polyCBMA hydrogels generally exhibit no UCST and a weak anti-polyelectrolyte effect. It is generally believed that the COO- on the side groups of polyCBMA is a weak electrolyte, which is not completely dissociated in solution. Therefore, polyCBMA exhibits a strong hydration effect that hinders self-association between polymer segments[90].
Figure 5. (A) The salt effect on the crosslinked networks in polyampholyte (PA) gels without chemical crosslinking (i) and with chemical crosslinking (ii). Reproduced with permission from[87]. Copyright 2023, American Chemical Society. The (B) anti-polyelectrolyte effect and (C) thermal responsiveness of PMEDAPA hydrogel. Reproduced with permission from[89]. Copyright 2019, The Royal Society of Chemistry. PA: Polyampholyte; PMEDAPA: poly(2-((2-(methacryloyloxy)ethyl)dimethylammonio)acetyl)(phenylsulfonyl)amide.
BIOMEDICAL APPLICATIONS OF ZWITTERIONIC HYDROGELS
Implantable biosensors
Chemical, physiological, or biological signals related to organisms are sensed, detected, and translated into measurable physical signals by implantable biosensors in vivo to achieve real-time monitoring and analysis of the state of organisms[91]. However, implantable biosensors face significant challenges related to stability and reliability. These challenges encompass the deactivation of biomaterials serving as recognition elements and the occurrence of false-positive signals due to nonspecific adsorption of proteins, bacteria, and platelets to the surface of the biosensor[92]. Furthermore, concerns arise from limitations in the transport of analytes caused by fibrotic capsules and thrombus formation, along with considerations regarding the service life of the biosensor[93]. Additionally, achieving conformal contact and bonding between biosensors and biological tissues in vivo emerges as a primary challenge that profoundly influences the overall stability and reliability of biosensors[94]. Effectively addressing these challenges is crucial for advancing the field of implantable biosensors and unlocking their full potential in diverse biomedical applications.
As a biocompatible polymer, zwitterionic hydrogel holds considerable importance in biodetection. Its applications in implantable biosensors play a crucial role in enhancing the detection performance of such biosensors. For instance, the remarkable capabilities of zwitterionic hydrogels in biomolecular detection can be realized by incorporating suitable biomolecular recognition elements (antibodies, enzymes, and aptamers) or implementing physicochemical modifications (such as charge, hydrophilicity, and hydrophobicity) into the hydrogel structure[95-97]. Through electrostatically induced hydration, the zwitterionic hydrogel interacts with water molecules, effectively pulling water away from the hydrophobic regions of the biomolecular recognition elements, which minimizes any detrimental effects on the activity of the biomolecular elements while simultaneously promoting strong interactions between the substrate and recognition elements[98]. The study has shown that the immobilized horseradish peroxidase (HRP) and laccase by the polyCB hydrogel significantly enhanced kinetic parameters, with the Kcat/Km values being 5.5 times[16] and 20 times[99] higher, respectively. This remarkable improvement enables the realization of highly sensitive detection of target substances. Additionally, we explored the polyCB hydrogel as an effective immobilization carrier for α-chymotrypsin (ChT). The results indicated that the zwitterionic hydrogel increased the inactivation temperature of ChT by an impressive 13 °C while extending its half-life by seven times[100]. These findings highlight the potential of zwitterionic hydrogels as valuable tools for enhancing enzyme stability and activity. This holds promising implications for various applications in biocatalysis and sensitive substance detection. Consequently, zwitterionic hydrogels can be employed for the immobilization of biomolecular recognition elements in implantable biosensors.
To ameliorate the issues of bacterial contamination and fibrous capsule formation on surfaces of biosensors in vivo, their surface modifications[101] have been developed, but with only limited success. PEG and its derivatives are known for forming a robust hydration layer through hydrogen bonding with water, thereby enhancing anti-biofouling properties[102]. However, PEG has drawbacks, such as hydrolysis and autooxidative degradation, leading to reduced antifouling efficacy over time[103]. As a promising alternative, poly-zwitterionic materials have shown excellent potential as effective anti-biofouling coatings[104,105]. The zwitterionic peptide hydrogel was successfully immobilized on a glass carbon electrode modified with conductive polymer poly(3,4-ethylenedioxythiophene) (PEDOT) and gold nanoparticles (AuNPs) through Au–S bonds. This enabled the immobilization of prostate-specific antigen (PSA) on the electrode surface, resulting in a highly sensitive antifouling biosensor with a low detection limit of 5.6 pg/mL for PSA, outperforming previous reports. The biosensor exhibited remarkable stability in serum, with less than 5% signal change after five hours in 20% serum, whereas the amphotericin-modified biosensor experienced around 20% signal change, highlighting the excellent stability of the zwitterionic sensor[106]. The potential of poly-zwitterionic materials was demonstrated by developing an electrochemical sensor modified with glucose oxidase (GOx) immobilized by sulfonate polySBMA-3,4-ethylenedioxythiophene (EDOT). This sensor exhibited high sensitivity (10.54 μA·cm-2·mM-1) for blood glucose detection after initial contact with the plasma, and it retained 90% of the current signal even after 14 days of storage in 100% human plasma. After 90 days, more than 14% of the current signal was still retained, showcasing the long-term stability of the zwitterionic-coated sensor. In contrast, the PEDOT-GOx-modified glucose sensor without zwitterionic coating lost more than 50% of its current signal after seven days in plasma and became completely ineffective after three weeks. This study confirmed that sulfonate betaine not only preserved enzyme activity but also exhibited excellent anti-protein and anti-biological contamination properties, significantly enhancing the stability and service life of the sensor[107]. Furthermore, a zwitterionic polymer coating was developed and applied to Medtronic continuous glucose monitoring (CGM) to reduce noise due to inflammation during CGM monitoring. The coated sensor shown in Figure 6A showed significant improvement, effectively eliminating sensing noise and accurately recording blood glucose levels after the first glucose measurement without recertification. This study demonstrates the capability of zwitterionic hydrogels to resist contamination and enhance the biocompatibility of implantable medical devices with living organisms[108]. However, as biocoatings for in vivo biosensors, zwitterionic hydrogels also need to exhibit excellent mechanical properties. Achieving anti-protein and anti-biological contamination properties requires maintaining good hydrophilicity, which could potentially compromise the mechanical integrity of zwitterionic hydrogels. To address this, certain studies[109,110] have developed zwitterionic hydrogels with anti-biological contamination, anti-swelling, and robust mechanical properties by combining carboxylic polyCB and polySB phases. The polyCB is the minor component while polySB is the major component, taking advantage of the high swellability of the polyCB network to integrate a considerable amount of the polySB network. The polySB network locks the entire zwitterionic hydrogel via strong intermolecular and intramolecular electrostatic interactions [Figure 6B], achieving polyCB/polySB zwitterionic-elastomeric-networked (ZEN) hydrogel with high mechanical strength, anti-swelling and anti-biological contamination properties, derived solely from pure zwitterionic components[111]. This innovative approach achieves a balance of exceptional mechanical properties and effective anti-fouling characteristics.
Figure 6. (A) A continuous glucose monitor by coating the sensor with a zwitterionic polymer. Reproduced with permission from[108]. Copyright 2018, Springer Nature; (B) The polyCB/polySB ZEN hydrogels: design principles, high mechanical properties, equilibrium swelling ratios and equilibrium water contents. Reproduced with permission from[111]. Copyright 2021, American Association for the Advancement of Science; (C) PDA-clay-polySBMA hydrogels: the schematic illustration, the adhesion mechanism, the hydrogel sensor for monitoring tissue vibration. Reproduced with permission from[24]. Copyright 2020, The Royal Society of Chemistry. CGM: Continuous glucose monitoring; ZEN: zwitterionic-elastomeric-networked; CB: carboxybetaine; SB: sulfobetaine; PDA: polydopamine; SBMA: sulfobetaine methacrylate.
In addition to addressing biological contamination, ensuring conformal contact between sensors and target biological tissues or organs is crucial for enhancing biosensor stability and reliability. Thus, sensors require modifications with conductive, highly stretchable, durable, and tissue-adhesive materials. Zwitterionic hydrogels with remarkable stretchability, self-healing capabilities, rapid recovery, and fatigue resistance were developed by cross-linking poly zwitterionic polymers (sulfonate betaine) with dopamine-modified clay nanosheets. The resulting nanocomposite zwitterionic hydrogel is not only highly stretchable but also self-healing. Notably, this zwitterionic hydrogel demonstrates an impressive ionic conductivity of
In implantable biosensors, challenges such as poor stability, leading to false-positive signals, and difficulties in combining target analytes with the biosensor due to protein contamination coating the sensor are prevalent. Zwitterionic hydrogels, known for their good biocompatibility, high hydrophilicity, and excellent anti-fouling properties, are extensively utilized in implantable biosensors. However, these hydrogels often exhibit mechanical weaknesses and susceptibility to water absorption and swelling, posing challenges for long-term sensor applications. This section summarizes various methods aimed at enhancing the mechanical properties of zwitterionic hydrogels, including the use of composites with distinct properties, incorporation of other polymers and nanoparticles, etc. These approaches bear significant implications for the effective application of zwitterionic hydrogels in implantable biosensors.
Flexible wearable sensors
Flexible wearable biosensors provide continuous and real-time data for human health monitoring and diagnosis by obtaining physiological information about organisms in a non-invasive or minimally invasive form. Central to the advancement and widespread adoption of biosensor technology is the detection of physiological signals, human-computer interaction, and the integration of flexible electronics. Zwitterionic hydrogels, known for their conductivity and sensitivity to external stimuli, have been utilized as flexible sensing materials[112,113]. Zwitterionic hydrogels achieve excellent flexibility and stretchability by optimizing their composition, structure, and mechanical properties, making them ideal substrate materials for fabricating flexible sensors. These flexible sensors can seamlessly conform to the contours and shapes of the human body, significantly enhancing the comfort and portability of wearable sensors.
Zwitterionic hydrogels are utilized as strain sensors to monitor human movement, posture, body state, and physiological conditions. When the sensor detects an external deformation, the internal ion motion of the zwitterionic hydrogel undergoes changes, resulting in a modification of its conductivity. The polyCBMA hydrogel has exhibited water molecule dissociation under pressure, facilitating ion transport through the cation and anion migration channels [Figure 7A]. Consequently, the ion current transiently increased at low pressure, resulting in a rapid signal response time of approximately 38 s. This sensor has been employed in a throat-wearable silent speech recognition system, where it converted subtle mechanical vibrations of the larynx into silent speech[114]. However, zwitterionic hydrogels are susceptible to rupture under external forces due to their limited mechanical properties. To address this limitation, several studies have explored the incorporation of nano-reinforcement systems and molecular co-design into zwitterionic hydrogels, significantly enhancing their compressive and tensile strength capabilities. For example, sulfonate betaine and nanoclay were polymerized in situ, resulting in effective nanoclay dispersion within the zwitterionic polymer and promoting full cross-linking with the zwitterionic polymer. The resulting hydrogels exhibited remarkable properties, including a high elongation at a break of 2,000%, fracture strength of up to 0.27 MPa, and fracture toughness of 2.45 MJ·m-3[52]. Cellulose nanocrystals were used as the physical cross-linking agent and reinforcing agent, and UV-initiated partial chemical cross-linking of SBMA and AM monomers to form the nanocomposite hydrogel [Figure 7B]. The addition of cellulose crystals significantly enhanced the mechanical and tensile properties of the hydrogel, leading to exceptional tensile properties with more than 1,000% strain, mechanical tensile strength of up to 0.61 MPa, and compressive strength of up to
Figure 7. (A) The zwitterionic polyCBMA hydrogel deformed under a tiny pressure and generates ions in a pure water system with no additional mobile ions. Reproduced with permission from[114]. Copyright 2023, Springer Nature; (B) A plant-inspired zwitterionic hydrogel was prepared through rapid UV initiation in the existence of cellulose nanocrystals as a physical crosslinker and reinforcing agent. Reproduced with permission from[25]. Copyright 2019, American Chemical Society; (C) High mechanical properties of zwitterionic hydrogel with multi-molecule cooperation. Reproduced with permission from[115]. Copyright 2022, Elsevier; (D) The strong tough PA hydrogel via the synergy of ionic and metal-ligand bonds. Reproduced with permission from[117]. Copyright 2021, Wiley-VCH. GmbH. SBMA: Sulfobetaine methacrylate; AA: acrylic acid; CBMA: carboxybetaine methacrylate; PA: polyampholyte.
Current research on bionic electronic skins has predominantly focused on their strain-stress responses, limiting their practical applications in recognizing multiple stimuli. To address this, a smart zwitterionic conductive hydrogel with a dual network structure (SAA: NaCl/sodium alginate/polyacrylic-AM) for biomimetic E-skin was developed[120]. This SAA hydrogel exhibited high sensitivity to both strain-pressure and temperature changes, with different types of stimulation easily distinguishable through the electrical signal waveform. As a result, SAA zwitterionic hydrogels hold significant promise as biomimetic electronic skins capable of responding to multiple stimuli for motion monitoring, human-machine interfaces, and soft robotics. In a separate study, the zwitterionic hydrogel was prepared by combining SBMA with glucose-sensitive molecule (3-methacrylamidophenyl) boronic acid (MPBA), creating a specific binding to glucose molecules[121]. The biosensor fabricated with this hydrogel not only enabled highly sensitive pressure detection, including gentle finger touch and vocal fold vibration, but also exhibited highly sensitive glucose detection [Figure 8A]. However, the simplicity of the monolayer structure may lead to signal interference between different stimuli. Thus, the team employed the synergistic effect of SBMA with good conductivity and healing, N-isopropylacrylamide (NIPAAm) with excellent temperature sensitivity, and MPBA with strong glucose response to fabricate a three-layer structure of a temperature-glucose insensitive capacitive sensor, effectively responding to strain and capacitance [Figure 8B][122]. This innovative approach enables continuous and stable real-time monitoring and differentiation of pressure, temperature, and glucose concentration signals and simultaneously promotes diabetic wound healing. It is important to be able to monitor wound status during wound healing. Lee et al. developed Basic amino acid-modified polyimide, which is expected to be used to monitor wounds, protect them from microbial infection, and promote wound healing[123].
Figure 8. (A) A zwitterionic-aromatic motif-based conductive hydrogel for a strain and glucose-responsive ionic skin sensor. Reproduced with permission from[121]. Copyright 2021, Elsevier; (B) A sandwich-structured sensor based on multi-response zwitterionic skin for multiple sensations and pro-healing. Reproduced with permission from[122]. Copyright 2021, Wiley-VCH GmbH. SBMA: Sulfobetaine methacrylate; NIPAAm: N-isopropylacrylamide; MPBA: (3-methacrylamidophenyl) boronic acid.
This section primarily delves into the development and application of flexible wearable biosensors. These sensors capture physiological information from organisms in a noninvasive or minimally invasive manner, offering continuous, real-time data for human health monitoring and diagnosis. Core technologies encompass physiological signal detection, human-computer interaction, and the integration of flexible electronics. Zwitterionic hydrogels, owing to their electrical conductivity and sensitivity to external stimuli, prove ideal for flexible sensing materials. Widely employed in strain sensors for monitoring human movement, posture, body state, and physiological conditions. However, zwitterionic hydrogels face challenges such as susceptibility to rupture under external forces. To address this, some studies utilize nano-strengthening systems and molecular co-design to enhance compressive and tensile strength. This innovative approach yields zwitterionic hydrogels with notable properties, including increased elongation, strength, and toughness at break. Researchers also explore the applications of zwitterionic hydrogels in biomimetic electronic skin, sound recognition, glucose detection, and various other areas. The introduction of intelligent zwitterionic conductive hydrogels enables responses to multiple stimuli, facilitating motion monitoring, human-machine interfaces, and soft robotics.
Energy storage devices
Flexible energy storage devices can supply energy to implantable and wearable medical devices, enabling medical devices to operate stably without external power supplies[124]. With the increasing prevalence of implantable and wearable medical devices, flexible energy storage devices matching them have attracted much attention, so it is necessary to develop flexible energy storage devices. At present, flexible energy storage devices that have received more attention mainly include batteries and supercapacitors. In order to meet the needs of diverse flexible devices, flexible energy storage devices need to be flexibly installed and applied on surfaces of various shapes and sizes while maintaining their flexibility, stretchability, and resilience to external forces. In this context, zwitterionic hydrogels have garnered significant attention as promising materials for the fabrication of flexible energy storage devices due to their remarkable water absorption capabilities and soft nature, which meet the basic requirements of flexible energy storage devices. Electrochemical energy storage devices generally consist of two electrodes separated by the electrolyte[125]. Traditional hydrogel electrolytes are mainly based on polyacrylamide (PAM), polyAA, polyvinyl alcohol matrices, sodium polyacrylate, gelatin, and so on[126-128]. Although these hydrogel electrolytes provide physical frameworks to support ion transport, improvements are needed in aspects such as the electrochemical stable potential window (ESPW) and ion transport efficiency when electrochemical reactions occur. Zwitterionic polymers are charged with unique zwitterionic groups in the repeat units. These polymers modified with ion channels can effectively promote salt dissociation in electrolytes and increase ionic conductivity[129].
Immobilization of zwitterionic surfactants on the surface of the polymer matrix provides a stable ionic gel. Zwitterionic dipole structures induce ion-dipole interactions facilitating the dissociation of ionic complexes. In the lithium iron phosphate half-cell test, the enhanced lithium ion conductivity in the zwitterionic hydrogel had a positive impact on the lithium plating/stripping behavior and performance, achieving optimal rate capacity which is 116.8 mAh·g-1 at 1 °C and initial discharge capacity of 148.9 mAh·g-1 (Volume decay rate = 0.069% per cycle)[130]. For aqueous zinc metal batteries, the charged groups of zwitterionic polymers can homogenize the ion distribution and achieve uniform zinc deposition. The polyzwitterionic hydrogel electrolyte (PZHE) prepared by SBMA achieved an ion conductivity of 32.0 mS·cm-1 at room temperature and a Zn2+ transference number of 0.656. With the PZHE, a stable cycle life of about 3,500 h can be achieved for the Zn metal anode at the current density of 3,500 mA·cm-2. The use of PZHE to fabricate full cells paired with various cathodes had high self-healing properties[131]. However, the salt-like structure of the polyzwitterion usually leads to the fragility of hydrogels, so reliable methods are needed to enhance the mechanical properties without losing the energy storage effect. Cellulose nanofibers are rich in hydroxyl groups, and their excellent hydrophilic framework can be used to improve the hydrophilicity of hydrogels, which are often used as reinforcing agents[132]. Cellulose nanofibers have been widely used as reinforcing agents due to their excellent hydrophilic skeleton which can improve the hydrophilicity of hydrogels. A novel zwitterionic hydrogel electrolyte, in which [2-(methacryloyloxy)ethyl]diethy-(3-sulopropyl) (MAEDS) was combined with cellulose to form a semi-interpenetrating network (ZSC-gel) [Figure 9A], was used in aqueous Zn-MnO2 cells. The interlacing microporous network formed in the hydrogel stabilized the ion transport channels while improving the mechanical properties, resulting in a greater rate capacity of the hydrogel electrolyte. The Zn-MnO2 battery with ZSC-gel exhibited a high capacity of 148 mAh·g-1 at 6.5 °C with a retention of 90.42% of the initial capacity after 1,200 cycles. The flexible planar and fiber-shaped batteries fabricated by the designed ZSC-gel electrolyte exhibited excellent flexibilities and weavabilities[133]. In addition, zwitterionic hydrogel electrolytes maintain good mechanical properties (modulus, strength, and toughness) in the complex biological environments, and this is a prerequisite for the long-term operation of energy storage devices. The double cross-linking network (DN), with strong reversible hydrogen bonds and electrostatic forces, can dissipate and disperse energy and quickly return to its initial shape after unloading. For example, a poly(acrylamide3-co-(sulfobetaine methacrylate)1)@polyacrylamide (P(A3-co-S1)@PAM) hydrogel electrolyte with unique DN [Figure 9B] maintained excellent elasticity even after being immersed in solutions with different pHs for more than seven days. Zinc-ion batteries and supercapacitors equipped with this DN hydrogel electrolyte exhibited excellent electrochemical stability electrolytes of different pHs[134]. In addition, the polar and charged groups associated with the zwitterionic groups can enhance the interfacial adhesion between the electrode and the gel[135], so zwitterionic hydrogels are suitable for use as polymer electrolytes for flexible batteries, and the introduction of zwitterionic polymers into the field of energy storage is very promising.
Figure 9. (A) Wearable applications of the zwitterionic sulfobetaine/cellulose hydrogel electrolyte: (i) semi-interpenetrating networks of the ZSC-gel; (ii) synthesis of the ZSC-gel; (iii) wearable applications of the polyzwitterion-battery. Reproduced with permission from[133]. Copyright 2020, Wiley-VCH; (B) The DN zwitterionic hydrogel electrolyte used for zinc-ion batteries and PANI-based supercapacitors: (i) structures of the first and second PAM network hydrogels; (ii) hydrogen bond or electrostatic interactions; (iii) the mechanism diagram of compressible progress; (iv) the hydrogel electrolyte interacting with water molecules and cations including H+, K+, Zn2+, and Mn2+, or anions including OH-, AC-, and SO42-; (v) GCD cycling curves of the P(A3-co-S1)@PAM devices before and after 50% compression dipped in pH = 14 and pH = 1 solutions; (vi) tensile stress-strain curves of P(A3-co-S1)@PAM hydrogel immersing into the mixed solution of 1 M ZnSO4 and 0.2 mL MnSO4 (pH = 6.5) for 0-7 days. Reproduced with permission from[134]. Copyright 2023, Wiley-VCH GmbH. MAEDS: [2-(methacryloyloxy)ethyl]diethy-(3-sulopropyl); PAM: polyacrylamide; PANI: polyaniline; GCD: galvanostatic chargeing discharge.
As important electrochemical energy storage devices, the supercapacitors have attracted extensive attention due to their unique advantages of fast charging and discharging ability, long cycle life and high power density[136]. They complement batteries for energy storage, especially where high power delivery is required. Polymer gel electrolytes and substrates, which are key parameters for portable and wearable device applications, have come a long way in recent years. In particular, the excellent adhesion of zwitterionic hydrogels enhances the interfacial contact between the electrodes and the electrolytes, which broadens the operating voltage of the capacitor. In Figure 10A, the introduction of betaine with zwitterionic structure into the polyAA system increased the interface adhesion between the electrolyte and the electrodes and widened the operating voltage of the zwitterionic hydrogel electrolyte. The zinc-ion hybrid supercapacitor (ZIHS) achieved high energy density and cycling stability in a voltage window of 0-2.2 V with the synergistic action of zinc sulfate heptahydrate. In addition, the excellent self-healing performance of the zwitterionic hydrogel electrolyte prolonged the service life of the supercapacitor[137]. In addition to zwitterionic monomers, anionic polymer (carboxymethyl cellulose) and cationic monomer [methyl acrylamide propyl trimethylammonium chloride (MAPTAC)] can be combined to prepare the zwitterionic conductive hydrogel with 1.70 MPa compressive strength, which can be used in supercapacitors with flexible activated carbon electrodes. A high operating voltage of 2.1 V was achieved, and its capacitance remained at 94.4% even after 10,000 cycles[138]. DN hydrogels are important for improving the mechanical properties and lifetime of supercapacitors. Based on the natural polymer backbone, acrylic acid (AA) and AM containing -COOH were introduced to construct a DN hydrogel and used to assemble a solid-state ZIHS (H-ZHS) with zinc foil and activated carbon electrode, which had an amazing wide and stable voltage window of 2.4 V. A high maximum energy density of 286.6 Wh·kg-1 at a power density of 220 W·kg-1 after 2,000 cycles at 2 A·g-1, the capacity retention rate was as high as 95.4% calculated from the mass of the activated carbon electrode[139]. Zwitterionic hydrogels can not only be used as hydrogel electrolytes but also can be used to fabricate integrated supercapacitors with sandwich structure [Figure 10B], which is of great significance for achieving the stability and multimodal response of wearable electronic devices. Some studies have immersed a DN zwitterionic hydrogel with thermal response and anti-swelling properties into a pyrrole (Py) solution, which was polymerized in situ into a continuous polypyrrole (PPy) pathway, and an integrated supercapacitor with sandwich structure was obtained by controlling the growth path of PPy. The supercapacitor had an ultra-high capacitance (299.79 mF·cm-2 at 0.5 mA·cm-2) and power density
Figure 10. (A) Zinc-ion hybrid supercapacitor based on zwitterionic hydrogel electrolytes: (i) design strategy; (ii) self-healing demonstration of ZIHS based on PAB40-Zn hydrogel; (iii) capacity recovery efficiency of ZIHS under different cutting/healing cycles; (iv) pictures of PAB40-Zn hydrogel adhered to various materials. Reproduced with permission from[137]. Copyright 2023, Elsevier; (B) All-in-one SCs with PVA/PAS-PPy gel: (i) the synthesis process of PVA/PAS-PPy gel; (ii) Sensing performance comparison before and after immersion in deionized water for 30 days; (iii) SCs are assembled to power a red LED. Reproduced with permission from[26]. Copyright 2022, American Chemical Society. AA: Acrylic acid; PVA: poly (vinyl alcohol); PAS: poly (acrylamide-co-[(3-(1-(4-vinylbenzyl)-1H-benzo[d]imidazole-3-ium-3-yl)propane-1-sulfonate); PPy: polypyrrole; ZIHS: zinc-ion hybrid supercapacitor; PAB: poly (acrylic acid-betaine); SCs: supercapacitors.
In addition, medical monitoring and treatment technologies are needed to monitor vital signs and ensure the safety of people working in polar medicine, marine research, or living in areas with high humidity. Therefore, biosensors and electrochemical energy storage devices sometimes need to adapt to extreme environments. However, hydrogels are prone to freeze at low temperatures and lose water at high temperatures, causing hydrogels to lose their existing properties. In order to overcome these problems, much effort has been made. We summarize the works of zwitterionic hydrogels able to adapt to wide temperature ranges in the last three years and present the applications and some properties of these zwitterionic hydrogels in Table 1[51,140-156].
The applications and properties of zwitterionic hydrogels with wide temperature ranges
Applications | Zwitterionic hydrogels | GF (tensile strain) | Conductivity (temperature) | Specific capacitance (temperature) | Energy density | Stretchability (tensile strength) | Toughness (MJ·m-3) | Operating temperature range (°C) | Ref. |
Sensor | PSM/TF/Zn2+/ MXene | 5.62 (0%~200%) | 30 mS·cm-1 (20 °C); 34 mS·cm-1 (40 °C); 14 mS·cm-1 (-40 °C) | / | / | 970% (0.31 MPa) | 0.2 | -60~40 | [140] |
Sensor | polySBMA-LM@PDA | 0.07 (0%~100%) 0.34 (100%~200%) 0.83 (200%~500%) | 0.45 S·m-1 | / | / | 1,555% (1.3 MPa) | 7.3 | 20~80 | [141] |
Sensor | agarose/poly (HEAA-co-AA)/ Ca2+ | / | 10.18 mS·cm-1 | / | / | 2,549.62% (1.23 MPa) | 12.54 | -80~25 | [142] |
Sensor | poly (SBMA-co-AA)/Li+ | 2.08 | 110 mS·cm-1 | / | / | 800% (0.06 MPa) | -80~25 | [143] | |
Sensor | Ca-GG/ PAAm-ZP | 1.76 (0%~120%) 3.6 (120%~250%) 4.68 (250%~500%) | 2.15 mS·cm-1 | / | / | 1,800% | 3.27 | -40~25 | [144] |
Sensor | ZIL/LysMA/ Am | 14.97 (100%~1,000%) | 24.86 mS·cm-1 | / | / | 2,060% (340 kPa) | 336 | -40~25 | [145] |
Sensors | PDMAP/ PHEMA | 1.49 (0%~100%) 2.25 (100%~500%) | 0.8 S·m-1 (60 °C) | / | / | 1,033% (123.0 kPa) | 0.07 | -60~60 | [146] |
Sensor | PGMG | 2.22 (0%~170%) 3.32 (170%~310%) 4.53 (310%~500%) | 0.79 mS·cm-1 | / | / | 1,124% (0.65 MPa) | 3.87 | -40~25 | [147] |
Sensor | ADM | 1.96 (500%~1,000%) | 5.81 × 10-6 S·cm-1 | / | / | 2,700% (0.24 MPa) | -20~80 | [148] | |
Sensor/ Capacitors | CP/EGZn/ betaine | / | 21~88.9 mS·cm-1 | 102.5~212.1 mAh·g-1 | 85.7~169.7 W·h·kg-1 | 2,150% (0.49 MPa) | 0.06 | -40~80 | [149] |
Sensor/ Supercapacitor | polySBMA/ HEA/Li+ | / | 12.6 mS·cm-1 (-40 °C) | 178 mF·cm-2 (60 °C); 134 mF·cm-2 (-30 °C) | / | 325% | / | -30~60 | [51] |
Sensor/ Capacitor | VBIPS/HEAA-SA/Fe3+ | / | 0.070 S·cm-1 (9 °C) | / | / | 260% (120 kPa) | / | -60~25 | [150] |
Supercapacitor | poly(SBMA- co-HEAA)/Li+ | / | 25.8 S·m-1 (25 °C); 2.21 S·m-1 (-40 °C) | 218.2 F·g-1 (1 A·g-1) (25 °C); 154 F·g-1 (1 A·g-1) (-20 °C) | / | 400% (45 kPa) | / | -40~25 | [151] |
Hybrid capacitor | TC-Zn/p(SBMA- co-AA) | / | 15.6 mS·cm-1 (-60 °C) | 81.5 mAh·g-1 | 80.5 Wh·kg-1 | 420% (2.67 MPa) | 0.02 | -60~25 | [152] |
Supercapacitor | PVA/P(AAm-VBIPS)-EMIMBF4 | / | 13.8 mS·cm-1 (50 °C) | 228 mF·cm-2 | 32 μWh·cm-2 | 300% | / | -60~50 | [153] |
Supercapacitor | PVA/P(AM-co-SBMA)/CaCl2 | / | 0.28 S·m-1 (-40 °C) | / | 5.8-5.0 μWh·cm-2 | 530% (60 kPa) | / | -60~50 | [154] |
Supercapacitor | polySH-EG | 5.44 (0%~100%) | 1.84 mS·cm-1 (-30 °C); 25.5 mS·cm-1 (25 °C) | 53.6 F·g-1 (60 °C); 24.2 F·g-1 (-20 °C) | / | 840% (6.5 kPa) | / | -20~60 | [155] |
Supercapacitor | polyAS-EG | / | 1.51 mS·cm-1 (-50 °C) | 93.5 F·g-1 (25 °C); 62.0 F·g-1 (-50 °C) | / | 1,840% (17.8 kPa) | / | -70~60 | [156] |
In summary, zwitterionic hydrogels offer distinct advantages in flexible energy storage devices. They enhance electrolyte conductivity, improve stability, and facilitate the dissociation of ionic complexes, thereby enhancing overall energy storage device performance. However, challenges such as susceptibility to freezing at low temperatures and water loss at high temperatures can affect their effectiveness. To address these issues and meet the application requirements of zwitterionic hydrogels in extreme environments, recent research has focused on developing zwitterionic hydrogels capable of adapting to a wide temperature range, aiming to improve their stability under extreme conditions.
Tissue engineering
Cells are the basis of tissue engineering. Through the culture of cells, biomedical applications such as disease treatment and tissue regeneration can be realized[157]. For example, hematopoietic cell transplantation is a medical treatment for many hematological malignancies, among which cord blood transplantation (CBT) is the most successful treatment. However, the application of CBT is currently limited by the low stem cell doses available[158]. In order to increase the amount of hematopoietic stem and progenitor cells, the super-hydrophilic and anti-biological contamination zwitterionic hydrogel was used as the culture matrix, which was conducive to the expansion of hematopoietic stem cells[159]. Zwitterionic hydrogels have the capability to prevent abnormal production of reactive oxygen species (ROS) both extracellularly and intracellularly. The tertiary amine group of 4-(2-hydroxyethyl)-1-piperazineethanesulfonic acid (HEPES) converts a significant portion of these short half-life ROS into long-lasting H2O2, leading to substantial damage to the cells. However, the zwitterionic betaine-based hydrogels as alternative cytocompatible buffers, their quaternized amine groups, over the tertiary amine groups in HEPES, can completely avoid the ROS production and the severe side effects found on HEPES. The whole process is shown in Figure 11A[160]. The above results also prove that zwitterionic hydrogels can effectively promote wound healing and can be used as wound dressings[161]. In order to further enhance the antioxidant effect on ROS produced during wound repair and the antibacterial effect of zwitterionic hydrogel, the synergistic effect of polydopamine (PDA) with antioxidant effect and Zn2+ with antibacterial effect was used to prepare the zwitterionic hydrogel dressings. So, the zwitterionic hydrogel wound dressing PDA/polySBMA/nanofibrillated cellulose (NFC)/Zn2+ (PSNZn) with excellent antibacterial properties, antioxidant activity, and good biocompatibility was prepared with the participation of NFC. The preparation scheme and experimental results are shown in Figure 11B[162]. MPC polymers have potential applications in many biomedical applications due to their excellent anti-fouling properties and low friction coefficients, such as soft contact lenses and artificial hip joints[163-165]. MPC-based copolymers have become a major synthetic biocompatible material because the MPC layer has high lubrication properties, which can significantly reduce the sliding friction under load. The sliding friction of the MPC layer exceeds that of the physiological system. In addition, the hydration shells surrounding the zwitterionic pendant groups of SB promote hydration lubrication and achieve interfacial bio-adhesion resistance[166-169]. Wang et al. have synthesized a zwitterionic poly(MPC-co-SBMA) copolymer hydrogel through chemical crosslinking[170]. However, the hydrophilicity of SBMA-based materials can still be improved by adding certain surface-active agents[171,172]. This new material design provides a valuable reference for the design and manufacture of synthetic biomaterials for artificial cartilage substitutes. Some studies have indicated that the friction caused by hydrogels is related to the interfacial interaction between the hydrogels and the liquid. The effects of changing the frictional properties of hydrogels by optimizing factors such as load, speed, and temperature have been studied[173,174]. Although many studies[175,176] have reported the mechanical and tribological properties of different hydrogels on frictional pairs, there are few studies focusing on the characteristics of the friction between hydrogels under sliding conditions. So far, researchers have developed hydrogel materials with excellent lubricating properties. However, these hydrogels require high hydration to achieve extremely low friction. Excessive hydration will cause the hydrogel to deform significantly under large contact pressures, which affects its bearing capacity. Therefore, the design of biomimetic synthetic articular cartilage to simultaneously achieve high load-bearing, long abrasion resistance, and low friction characteristics under in vitro physiological conditions such as cartilage replacement is a major scientific challenge. A double-layer hydrogel material shown in Figure 11C was used to mimic natural cartilage and has thick hydrophilic polyelectrolyte brushes entangled in the lower hard layer[27]. This material was obtained using an initiator to graft a hydrophilic polyanionic poly(3-sulfopropyl methacrylate potassium) (polySPMA) or polySBMA brush onto the subsurface of a hard hydrogel to form the double-layer structure. The polySBMA and PSPMA brushes interlocked with the high-strength hydrogel-Br (HHy-Br), resulting in the bilayered hydrogels HHy-g-polySBMA and HHy-g-polySPMA, respectively. The HHy-Br hydrogel layer can withstand high contact pressure. The top layer was composed of a hydrophilic polymer brush, which was covalently entangled below the surface of the hard hydrogel (tens of microns) to achieve a continuous transition from the top hydrated layer to the lower hydrogel without delamination. The top layer showed excellent lubricity due to the bionic design, while the bottom hydrogel helped reduce the contact stress of the entire subsurface. Simultaneously, some researchers have synthesized a nanocomposite hydrogel composed of graphene oxide (GO) nanosheets and polySBMA in an aqueous system through chemical and physical crosslinking[177]. The GO nanosheets were well dispersed in the hydrogel and effectively crosslinked into the SBMA polymer chains through electrostatic interactions. After adding the GO nanosheets, the compressive stress of the polySBMA hydrogel was significantly increased (close to a five-fold increase), and the coefficient of friction (COF) was significantly reduced (52%-76% decrease). These improvements indicated a synergistic interaction and good compatibility between the GO nanosheets and the polySBMA hydrogel matrix, which led to an entangled network structure with a high bearing capacity and excellent lubricating properties. This research provides the potential for developing new graphene-polymer composite materials with high mechanical properties and excellent lubricating properties, which could be beneficial for replacing cartilage.
Figure 11. (A) ROS production in RPMI 1640 mediums associated with HEPES and zwitterionic polybetaines. Reproduced with permission from[160]. Copyright 2023, Elsevier; (B) Zwitterionic composite hydrogel for wound healing. Reproduced with permission from[162]. Copyright 2022, Elsevier; (C) Cartilage mimicking bilayer structure by brushing up stiff hydrogels from subsurface. Reproduced with permission from[27]. Copyright 2020, Wiley-VCH GmbH. SBMA: Sulfobetaine methacrylate; MPC: 2-methacryloyloxyethyl phosphorylcholine; CBMA: carboxybetaine methacrylate; HEPES: 4-(2-hydroxyethyl)-1-piperazineethanesulfonic acid; NFC: nanofibrillated cellulose; PDA: polydopamine; HHy: high-strength hydrogel; ROS: reactive oxygen species; RPMI: Roswell Park Memorial Institute.
However, the soft surface of this hydrogel often has a permanent “water-locking” phenomenon. The hydrogel easily forms a water film on the interface on a small scale. These problems will lead to weak adhesion of the hydrogel in underwater conditions. Researchers[178] have combined flexible hydrogels containing dynamic bonds with biologically inspired surface drainage structures and proposed a method for preparing flexible hydrogels with rapid, reversible, and strong adhesion underwater. Hydrogels prepared by this method can adhere to another solid surface with a small amount of force quickly, and the adhesive strength and peeling energy can reach 25 kPa and 50 J/m2, respectively.
This section delves into the detailed application of zwitterionic hydrogels in tissue engineering. It begins by emphasizing the advantages of superhydrophilic and bio-contamination-resistant zwitterionic hydrogels for cell culture applications. In contrast to conventional cell culture substrates such as HEPES, the zwitterionic hydrogel culture matrix prevents the conversion of ROS to H2O2, which can be detrimental to cells. The zwitterionic hydrogel demonstrates potential applications in hematopoietic stem cell expansion and exhibits positive outcomes in wound healing and dressing. Subsequently, the discussion extends to the use of zwitterionic hydrogels in biomimetic synthesis of articular cartilage, focusing on lubrication and friction properties. Researchers have successfully designed and synthesized artificial cartilage substitutes, creating bilayer hydrogel materials with high load-bearing capacity, extended wear resistance, and low friction characteristics. This development serves as a valuable reference for cartilage replacement. It also points out the shortcomings in the study of friction properties between zwitterionic hydrogels and highlights the challenge of developing novel zwitterionic hydrogels to solve the permanent “water lock” phenomenon.
Multifunctional drug carriers with controlled release
Nanoscale drug carriers are generally composed of polymer materials, which can be used as carriers to carry or transport drugs[179]. When nano-drug delivery systems are introduced into the body, they commonly encounter non-specific adsorption of contaminating proteins, cells, or tissues, leading to phagocytosis by the human immune system. Additionally, steric hindrance often results in inadequate drug intake[180,181]. These problems seriously hinder the therapeutic effect of drugs on diseases. Zwitterionic hydrogel-carriers have excellent resistance to non-specific adsorption of proteins and theoretically can reduce adhesion to Biological pollutants[182,183]. poly (2-methacryloyloxyethyl phosphorylcholine) (PMPC) with the same zwitterionic phosphocholine groups as PC lipids has been shown to have excellent resistance to nonspecific adsorption of proteins and are widely used as drug carriers[172]. Phosphoserine, the head group of a natural lipid, was modified to zwitterionic polymer ZPS (zwitterionic phosphoserine-mimetic polymer) with immunomodulatory function [Figure 12A]. Unlike the non-scaling but biologically inert MPC, ZPS can actively reduce vulnerability to phagocyte clearance and is the first polymer to achieve both non-scaling and immunomodulatory functions. The development of safe and effective biopharmaceuticals can be accelerated[184]. The structure of CBMA is similar to glycine betaine, which is present in the human body. L-asparaginase (ASP) is a highly immunogenic enzyme drug; polyCBMA conjugation to it manifests to significantly promote the diffusion of ASP into the lymphatic system while mitigating its immunogenicity. This overcomes the phenomenon that hydrophobic interaction of PEG with extracellular matrix may attenuate the diffusion of PEGylated drugs in the interstitial space[185]. CBMA and SBMA with good pH stability and thermal response were used to prepare zwitterion photoresist. The photoresist was used for the preparation of micro-medical robots, which can improve the biological hiding performance of the bio-medical robots and avoid the immune clearance of macrophages [Figure 12B]. The service life and work efficiency of satellite medical robots in drug delivery were greatly improved[186]. In addition, due to the nanostructures and zwitterionic structure, polyCB-modified nano-drug carriers can prolong blood circulation time, which will facilitate tumor accumulation through the enhanced permeability and retention (EPR) effect. In the tumor microenvironment, the amide bond of zwitterionic structure will respond to tumor pH (6.5-6.8) and achieve acid-responsive cleavage, resulting in the drug carrier with cationic, which can rapidly enter tumor cells through effective electrostatic interaction with the negatively charged cell membrane. The electrostatic interaction can improve the cellular internalization efficiency of nano-drug carriers, promoting the drug delivery efficiency[187]. Gelatin methacrylate (GelMA) microspheres prepared with a microfluidic emulsion method (denoted as MGS) were modified with a novel copolymer [dopamine methacrylamide (DMA)-SBMA] which had good hydration. Diclofenac sodium (DS) was encapsulated in this hydrogel to form hyper-lubricating MGS@DMA-SBMA@DS, which could be used for the treatment of osteoarthritis. Experimental results demonstrated that the grafting of polySBMA brushes enhanced lubrication, reduced degradation, and sustained drug release of MGS [Figure 12C]. The drug-loaded hyper-lubricated MGS@ (MGS@DMA-SBMA@DS) developed was capable of excellent hydration lubrication and sustained drug release[28]. It has shown great potential in the treatment of osteoarthritis and can also be extended to a variety of other biological systems (ocular surface, pleural cavity, and internal organs).
Figure 12. (A) zwitterionic polymer ZPS (PS-mimetic polymers): design scheme, fibrinogen adsorbed onto different hydrogel surfaces, the status of macrophage evaluated by measuring the production and release of TNF-α. Reproduced with permission from[184]. NS: No significance; *P < 0.05, ***P < 0.001. Data are represented as mean ± SEM. Copyright 2020, American Association for the Advancement of Science; (B) Non-immunogenic stealth microrobots prepared by CBMA and SBMA. Reproduced with permission from[186]. Copyright 2020, Wiley-VCH GmbH; (C) DS-loaded MGS@DMA-SBMA (superlubricated microspheres): schematic illustration, hydration lubrication and sustained drug release, COF-time curve, drug release profile. Reproduced with permission from[28]. Copyright 2020, Wiley-VCH GmbH. MPC: 2-Methacryloyloxyethyl phosphorylcholine; ZPS: zwitterionic phosphoserine-mimetic polymer; DS: diclofenac sodium; DMA: dopamine methacrylamide; SBMA: sulfobetaine methacrylate; CBMA: carboxybetaine methacrylate; COF: coefficient of friction.
This segment provides an overview of the applications of zwitterionic hydrogels in drug delivery. Currently, nano-drug carriers encounter various challenges within drug delivery systems, including the non-specific adsorption of contaminating proteins, cells, or tissues, phagocytosis by the immune system, and limited drug intake due to steric hindrance. Zwitterionic hydrogels, with their remarkable anti-biological contamination ability, can enhance resistance of drug carriers to non-specific proteins. Their utilization in micro-medical robots implanted in vivo helps evade macrophage phagocytosis, thereby extending the service life of medical robots for improved disease diagnosis and treatment. Furthermore, the synergistic effects of superhydrophilicity and drug-loading capabilities of zwitterionic hydrogels can be harnessed to create drug carriers with a super-lubricating effect, offering effective treatment for arthritis.
CHALLENGES AND PROSPECTS
The superhydrophilic properties of zwitterionic hydrogels can block the interference of external biological contaminants and provide reliability for long-term stable operation of biosensors. Compared with other polymers, the existence of ionic structure in zwitterionic hydrogels gives them excellent ionic properties, which is of great significance for improving the sensitivity of biosensors and promoting the storage and release of energy in energy storage devices. In addition, zwitterionic hydrogels as scaffolds and fillings can provide the support required for cell attachment, proliferation and differentiation, and contribute to a suitable physical and biochemical environment for tissue regeneration. They can also achieve precisely controlled drug release by external stimuli such as pH changes, ion concentration regulation, or temperature changes. At present, the applications of zwitterionic hydrogels in the biomedical field have achieved a series of important results and shown great potential. However, although some encouraging progress has been made, a number of challenges and unresolved issues endure. In the following, we summarize the problems that remain to be solved and look forward to the feasible scheme.
Enhance the conductivity of zwitterionic hydrogels
Zwitterionic hydrogels inherently exhibit a certain level of ion conductivity. However, in certain applications, their ion conductivity may be insufficient to meet specific requirements. The simplest approach to enhance the conductivity of zwitterionic hydrogels is by incorporating conductive fillers (such as carbon nanotubes, graphene, metal nanoparticles, etc.)[188-191]. However, these conductive fillers are prone to uneven dispersion in zwitterionic hydrogels, leading to reduced transparency. To improve the conductivity and stability of zwitterionic hydrogels while minimizing the compromise of their other merits, effective and feasible methods include increasing the ion concentration inside the zwitterionic hydrogel, introducing a conductive molecular layer at the specific interface of the zwitterionic hydrogel[192], and incorporating ionic liquids (ILs)[193] are effective and feasible.
Enhance the mechanical property of zwitterionic hydrogels
Due to the substantial water content, low density, and weak polymer chain interactions within the structure, traditional zwitterionic hydrogels exhibit poor mechanical properties that limit their applications. Improving the mechanical properties of these hydrogels can be pursued from three aspects. Firstly, the molecular-scale mechanical properties of hydrogels can be enhanced by introducing nanomaterials such as nanoparticles and nanofibers[194]. Secondly, the stability and durability of zwitterionic hydrogels can be improved by increasing the cross-linking points through multifunctional cross-linking agents, enhancing the connection of molecular networks. Thirdly, superior mechanical properties of zwitterionic hydrogels can be achieved by interweaving the flexible and rigid networks to form a dual network structure[195,196].
Design zwitterionic hydrogels with the excellent mechanical property, anti-fibrosis property, and degradation property
When enhancing the mechanical properties of zwitterionic hydrogels, other properties, such as degradability and anti-fibrosis tendencies, may be compromised. In practical applications, it is necessary to design and optimize the composition and structure of hydrogels to strike a balance between mechanical properties, degradation characteristics, and anti-fibrosis attributes[197]. Two approaches are summarized in this paper. Firstly, we integrate two types of zwitterionic hydrogels into a pure zwitterionic hydrogel with elastomer-like properties. This approach allows the zwitterionic hydrogel to achieve an optimal elastic viscosity ratio without introducing additional substances, enabling it to resist fibrous encapsulation for an extended period[111,198]. Another solution is to introduce disulfide bond cross-linkers into the zwitterionic hydrogel networks, allowing the hydrogel to degrade under reducing conditions[199]. Simultaneously, the disulfide bond-crosslinked zwitterionic hydrogels exhibit the properties of soft conductors, making them applicable to electronic skin for detecting both large and small mechanical deformations, demonstrating sufficient sensitivity and long-term sensing durability.
Improve the precision of drug release during drug delivery
Zwitterionic hydrogels have demonstrated significant potential in the field of drug delivery owing to their responsiveness to temperature and pH. Enhancing the precision of the release process necessitates improvements in the design and preparation methods of zwitterionic hydrogel drug carriers. Firstly, achieving the versatility of zwitterionic hydrogel drug carriers involves preparing multifunctional versions capable of both diagnosis and treatment concurrently, thereby achieving a more comprehensive treatment effect. Secondly, zwitterionic hydrogels, rich in functional groups, are easily modifiable, enabling the introduction of targeted molecules on zwitterionic drug carriers for more accurate drug delivery to specific cells or tissues. Thirdly, the preparation of nanometer-sized zwitterionic nanohydrogel drug carriers can yield a large specific surface area conducive to efficient drug loading and release.
CONCLUSIONS
As a multifunctional biological material, zwitterionic hydrogels exhibit ionic conductivity, anti-biological contamination properties, thermal and pH sensitivity, and an anti-polyelectrolyte effect. They have demonstrated great potential in biosensors, wearable electronic devices, tissue engineering, and drug delivery. This paper provides a detailed summary of their structures, properties, and biomedical applications. Finally, the limitations in the biomedical field are discussed, and corresponding solutions are proposed. In conclusion, these hydrogels have made significant strides in biomedicine. With continuous technological progress and in-depth research, zwitterionic hydrogels are poised to bring about more breakthroughs and innovations in the field of biomedicine in the future.
DECLARATIONS
Authors’ contributions
Conceptualization, writing - original draft, validation, investigation, writing - review and editing: Li C
Writing - review and editing: Zheng H, Zhang X
Writing - review and editing, supervision, funding acquisition: Pu Z
Supervision, funding acquisition: Li D
Availability of data and materials
Not applicable.
Financial support and sponsorship
This work was supported by the National Key R&D Program of China (No. 2022YFB3203700), the National Natural Science Foundation of China (No. 82072012 and No. 82102230), and the 111 Project of China (No. B07014).
Conflict of interest
All authors declared that there are no conflicts of interest.
Ethical approval and consent to participate
Not applicable.
Consent for publication
Not applicable.
Copyright
© The Author(s) 2024.
REFERENCES
1. Yu M, Peng Y, Wang X, Ran F. Emerging design strategies toward developing next-generation implantable batteries and supercapacitors. Adv Funct Mater 2023;33:2301877.
2. Hu X, Ma Z, Li J, et al. Superior water anchoring hydrogel validated by colorimetric sensing. Mater Horiz 2020;7:3250-7.
4. Laschewsky A, Rosenhahn A. Molecular design of zwitterionic polymer interfaces: searching for the difference. Langmuir 2019;35:1056-71.
5. Sakai-Kato K, Kato M, Ishihara K, Toyo’oka T. An enzyme-immobilization method for integration of biofunctions on a microchip using a water-soluble amphiphilic phospholipid polymer having a reacting group. Lab Chip 2004;4:4-6.
6. Morawetz H. Polyelectrolyte solutions: phenomena and interpretation. ACS Symposium Series 2006;937:1-18.
7. Donnio B, Guillon D, Harada A, et al. Supramolecular polymers/polymeric betains/oligomers. Heidelberg: Springer Berlin; 2006.
8. Bonyadi SZ, Demott CJ, Grunlan MA, Dunn AC. Cartilage-like tribological performance of charged double network hydrogels. J Mech Behav Biomed Mater 2021;114:104202.
9. Sun Y, Lu S, Li Q, et al. High strength zwitterionic nano-micelle hydrogels with superior self-healing, adhesive and ion conductive properties. Eur Polym J 2020;133:109761.
10. Qi X, Zhang H, Li Y, Zhang X, Ma H, Zhang L. Nonfouling and antibacterial zwitterionic contact lenses loaded with heme-mimetic gallium porphyrin for treating keratitis. Langmuir 2022;38:14335-44.
11. Decarli NO, Zapp E, de Souza BS, Santana ER, Winiarski JP, Vieira IC. Biosensor based on laccase-halloysite nanotube and imidazolium zwitterionic surfactant for dopamine determination. Biochem Eng J 2022;186:108565.
12. Ladd J, Zhang Z, Chen S, Hower JC, Jiang S. Zwitterionic polymers exhibiting high resistance to nonspecific protein adsorption from human serum and plasma. Biomacromolecules 2008;9:1357-61.
13. Jain P, Hung HC, Li B, et al. Zwitterionic hydrogels based on a degradable disulfide carboxybetaine cross-linker. Langmuir 2019;35:1864-71.
14. Zhang C, Zhou Y, Han H, Zheng H, Xu W, Wang Z. Dopamine-triggered hydrogels with high transparency, self-adhesion, and thermoresponse as skinlike sensors. ACS Nano 2021;15:1785-94.
15. Wang J, Wang L, Wu C, et al. Antibacterial zwitterionic polyelectrolyte hydrogel adhesives with adhesion strength mediated by electrostatic mismatch. ACS Appl Mater Interfaces 2020;12:46816-26.
16. Zhu Y, Zhang J, Song J, et al. A multifunctional pro-healing zwitterionic hydrogel for simultaneous optical monitoring of pH and glucose in diabetic wound treatment. Adv Funct Mater 2020;30:1905493.
17. Yu P, Li Y, Sun H, et al. Mimicking antioxidases and hyaluronan synthase: a zwitterionic nanozyme for photothermal therapy of osteoarthritis. Adv Mater 2023;35:2303299.
18. Yin H, King DR, Sun TL, et al. Polyzwitterions as a versatile building block of tough hydrogels: from polyelectrolyte complex gels to double-network gels. ACS Appl Mater Interfaces 2020;12:50068-76.
19. Sällström N, Capel A, Lewis MP, Engstrøm DS, Martin S. 3D-printable zwitterionic nano-composite hydrogel system for biomedical applications. J Tissue Eng 2020;11:2041731420967294.
20. Wen C, Zhang J, Li Y, et al. A zwitterionic hydrogel coated titanium surface with high-efficiency endothelial cell selectivity for rapid re-endothelialization. Biomater Sci 2020;8:5441-51.
21. Zhao J, Diaz-Dussan D, Wu M, et al. Dual-cross-linked network hydrogels with multiresponsive, self-healing, and shear strengthening properties. Biomacromolecules 2021;22:800-10.
22. Shen W, Zhang Y, Wan P, et al. Antineoplastic drug-free anticancer strategy enabled by host-defense-peptides-mimicking synthetic polypeptides. Adv Mater 2020;32:2001108.
23. Men Y, Peng S, Yang P, et al. Biodegradable zwitterionic nanogels with long circulation for antitumor drug delivery. ACS Appl Mater Interfaces 2018;10:23509-21.
24. Pei X, Zhang H, Zhou Y, Zhou L, Fu J. Stretchable, self-healing and tissue-adhesive zwitterionic hydrogels as strain sensors for wireless monitoring of organ motions. Mater Horiz 2020;7:1872-82.
25. Yang B, Yuan W. Highly stretchable, adhesive, and mechanical zwitterionic nanocomposite hydrogel biomimetic skin. ACS Appl Mater Interfaces 2019;11:40620-8.
26. Wang S, Zhang D, He X, et al. Anti-swelling zwitterionic hydrogels as multi-modal underwater sensors and all-in-one supercapacitors. ACS Appl Polym Mater 2022;4:7498-507.
27. Rong M, Liu H, Scaraggi M, et al. High lubricity meets load capacity: cartilage mimicking bilayer structure by brushing up stiff hydrogels from subsurface. Adv Funct Mater 2020;30:2004062.
28. Yang J, Han Y, Lin J, et al. Ball-bearing-inspired polyampholyte-modified microspheres as bio-lubricants attenuate osteoarthritis. Small 2020;16:2006356.
30. Yuan YY, Mao CQ, Du XJ, Du JZ, Wang F, Wang J. Surface charge switchable nanoparticles based on zwitterionic polymer for enhanced drug delivery to tumor. Adv Mater 2012;24:5476-80.
31. Zheng J, Li M, Yao Y, Zhang X, Wang L. Zwitterionic carbon nanotube assisted thin-film nanocomposite membranes with excellent efficiency for separation of mono/divalent ions from brackish water. J Mater Chem A 2017;5:13730-9.
32. Li G, Xue H, Gao C, Zhang F, Jiang S. Nonfouling polyampholytes from an ion-pair comonomer with biomimetic adhesive groups. Macromolecules 2010;43:14-6.
33. Ehrmann M, Mathis A, Meurer B, Scheer M, Galin JC. Statistical n-butyl acrylate-(sulfopropyl)ammonium betaine copolymers. 2. Structural studies. Macromolecules 1992;25:2253-61.
34. Lowe AB, Billingham NC, Armes SP. Synthesis and aqueous solution properties of novel zwitterionic block copolymers. Chem Commun 1997:1035-6.
35. Baker JP, Blanch HW, Prausnitz JM. Swelling properties of acrylamide-based ampholytic hydrogels: comparison of experiment with theory. Polymer 1995;36:1061-9.
36. Shao Q, Jiang S. Molecular understanding and design of zwitterionic materials. Adv Mater 2015;27:15-26.
37. Chen Y, Han H, Tong H, et al. Zwitterionic phosphorylcholine - TPE conjugate for pH-responsive drug delivery and AIE active imaging. ACS Appl Mater Interfaces 2016;8:21185-92.
38. Liu Z, Wang Y, Ren Y, et al. Poly(ionic liquid) hydrogel-based anti-freezing ionic skin for a soft robotic gripper. Mater Horiz 2020;7:919-27.
39. Jiang S, Cao Z. Ultralow-fouling, functionalizable, and hydrolyzable zwitterionic materials and their derivatives for biological applications. Adv Mater 2010;22:920-32.
40. Zhang Z, Chao T, Chen S, Jiang S. Superlow fouling sulfobetaine and carboxybetaine polymers on glass slides. Langmuir 2006;22:10072-7.
41. Chang Y, Chen S, Yu Q, Zhang Z, Bernards M, Jiang S. Development of biocompatible interpenetrating polymer networks containing a sulfobetaine-based polymer and a segmented polyurethane for protein resistance. Biomacromolecules 2007;8:122-7.
42. Chang Y, Chang WJ, Shih YJ, Wei TC, Hsiue GH. Zwitterionic sulfobetaine-grafted poly(vinylidene fluoride) membrane with highly effective blood compatibility via atmospheric plasma-induced surface copolymerization. ACS Appl Mater Interfaces 2011;3:1228-37.
43. Matsuno R, Ishihara K. Integrated functional nanocolloids covered with artificial cell membranes for biomedical applications. Nano Today 2011;6:61-74.
44. Liu Q, Li W, Wang H, Newby BZ, Cheng F, Liu L. Amino acid-based zwitterionic polymer surfaces highly resist long-term bacterial adhesion. Langmuir 2016;32:7866-74.
45. Li D, Wei Q, Wu C, et al. Superhydrophilicity and strong salt-affinity: zwitterionic polymer grafted surfaces with significant potentials particularly in biological systems. Adv Colloid Interface Sci 2020;278:102141.
46. Deng P, Li X, Wang Y, et al. Highly stretchable ionic and electronic conductive fabric. Adv Fiber Mater 2023;5:198-208.
47. Rose MA, Šmíd B, Vorokhta M, et al. Identifying ionic and electronic charge transfer at oxide heterointerfaces. Adv Mater 2021;33:2004132.
48. McDaniel JG, Yethiraj A. Influence of electronic polarization on the structure of ionic liquids. J Phys Chem Lett 2018;9:4765-70.
49. Jin Z, Kong X, Huang H, et al. Garnet-type solid-state mixed ionic and electronic conductor. Energy Storage Mater 2023;59:102788.
50. Cardoso J, Huanosta A, Manero O. Ionic conductivity studies on salt-polyzwitterion systems. Macromolecules 1991;24:2890-5.
51. Yang J, Xu Z, Wang J, et al. Antifreezing zwitterionic hydrogel electrolytes: antifreezing zwitterionic hydrogel electrolyte with high conductivity of 12.6 mS cm-1 at -40 °C through hydrated lithium ion hopping migration (Adv. Funct. Mater. 18/2021). Adv Funct Mater 2021;31:2170121.
52. Wang L, Gao G, Zhou Y, et al. Tough, adhesive, self-healable, and transparent ionically conductive zwitterionic nanocomposite hydrogels as skin strain sensors. ACS Appl Mater Interfaces 2019;11:3506-15.
53. Tiyapiboonchaiya C, Pringle JM, Sun J, et al. The zwitterion effect in high-conductivity polyelectrolyte materials. Nat Mater 2004;3:29-32.
54. Jin X, Jiang H, Qiao F, et al. Fabrication of alginate-P (SBMA-co-AAm) hydrogels with ultrastretchability, strain sensitivity, self-adhesiveness, biocompatibility, and self-cleaning function for strain sensors. J Appl Polym Sci 2021;138:49697.
55. Gao G, Yang F, Zhou F, et al. Bioinspired self-healing human-machine interactive touch pad with pressure-sensitive adhesiveness on targeted substrates. Adv Mater 2020;32:2004290.
56. Cai N, Li Q, Zhang J, et al. Antifouling zwitterionic hydrogel coating improves hemocompatibility of activated carbon hemoadsorbent. J Colloid Interface Sci 2017;503:168-77.
57. Huang H, Zhang C, Crisci R, et al. Strong surface hydration and salt resistant mechanism of a new nonfouling zwitterionic polymer based on protein stabilizer TMAO. J Am Chem Soc 2021;143:16786-95.
58. Carr L, Cheng G, Xue H, Jiang S. Engineering the polymer backbone to strengthen nonfouling sulfobetaine hydrogels. Langmuir 2010;26:14793-8.
59. Vaisocherová H, Zhang Z, Yang W, et al. Functionalizable surface platform with reduced nonspecific protein adsorption from full blood plasma - material selection and protein immobilization optimization. Biosens Bioelectron 2009;24:1924-30.
60. Liu Y, Zhang D, Ren B, et al. Molecular simulations and understanding of antifouling zwitterionic polymer brushes. J Mater Chem B 2020;8:3814-28.
61. Vales TP, Jee JP, Lee WY, Min I, Cho S, Kim HJ. Protein adsorption and bacterial adhesion resistance of cross-linked copolymer hydrogels based on poly(2-methacryloyloxyethyl phosphorylcholine) and poly(2-hydroxyethyl methacrylate). Bulletin Korean Chem Soc 2020;41:406-12.
62. Ishihara K, Nomura H, Mihara T, Kurita K, Iwasaki Y, Nakabayashi N. Why do phospholipid polymers reduce protein adsorption? J Biomed Mater Res 1998;39:323-30.
63. Zhou L, Lei D, Wang Q, Luo X, Chen Y. Biocompatible polyphosphorylcholine hydrogels with inherent antibacterial and nonfouling behavior effectively promote skin wound healing. ACS Appl Bio Mater 2020;3:5357-66.
64. Zhou L, Lei D, Wang Q, Ouyang Y, Luo X. Rational design of polyphosphorylcholine-based micelles for superior anti-biofilm activity. Macro Mater Eng 2022;307:2100806.
65. Fu F, Wang J, Tan Y, Yu J. Super-hydrophilic zwitterionic polymer surface modification facilitates liquid transportation of microfluidic sweat sensors. Macromol Rapid Commun 2022;43:2100776.
66. Yu X, Liu Z, Janzen J, et al. Polyvalent choline phosphate as a universal biomembrane adhesive. Nat Mater 2012;11:468-76.
68. Nyyssölä A. Pathways of glycine betaine synthesis in two extremely halophilic bacteria, actinopolyspora halophila and ectothiorhodospira halochloris. Available from: https://aaltodoc.aalto.fi/items/5b13c16e-8c5c-4589-a16b-2667cb8a7015. [Last accessed on 23 Feb 2024]
69. Vaisocherová H, Yang W, Zhang Z, et al. Ultralow fouling and functionalizable surface chemistry based on a zwitterionic polymer enabling sensitive and specific protein detection in undiluted blood plasma. Anal Chem 2008;80:7894-901.
70. Mi L, Bernards MT, Cheng G, Yu Q, Jiang S. pH responsive properties of non-fouling mixed-charge polymer brushes based on quaternary amine and carboxylic acid monomers. Biomaterials 2010;31:2919-25.
71. West SL, Salvage JP, Lobb EJ, et al. The biocompatibility of crosslinkable copolymer coatings containing sulfobetaines and phosphobetaines. Biomaterials 2004;25:1195-204.
72. Fang K, Wang R, Zhang H, et al. Mechano-responsive, tough, and antibacterial zwitterionic hydrogels with controllable drug release for wound healing applications. ACS Appl Mater Interfaces 2020;12:52307-18.
73. Zhang Z, Vaisocherová H, Cheng G, Yang W, Xue H, Jiang S. Nonfouling behavior of polycarboxybetaine-grafted surfaces: structural and environmental effects. Biomacromolecules 2008;9:2686-92.
74. Zhu Y, Batchelor R, Lowe AB, Roth PJ. Design of thermoresponsive polymers with aqueous LCST, UCST, or both: modification of a reactive poly(2-vinyl-4,4-dimethylazlactone) scaffold. Macromolecules 2016;49:672-80.
75. Li Z, Hao B, Tang Y, et al. Effect of end-groups on sulfobetaine homopolymers with the tunable upper critical solution temperature (UCST). Eur Polym J 2020;132:109704.
76. Lewoczko EM, Wang N, Lundberg CE, et al. Effects of N-substituents on the solution behavior of poly(sulfobetaine methacrylate)s in water: upper and lower critical solution temperature transitions. ACS Appl Polym Mater 2021;3:867-78.
77. Hildebrand V, Laschewsky A, Wischerhoff E. Modulating the solubility of zwitterionic poly((3-methacrylamidopropyl)ammonioalkane sulfonate)s in water and aqueous salt solutions via the spacer group separating the cationic and the anionic moieties. Polym Chem 2016;7:731-40.
78. Saha P, Santi M, Frenken M, et al. Dual-temperature-responsive microgels from a zwitterionic functional graft copolymer with superior protein repelling property. ACS Macro Lett 2020;9:895-901.
79. Tamaki M, Kojima C. pH-switchable LCST/UCST-type thermosensitive behaviors of phenylalanine-modified zwitterionic dendrimers. RSC Adv 2020;10:10452-60.
80. Quan X, Zhao D, Li L, Zhou J. Understanding the cellular uptake of pH-responsive zwitterionic gold nanoparticles: a computer simulation study. Langmuir 2017;33:14480-9.
81. Zhou Y, Chen Z, Zhao D, Li D, He C, Chen X. A pH-triggered self-unpacking capsule containing zwitterionic hydrogel-coated MOF nanoparticles for efficient oral exendin-4 delivery. Adv Mater 2021;33:2102044.
82. Zhang Y, Liao J, Wang T, Sun W, Tong Z. Polyampholyte hydrogels with pH modulated shape memory and spontaneous actuation. Adv Funct Mater 2018;28:1707245.
83. Wang T, Wang X, Long Y, Liu G, Zhang G. Ion-specific conformational behavior of polyzwitterionic brushes: exploiting it for protein adsorption/desorption control. Langmuir 2013;29:6588-96.
84. Mccormick CL, Johnson CB. Water-soluble polymers. 28. Ampholytic copolymers of sodium 2-acrylamido-2-methylpropanesulfonate with (2-acrylamido-2-methylpropyl)dimethylammonium chloride: synthesis and characterization. Macromolecules 1988;21:686-93.
85. Han X, Leng C, Shao Q, Jiang S, Chen Z. Absolute orientations of water molecules at zwitterionic polymer interfaces and interfacial dynamics after salt exposure. Langmuir 2019;35:1327-34.
86. Zheng SY, Zhou J, Si M, et al. A molecularly engineered zwitterionic hydrogel with strengthened anti-polyelectrolyte effect: from high-rate solar desalination to efficient electricity generation. Adv Funct Mater 2023;33:2303272.
87. Li X, Luo F, Sun TL, et al. Effect of salt on dynamic mechanical behaviors of polyampholyte hydrogels. Macromolecules 2023;56:535-44.
88. Fang Y, Huang S, Gong X, et al. Salt sensitive purely zwitterionic physical hydrogel for prevention of postoperative tissue adhesion. Acta Biomater 2023;158:239-51.
89. Yu X, Liu J, Xin Y, et al. Temperature and salt responsive zwitterionic polysulfamide-based nanogels with surface regeneration ability and controlled drug release. Polym Chem 2019;10:6423-31.
90. Shao Q, Mi L, Han X, et al. Differences in cationic and anionic charge densities dictate zwitterionic associations and stimuli responses. J Phys Chem B 2014;118:6956-62.
91. Sabu C, Henna TK, Raphey VR, Nivitha KP, Pramod K. Advanced biosensors for glucose and insulin. Biosens Bioelectron 2019;141:111201.
92. Erathodiyil N, Chan HM, Wu H, Ying JY. Zwitterionic polymers and hydrogels for antibiofouling applications in implantable devices. Mater Today 2020;38:84-98.
93. Huang KT, Hsieh PS, Dai LG, Huang CJ. Complete zwitterionic double network hydrogels with great toughness and resistance against foreign body reaction and thrombus. J Mater Chem B 2020;8:7390-402.
94. Rebelo R, Barbosa AI, Correlo VM, Reis RL. An outlook on implantable biosensors for personalized medicine. Engineering 2021;7:1696-9.
95. Dave KM, Han L, Jackson MA, Kadlecik L, Duvall CL, Manickam DS. DNA polyplexes of a phosphorylcholine-based zwitterionic polymer for gene delivery. Pharm Res 2020;37:176.
96. Xu Z, Han R, Liu N, Gao F, Luo X. Electrochemical biosensors for the detection of carcinoembryonic antigen with low fouling and high sensitivity based on copolymerized polydopamine and zwitterionic polymer. Sensor Actuat B Chem 2020;319:128253.
97. Lu S, Fu B, Zhang Z. Zwitterionic polymers coating antibiofouling photoelectrochemical aptasensor for in vivo antibiotic metabolism monitoring and tracking. Anal Chem 2022;94:14509-16.
98. Keefe AJ, Jiang S. Poly(zwitterionic)protein conjugates offer increased stability without sacrificing binding affinity or bioactivity. Nat Chem 2011;4:59-63.
99. Zhu Y, Song J, Zhang J, et al. Encapsulation of laccase within zwitterionic poly-carboxybetaine hydrogels for improved activity and stability. Catal Sci Technol 2018;8:5217-24.
100. Erfani A, Zarrintaj P, Seaberg J, Ramsey JD, Aichele CP. Zwitterionic poly(carboxybetaine) microgels for enzyme (chymotrypsin) covalent immobilization with extended stability and activity. J Appl Polym Sci 2021;138:50545.
101. Meyers SR, Grinstaff MW. Biocompatible and bioactive surface modifications for prolonged in vivo efficacy. Chem Rev 2012;112:1615-32.
102. Hawkins ML, Schott SS, Grigoryan B, et al. Anti-protein and anti-bacterial behavior of amphiphilic silicones. Polym Chem 2017;8:5239-51.
103. Sung HJ, Luk A, Murthy NS, et al. Poly(ethylene glycol) as a sensitive regulator of cell survival fate on polymeric biomaterials: the interplay of cell adhesion and pro-oxidant signaling mechanisms. Soft Matter 2010;6:5196-205.
104. Lee DU, Kayumov M, Park J, et al. Antibiofilm and antithrombotic hydrogel coating based on superhydrophilic zwitterionic carboxymethyl chitosan for blood-contacting devices. Bioact Mater 2024;34:112-24.
105. Yao M, Sun H, Guo Z, et al. A starch-based zwitterionic hydrogel coating for blood-contacting devices with durability and bio-functionality. Chem Eng J 2021;421:129702.
106. Du Q, Wang W, Zeng X, Luo X. Antifouling zwitterionic peptide hydrogel based electrochemical biosensor for reliable detection of prostate specific antigen in human serum. Anal Chim Acta 2023;1239:340674.
107. Wu H, Lee CJ, Wang H, et al. Highly sensitive and stable zwitterionic poly(sulfobetaine-3,4-ethylenedioxythiophene) (PSBEDOT) glucose biosensor. Chem Sci 2018;9:2540-6.
108. Xie X, Doloff JC, Yesilyurt V, et al. Reduction of measurement noise in a continuous glucose monitor by coating the sensor with a zwitterionic polymer. Nat Biomed Eng 2018;2:894-906.
109. Li X, Tang C, Liu D, et al. High-strength and nonfouling zwitterionic triple-network hydrogel in saline environments. Adv Mater 2021;33:2102479.
110. Yao M, Wei Z, Li J, et al. Microgel reinforced zwitterionic hydrogel coating for blood-contacting biomedical devices. Nat Commun 2022;13:5339.
111. Dong D, Tsao C, Hung HC, et al. High-strength and fibrous capsule-resistant zwitterionic elastomers. Sci Adv 2021;7:eabc5442.
112. Zhang W, Wu B, Sun S, Wu P. Skin-like mechanoresponsive self-healing ionic elastomer from supramolecular zwitterionic network. Nat Commun 2021;12:4082.
113. Sun Y, Wang Y, Liu Y, Wu S, Zhang S, Niu W. Biomimetic chromotropic photonic-ionic skin with robust resilience, adhesion, and stability. Adv Funct Mater 2022;32:2204467.
114. Xu S, Yu JX, Guo H, et al. Force-induced ion generation in zwitterionic hydrogels for a sensitive silent-speech sensor. Nat Commun 2023;14:219.
115. Xu T, Zhang L, Song B, et al. High-strain sensitive zwitterionic hydrogels with swelling-resistant and controllable rehydration for sustainable wearable sensor. J Colloid Interface Sci 2022;620:14-23.
116. Zhang D, Tang Y, Zhang Y, et al. Highly stretchable, self-adhesive, biocompatible, conductive hydrogels as fully polymeric strain sensors. J Mater Chem A 2020;8:20474-85.
117. Huang Y, Xiao L, Zhou J, et al. Strong tough polyampholyte hydrogels via the synergistic effect of ionic and metal-ligand bonds. Adv Funct Mater 2021;31:2103917.
118. Bai J, Wang R, Wang X, et al. Biomineral calcium-ion-mediated conductive hydrogels with high stretchability and self-adhesiveness for sensitive iontronic sensors. Cell Rep Phys Sci 2021;2:100623. Available from: https://www.sciencedirect.com/science/article/pii/S2666386421003416. [Last accessed on 23 Feb 2024]
119. Wang H, Li X, Ji Y, et al. Highly transparent, mechanical, and self-adhesive zwitterionic conductive hydrogels with polyurethane as a cross-linker for wireless strain sensors. J Mater Chem B 2022;10:2933-43.
120. Huang H, Han L, Fu X, et al. Multiple stimuli responsive and identifiable zwitterionic ionic conductive hydrogel for bionic electronic skin. Adv Elect Mater 2020;6:2000239.
121. Guo H, Bai M, Wen C, et al. A zwitterionic-aromatic motif-based ionic skin for highly biocompatible and glucose-responsive sensor. J Colloid Interface Sci 2021;600:561-71.
122. Guo H, Bai M, Zhu Y, et al. Pro-healing zwitterionic skin sensor enables multi-indicator distinction and continuous real-time monitoring. Adv Funct Mater 2021;31:2106406.
123. Lee DU, Kim SC, Choi DY, Jung WK, Moon MJ. Basic amino acid-mediated cationic amphiphilic surfaces for antimicrobial pH monitoring sensor with wound healing effects. Biomater Res 2023;27:14.
124. Li C, Zhang K, Cheng X, et al. Polymers for flexible energy storage devices. Prog Polym Sci 2023;143:101714.
125. Zhao F, Bae J, Zhou X, Guo Y, Yu G. Nanostructured functional hydrogels as an emerging platform for advanced energy technologies. Adv Mater 2018;30:1801796.
126. Wang Z, Yao S, Wang S, et al. Self-powered energy harvesting and implantable storage system based on hydrogel-enabled all-solid-state supercapacitor and triboelectric nanogenerator. Chem Eng J 2023;463:142427.
127. Dutta B, Ray SK. Synthesis of copolymer nanocomposite by in situ intercalative polymerization for batch and fixed bed adsorption. Polym Eng Sci 2023;63:2578-95.
128. Qiu M, Liu H, Tawiah B, Jia H, Fu S. Zwitterionic triple-network hydrogel electrolyte for advanced flexible zinc ion batteries. Compos Commun 2021;28:100942.
129. Lee JH, Yeon JS, Kim J, et al. Accelerated Li-ion transport through a zwitterion-anchored separator for high-performance Li-S batteries. J Mater Chem A 2021;9:25463-73.
130. Lee JH, Shin JC, Kim J, et al. Zwitterionic surfactant-stabilized ionogel electrolytes with high ionic conductivity for lithium secondary batteries. J Power Sources 2023;557:232565.
131. Leng K, Li G, Guo J, et al. A safe polyzwitterionic hydrogel electrolyte for long-life quasi-solid state zinc metal batteries. Adv Funct Mater 2020;30:2001317.
132. Khalil H, Bhat A, Ireana Yusra A. Green composites from sustainable cellulose nanofibrils: a review. Carbohyd Polym 2012;87:963-79.
133. Mo F, Chen Z, Liang G, et al. Zwitterionic sulfobetaine hydrogel electrolyte building separated positive/negative ion migration channels for aqueous Zn-MnO2 batteries with superior rate capabilities. Adv Energy Mater 2020;10:2000035.
134. Li C, Yang S, Guo Y, et al. Hydrogel electrolyte with high tolerance to a wide spectrum of pHs and compressive energy storage devices based on it. Small Methods 2023;7:e2201448.
135. Peng X, Liu H, Yin Q, et al. A zwitterionic gel electrolyte for efficient solid-state supercapacitors. Nat Commun 2016;7:11782.
136. Amiri A, Bruno A, Polycarpou AA. Configuration-dependent stretchable all-solid-state supercapacitors and hybrid supercapacitors. Carbon Energy 2023;5:e320.
137. Zhang Z, Gao Y, Gao Y, Jia F, Gao G. A self-adhesive, self-healing zwitterionic hydrogel electrolyte for high-voltage zinc-ion hybrid supercapacitors. Chem Eng J 2023;452:139014.
138. Wei J, Zhou J, Su S, Jiang J, Feng J, Wang Q. Water-deactivated polyelectrolyte hydrogel electrolytes for flexible high-voltage supercapacitors. ChemSusChem 2018;11:3410-5.
139. Han L, Huang H, Fu X, et al. A flexible, high-voltage and safe zwitterionic natural polymer hydrogel electrolyte for high-energy-density zinc-ion hybrid supercapacitor. Chem Eng J 2020;392:123733.
140. Guo WY, Mai T, Huang LZ, et al. Multifunctional MXene conductive zwitterionic hydrogel for flexible wearable sensors and arrays. ACS Appl Mater Interfaces 2023;15:24933-47.
141. Chen Y, Zhang C, Yin R, et al. Ultra-robust, high-adhesive, self-healing, and photothermal zwitterionic hydrogels for multi-sensory applications and solar-driven evaporation. Mater Horiz 2023;10:3807-20.
142. Tang L, Wu S, Li Y, et al. A super-tough ionic conductive hydrogel with anti-freezing, water retention, and self-regenerated properties for self-powered flexible sensor. Appl Mater Today 2023;32:101820.
143. Sui X, Guo H, Cai C, et al. Ionic conductive hydrogels with long-lasting antifreezing, water retention and self-regeneration abilities. Chem Eng J 2021;419:129478.
144. Jiao Q, Cao L, Zhao Z, Zhang H, Li J, Wei Y. Zwitterionic hydrogel with high transparency, ultrastretchability, and remarkable freezing resistance for wearable strain sensors. Biomacromolecules 2021;22:1220-30.
145. Zhang Y, Li T, Miao L, et al. A highly sensitive and ultra-stretchable zwitterionic liquid hydrogel-based sensor as anti-freezing ionic skin. J Mater Chem A 2022;10:3970-88.
146. Lan J, Zhou B, Yin C, Weng L, Ni W, Shi L. Zwitterionic dual-network strategy for highly stretchable and transparent ionic conductor. Polymer 2021;231:124111.
147. Cao L, Zhao Z, Li J, Yi Y, Wei Y. Gelatin-reinforced zwitterionic organohydrogel with tough, self-adhesive, long-term moisturizing and antifreezing properties for wearable electronics. Biomacromolecules 2022;23:1278-90.
148. Hu R, Yang X, Cui W, et al. An ultrahighly stretchable and recyclable starch-based gel with multiple functions. Adv Mater 2023;35:2303632.
149. Fu Q, Hao S, Zhang X, Zhao H, Xu F, Yang J. All-round supramolecular zwitterionic hydrogel electrolytes enabling environmentally adaptive dendrite-free aqueous zinc ion capacitors. Energy Environ Sci 2023;16:1291-311.
150. Xiao S, He X, Zhao Z, et al. Strong anti-polyelectrolyte zwitterionic hydrogels with superior self-recovery, tunable surface friction, conductivity, and antifreezing properties. Eur Polym J 2021;148:110350.
151. Zhang G, Yang X, Shu H, Zhong W. Ultrahigh conductivity and antifreezing zwitterionic sulfobetaine hydrogel electrolyte for low-temperature resistance flexible supercapacitors. J Mater Chem A 2023;11:9097-111.
152. Fu Q, Hao S, Meng L, Xu F, Yang J. Engineering self-adhesive polyzwitterionic hydrogel electrolytes for flexible zinc-ion hybrid capacitors with superior low-temperature adaptability. ACS Nano 2021;15:18469-82.
153. Wang S, Zhang D, He X, et al. Polyzwitterionic double-network ionogel electrolytes for supercapacitors with cryogenic-effective stability. Chem Eng J 2022;438:135607.
154. Hu O, Lu J, Weng S, Hou L, Zhang X, Jiang X. An adhesive, anti-freezing, and environment stable zwitterionic organohydrogel for flexible all-solid-state supercapacitor. Polymer 2022;254:125109.
155. Sun W, Yang J, Ji X, et al. Antifreezing zwitterionic hydrogel electrolyte with high conductivity at subzero temperature for flexible sensor and supercapacitor. Sustain Mater Technol 2022;32:e00437.
156. Sun W, Xu Z, Qiao C, et al. Antifreezing proton zwitterionic hydrogel electrolyte via ionic hopping and grotthuss transport mechanism toward solid supercapacitor working at -50 °C. Adv Sci 2022;9:e2201679.
157. Lu X, Xu G, Lin Z, et al. Engineered exosomes enriched in netrin-1 modRNA promote axonal growth in spinal cord injury by attenuating inflammation and pyroptosis. Biomater Res 2023;27:3.
158. Ballen KK, Gluckman E, Broxmeyer HE. Umbilical cord blood transplantation: the first 25 years and beyond. Blood 2013;122:491-8.
159. Bai T, Li J, Sinclair A, et al. Expansion of primitive human hematopoietic stem cells by culture in a zwitterionic hydrogel. Nat Med 2019;25:1566-75.
160. Liu P, Sun J, Peng W, et al. Zwitterionic betaines over HEPES as the new generation biocompatible pH buffers for cell culture. Bioact Mater 2023;24:376-86.
161. Xiao Z, Zheng X, An Y, et al. Zwitterionic hydrogel for sustained release of growth factors to enhance wound healing. Biomater Sci 2021;9:882-91.
162. Wang S, Liu R, Bi S, et al. Mussel-inspired adhesive zwitterionic composite hydrogel with antioxidant and antibacterial properties for wound healing. Colloid Surface B 2022;220:112914.
163. Chang Y, Shu SH, Shih YJ, Chu CW, Ruaan RC, Chen WY. Hemocompatible mixed-charge copolymer brushes of pseudozwitterionic surfaces resistant to nonspecific plasma protein fouling. Langmuir 2010;26:3522-30.
164. Chang Y, Mallika Arjunan M, N’guérékata G, Kavitha V. On global solutions to fractional functional differential equations with infinite delay in Fréchet spaces. Comput Math Appl 2011;62:1228-37.
165. Yu Y, Vancso GJ, de Beer S. Substantially enhanced stability against degrafting of zwitterionic PMPC brushes by utilizing PGMA-linked initiators. Eur Polym J 2017;89:221-9.
166. Chen M, Briscoe WH, Armes SP, Klein J. Lubrication at physiological pressures by polyzwitterionic brushes. Science 2009;323:1698-701.
167. Hayes WC, Bodine AJ. Flow-independent viscoelastic properties of articular cartilage matrix. J Biomech 1978;11:407-19.
168. Charalambides MN, Goh SM, Wanigasooriya L, Williams JG, Xiao W. Effect of friction on uniaxial compression of bread dough. J Mater Sci 2005;40:3375-81.
169. Cai M, Yu Q, Zhou F, Liu W. Physicochemistry aspects on frictional interfaces. Friction 2017;5:361-82.
170. Wang Z, Li J, Liu Y, Luo J. Synthesis and characterizations of zwitterionic copolymer hydrogels with excellent lubrication behavior. Tribol Int 2020;143:106026.
171. Zhang Z, Chao T, Liu L, Cheng G, Ratner BD, Jiang S. Zwitterionic hydrogels: an in vivo implantation study. J Biomater Sci Polym Ed 2009;20:1845-59.
172. Zhang K, Yang J, Sun Y, et al. Gelatin-based composite hydrogels with biomimetic lubrication and sustained drug release. Friction 2022;10:232-46.
173. Galante R, Ghisleni D, Paradiso P, et al. Sterilization of silicone-based hydrogels for biomedical application using ozone gas: Comparison with conventional techniques. Mater Sci Eng C Mater Biol Appl 2017;78:389-97.
174. Willis SL, Court JL, Redman RP, et al. A novel phosphorylcholine-coated contact lens for extended wear use. Biomaterials 2001;22:3261-72.
175. Zhang D, Chen K, Wu L, Wang D, Ge S. Synthesis and characterization of PVA-HA-silk composite hydrogel by orthogonal experiment. J Bionic Eng 2012;9:234-42.
176. Ma R, Xiong D, Miao F, Zhang J, Peng Y. Novel PVP/PVA hydrogels for articular cartilage replacement. Mater Sci Eng C 2009;29:1979-83.
177. Wang Z, Li J, Jiang L, Xiao S, Liu Y, Luo J. Zwitterionic hydrogel incorporated graphene oxide nanosheets with improved strength and lubricity. Langmuir 2019;35:11452-62.
178. Rao P, Sun TL, Chen L, et al. Tough hydrogels with fast, strong, and reversible underwater adhesion based on a multiscale design. Adv Mater 2018;30:e1801884.
179. Sun H, Yan L, Zhang R, Lovell JF, Wu Y, Cheng C. A sulfobetaine zwitterionic polymer-drug conjugate for multivalent paclitaxel and gemcitabine co-delivery. Biomater Sci 2021;9:5000-10.
180. Dobrovolskaia MA, Aggarwal P, Hall JB, McNeil SE. Preclinical studies to understand nanoparticle interaction with the immune system and its potential effects on nanoparticle biodistribution. Mol Pharm 2008;5:487-95.
181. Gafur A, Kristi N, Maruf A, Wang G, Ye Z. Transforming stealthy to sticky nanocarriers: a potential application for tumor therapy. Biomater Sci 2019;7:3581-93.
182. Yang F, Xu L, Kuang D, Ge Y, Guo G, Wang Y. Polyzwitterion-crosslinked hybrid tissue with antithrombogenicity, endothelialization, anticalcification properties. Chem Eng J 2021;410:128244.
183. Lin W, Kluzek M, Iuster N, et al. Cartilage-inspired, lipid-based boundary-lubricated hydrogels. Science 2020;370:335-8.
184. Li B, Yuan Z, Jain P, et al. De novo design of functional zwitterionic biomimetic material for immunomodulation. Sci Adv 2020;6:eaba0754.
185. Li B, Yuan Z, He Y, Hung HC, Jiang S. Zwitterionic nanoconjugate enables safe and efficient lymphatic drug delivery. Nano Lett 2020;20:4693-9.
186. Cabanach P, Pena-Francesch A, Sheehan D, et al. Zwitterionic 3D-printed non-immunogenic stealth microrobots. Adv Mater 2020;32:2003013.
187. Wang S, Zhang F, Yu G, et al. Zwitterionic-to-cationic charge conversion polyprodrug nanomedicine for enhanced drug delivery. Theranostics 2020;10:6629-37.
188. Yang W, Ella-Menye JR, Liu S, et al. Cross-linked carboxybetaine SAMs enable nanoparticles with remarkable stability in complex media. Langmuir 2014;30:2522-9.
189. Liu C, Faria AF, Ma J, Elimelech M. Mitigation of biofilm development on thin-film composite membranes functionalized with zwitterionic polymers and silver nanoparticles. Environ Sci Technol 2017;51:182-91.
190. Huang KT, Fang YL, Hsieh PS, Li CC, Dai NT, Huang CJ. Zwitterionic nanocomposite hydrogels as effective wound dressings. J Mater Chem B 2016;4:4206-15.
191. Zhang C, Sun W, Wang Y, et al. Gd-/CuS-loaded functional nanogels for MR/PA imaging-guided tumor-targeted photothermal therapy. ACS Appl Mater Interfaces 2020;12:9107-17.
192. Xue H, Wang D, Jin M, et al. Hydrogel electrodes with conductive and substrate-adhesive layers for noninvasive long-term EEG acquisition. Microsyst Nanoeng 2023;9:79.
193. Sankar Sivasankarapillai V, Sundararajan A, Chonnur Easwaran E, et al. Application of ionic liquids in rubber elastomers: perspectives and challenges. J Mol Liq 2023;382:121846.
194. Lin S, Liu J, Liu X, Zhao X. Muscle-like fatigue-resistant hydrogels by mechanical training. Proc Natl Acad Sci U S A 2019;116:10244-9.
195. Na Y, Kurokawa T, Katsuyama Y, et al. Structural characteristics of double network gels with extremely high mechanical strength. Macromolecules 2004;37:5370-4.
196. Diao W, Wu L, Ma X, et al. Highly stretchable, ionic conductive and self-recoverable zwitterionic polyelectrolyte-based hydrogels by introducing multiple supramolecular sacrificial bonds in double network. J Appl Polym Sci 2019;136:47783.
197. Mou X, Miao W, Zhang W, et al. Zwitterionic polymers-armored amyloid-like protein surface combats thrombosis and biofouling. Bioact Mater 2024;32:37-51.
198. Ren J, Liu Y, Wang Z, et al. An anti-swellable hydrogel strain sensor for underwater motion detection. Adv Funct Mater 2022;32:2107404.
Cite This Article

How to Cite
Download Citation
Export Citation File:
Type of Import
Tips on Downloading Citation
Citation Manager File Format
Type of Import
Direct Import: When the Direct Import option is selected (the default state), a dialogue box will give you the option to Save or Open the downloaded citation data. Choosing Open will either launch your citation manager or give you a choice of applications with which to use the metadata. The Save option saves the file locally for later use.
Indirect Import: When the Indirect Import option is selected, the metadata is displayed and may be copied and pasted as needed.
About This Article
Copyright
Author Biographies
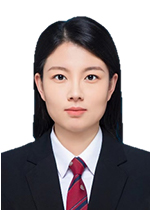
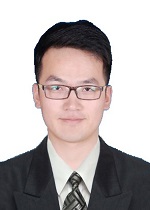
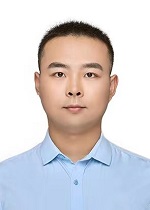
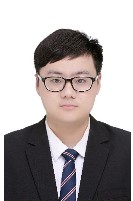
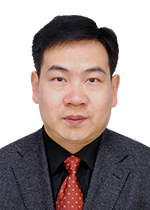
Comments
Comments must be written in English. Spam, offensive content, impersonation, and private information will not be permitted. If any comment is reported and identified as inappropriate content by OAE staff, the comment will be removed without notice. If you have any queries or need any help, please contact us at [email protected].