Immobilized enzymatic alcohol oxidation as a versatile reaction module for multienzyme cascades
Abstract
Enzymatic alcohol oxidation (EAO) is highly attractive thanks to its efficiency, selectivity, and sustainability benefits, but it is often neglected as a catalytic tool for practical production due to the instability and non-reusability of enzymes. Herein, a non-enantioselective alcohol dehydrogenase engineered from Candida parapsilosis (CpsADH) and a laccase from Trametes versicolor was immobilized on mesoporous silica nanoflowers (MSNs), fabricating CpsADH@MSNs (41 U/gsupport) and laccase@MSNs (67 U/gsupport) for EAO, respectively. The structural and functional properties of the MSNs endowed the immobilized enzymes with higher stability than free enzymes, and the relative activity of the immobilized enzyme was 52% and 63%, respectively, after being reused five times. The immobilized enzymes exhibited high activity, selectivity, and complementary substrate specificity in alcohol oxidation. The optimized EAO, as a versatile cascade module, was coupled with several other enzymatic transformations for multi-enzymatic synthesis of high value-added chemicals. The chiral alcohols and amines were produced with 99% ee and 84% to 98% ee, respectively, and (R)-benzoin and 2-furoic acid were prepared with 91% yield, 99% ee and 86% yield, respectively, demonstrating the synthetic utility of the immobilized enzymes.
Keywords
INTRODUCTION
Selective oxidation of alcohols to corresponding carbonyl compounds, such as aldehydes, ketones, and carboxylic acids, has become one of the most important chemical transformations in organic chemistry and chemical industries[1]. Various approaches have been developed for this fundamental reaction. Conventional methods typically required stoichiometric toxic oxidants such as sodium hypochlorite, potassium permanganate, potassium dichromate, and organic peroxides[2-4], resulting in high cost and environmental problems[5-7]. Nowadays, catalytic oxidation approaches enable the utilization of environmentally more acceptable oxidants such as molecular oxygen (O2). Among them, metal-catalyzed aerobic oxidation of alcohols has attracted considerable attention[8-13]. Particularly, the heterogeneous catalysis is more preferred by industrial production due to its advantages of high recyclability, low toxicity, and simple protocol[14-16]. Although significant improvements have been made, the metal-catalyzed systems usually suffer from harsh reaction conditions (high temperatures and volatile organic solvents), metal residues, and low selectivity. In addition, O2 cannot be used on a large scale due to the tendency to form explosive mixtures. Therefore, it is still a critical task to develop metal-free catalytic systems for the green, mild, selective, and safe oxidation of alcohols.
From an environmental and safety perspective, enzymatic alcohol oxidation (EAO) is more attractive due to its mild reaction conditions, high selectivity, and eco-friendliness[17]. Various enzymes are capable of supporting this task, for instance, the most widely used alcohol dehydrogenases (ADHs), which utilize the oxidized nicotinamide cofactor [NAD(P)+] as a hydride acceptor[18]. Although the complete oxidation of primary alcohols to aldehydes by ADHs has been extensively explored[19], the similar transformation from racemic secondary alcohols towards prochiral ketones is generally problematic because only one of the enantiomers can be oxidized. To solve this issue, two enantiocomplementary ADHs are usually applied[20], albeit with a more complicated reaction scheme. Non-enantioselective ADHs are ideal biocatalysts for the complete oxidation of racemic alcohols but remain highly scarce. Recently, a non-enantioselective ADH engineered from Candida parapsilosis (CpsADH) with high specificity toward secondary benzyl alcohols has been reported[20]. Besides ADHs, complete oxidation of racemic sec-alcohols (e.g., activated benzylic, allylic, and propargylic alcohols) by laccase has also been reported, where the organocatalyst 2,2’,6,6’-tetramethylpiperidine-N-oxyl (TEMPO) is commonly used as the oxidant[21-24], although it is generally reported to selectively oxidize primary alcohols. In addition, EAO has proven to be a versatile cascade module that can be coupled with a broad of enzymatic transformations for multi-enzymatic production of high-value chemicals[25]. Despite this, enzymes are often neglected as catalytic tools for practical production due to their instability and non-reusability[26,27].
Immobilization of enzymes on suitable support has been proven to be effective in improving their stability, reusability, and even activity[28-30]. The nature of the support materials has a significant influence on the catalytic performance of enzymes. Mesoporous silica materials have emerged as an attractive immobilization platform due to their distinct structural and functional properties, including high surface area, high stability, high pore accessibility, high permeability, high biocompatibility, and easily functionalized groups[28,31,32]. Particularly, mesoporous silica nanoflowers (MSNs) have demonstrated superiority in immobilizing various enzymes such as lipases[33,34], transaminases[35], and ene-reductases[36,37]. However, to the best of our knowledge, MSN-based immobilized enzymes for alcohol oxidation have not been reported yet. Herein, we have fabricated two MSN-based immobilized enzymes, i.e., the non-enantioselective CpsADH (CpsADH@MSNs) and laccase (laccase@MSNs), for the efficient and complete oxidation of racemic alcohols. Compared to free enzymes, MSN-based biocatalysts achieved significant improvements in pH, thermal, mechanical, and storage stability. The CpsADH@MSNs exhibited high activity and specificity toward sec-alcohols, while the laccase@MSNs showed high catalytic performance in the full oxidation of both prim- and sec-alcohols. The synthetic utility of the immobilized enzymes and the EAO was demonstrated in several multienzyme cascades for the synthesis of high value-added synthons.
EXPERIMENTAL
Materials
Tetraethyl orthosilicate (TEOS), sodium acetate, ethanol, glutaraldehyde (GA), cyclohexane, n-butyl alcohol, and urea of analytical grade were purchased from Tianjin Chemical. Additionally,
Preparation of CpsADH@MSNs and laccase@MSNs
For CpsADH immobilization, 50 mg of MSNs activated under different conditions were uniformly dispersed in 5 mL PBS (50 mM, pH = 8.5) by ultrasound for 30 min. Then, 5 mL of CpsADH solution
For laccase immobilization, 50 mg of MSNs activated under different conditions were uniformly dispersed in 5 mL acetate buffer solution (pH = 4.5, 0.1 M) by ultrasound for 30 min. Then, 5 mL of laccase solution (2-16 mgenzyme powder/mL) was added, and the mixture was shaken at 170 rpm for different times to explore the optimum GA concentration, covalent bonding time immobilized time, and laccase concentration.
Oxidation of alcohols by CpsADH@MSNs or laccase@MSNs
The oxidation of alcohols (20 mM) by CpsADH@MSNs (10 mg) was performed in 1 mL PBS buffer (50 mM, pH = 8.5) containing NAD+ (0.2 mM) and acetone (200 mM). After the reaction at 40 °C and 180 rpm for 6 h, the catalyst was separated by centrifugation; the reaction product was extracted by ethyl acetate, and the organic phase was dried with anhydrous Na2SO4. The solvent was concentrated in a vacuum to obtain crude products. The product was purified by column chromatography (petroleum ether/ethyl acetate = 8/1, v/v).
The oxidation of alcohols (20 mM) by laccase@MSNs (10 mg) was performed with laccase@MSNs in 1 mL acetate buffer solution (100 mM, pH = 4.5) containing TEMPO (12 mM). After the reaction at 40 °C and 180 rpm for 24 h, the catalyst was separated by centrifugation, the reaction product was extracted by ethyl acetate, and the organic phase was dried with anhydrous Na2SO4. The solvent was concentrated in a vacuum to obtain crude products. The product was purified by column chromatography (petroleum ether/ethyl acetate = 8/1, v/v).
Bienzymatic deracemization of alcohols
The substrate (20 mM) was oxidized with CpsADH@MSNs (10 mg) in 1 mL PBS buffer (50 mM, pH = 8.5) containing NAD+ (0.2 mM) and acetone (200 mM). After the reaction at 40 °C and 180 rpm for 12 h, the catalyst was separated by centrifugation. The acetone was removed by heating at 60 °C, and the pH was adjusted to 9.5. Then 1 mL ADH-A (from Rhodococcus ruber)[38] solution and isopropanol (30% v/v) were added. After the reaction at 30 °C for 24 h, the reaction solution was extracted by ethyl acetatethe reaction solution was extracted by ethyl acetate. The yields and ee values were calculated by gas chromatography.
Bienzymatic hydrogen-borrowing alcohol amination
The substrate (20 mM), NAD+ (0.2 mM), CpsADH@MSNs (10 mg) were added to the 5 mL round-bottomed flask containing 1 mL NH4Cl/NH4OH buffer (2 M, pH = 9.0) and 10 μL DMSO. Subsequently, 1 mL Ja-AmDH (from Jeotgalicoccus aerolatus)[39] crude enzyme solution was added. The mixture was stirred at 30 °C for 48 h. After the reaction was complete, the catalyst was separated by centrifugation. The product was extracted with diethyl ether (5 mL), and the organic phase was dried with anhydrous Na2SO4. The solvent was concentrated in a vacuum to obtain a crude product. The product was purified by column chromatography (ethyl acetate/methanol = 99/1, v/v).
Bienzymatic carboligation
Benzyl alcohol (20 mM) was oxidized with laccase@MSNs (10 mg) in 1 mL sodium acetate buffer (100 mM, pH = 4.5) containing TEMPO (12 mM). After the reaction at 40 °C and 180 rpm for 24 h, the catalyst was separated by centrifugation. Then, pH was adjusted to 7.5 with hydrochloric acid, and MgSO4 (2.5 mM), ThDP (0.15 mM), and 1 mL of benzaldehyde lyase (BAL) (from Pseudomonas fluorescens Biovar I)[40] solution were added. After the reaction at 30 °C for 24 h, the reaction solution was extracted by ethyl acetate, and the organic phase was dried with anhydrous Na2SO4. The solvent was concentrated in a vacuum to obtain a crude product. The product was purified by column chromatography (petroleum ether/ethyl acetate = 5/1, v/v).
Biocatalytic relay oxidation
Furfuryl alcohol (20 mM) was oxidized with laccase@MSNs (10 mg) in 1 mL sodium acetate buffer (100 mM, pH = 4.5) containing TEMPO (12 mM). After the reaction at 40 °C and 180 rpm for 24 h, the catalyst was separated by centrifugation, and the pH of the reaction solution was adjusted to 7. Then, 14 mg of whole cell catalysts (dry weight) over-expressing horse liver ADH (HLADH) (from Equus caballus)[41] were added. After the reaction at 30 °C for 96 h, the reaction solution was extracted by ethyl acetate, and the organic phase was dried with anhydrous Na2SO4. The solvent was concentrated in a vacuum to obtain a crude product. The product was purified by column chromatography (petroleum ether/ethyl acetate = 2/1, v/v).
RESULTS AND DISCUSSION
Preparation and characterization of the immobilized enzymes
Wrinkled MSNs were prepared by a modified reverse microemulsion method[38] and then functionalized with an amino (-NH2) group, providing reaction sites for covalent immobilization of enzymes. The morphology and pore structures of the MSNs were characterized by SEM and TEM. As shown in Supplementary Figure 1A, the MSNs were flower-like nanospheres with an average diameter of 390 nm, and they have a large specific surface area due to their small size and surface folds. The TEM images of the MSNs demonstrated the distinctive fibrous center-radial channels [Supplementary Figure 1B], which can facilitate the mass transfer. The specific surface area and pore diameter were calculated based on N2 adsorption-desorption isotherms and pore size distributions [Supplementary Figure 2], which are
Although the enzymes for complete oxidation of sec-alcohols, especially benzyl alcohols to ketones, are highly constrained, non-enantioselective CpsADH and laccase have been reported to achieve this goal and were, therefore, chosen as the model enzymes. The enzymes were covalently bound to the MSNs using GA as a crosslinking agent. Immobilized enzymes were prepared using FITC-labeled CpsADH and Rhodamine B-labeled laccase for the confocal laser scanning microscope (CLSM) analysis. The green fluorescence of immobilized CpsADH [Figure 1A] and the red fluorescence of immobilized laccase [Figure 1B] can be clearly observed, evidencing the successful immobilization of the enzymes. In FT-IR spectra, a strong band at 1,630 cm−1 appeared, which was attributed to the presence of the C=N bond formed between GA and enzymes [Figure 1C]. In addition, the absorption peaks at 1,394 and 1,540 cm−1 were assigned to the stretching vibration of C-N bonds and N-H bending vibration, respectively[42-44]. These results further confirmed the successful enzyme immobilization.
Figure 1. CLSM images of CpsADH@MSNs (A) and laccase@MSNs (B); Fourier transform infrared spectroscopy (FT-IR) spectra of MSNs-GA, CpsADH@MSNs, and laccase@MSNs (C).
The effectiveness of enzyme immobilization was dependent on the GA concentration, covalent bonding time, enzyme concentration, and immobilization time. The relationship between the relative enzyme activity and GA concentration was shown in Figure 2A and B. With the increase of GA concentration, the relative activities of CpsADH@MSNs and laccase@MSNs increased gradually due to the greater immobilization of enzyme molecules on the support by formation of Schiff bases between GA and enzymes[45,46]. However, too high GA concentrations also caused decreased relative enzyme activity because the excess GA caused enzyme conformation change and enzyme self-crosslinking, which led to a steric hindrance effect on enzyme immobilization[47,48]. The maximum relative activity of CpsADH@MSNs and laccase@MSNs were obtained with the use of 3 wt% and 5 wt% GA, respectively.
Figure 2. Effects of GA concentration on the relative activity (RA) of immobilized enzyme and adsorption capacity (AC) of MSNs: CpsADH@MSNs (A); laccase@MSNs (B); Effects of covalent bonding time on the relative activity (RA) of immobilized enzyme and adsorption capacity (AC) of MSNs: CpsADH@MSNs (C); laccase@MSNs (D).
The crosslinking time of GA and MSNs also has a significant effect on immobilization efficiency. As depicted in Figure 2C and D, the optimal activity of CpsADH@MSNs and laccase@MSNs were obtained after crosslinking for 3 h and 4 h, respectively. With the increase of covalent bonding time, the enzyme attachment sites on the nanoparticles increased, resulting in an increase in the amount of protein fixation and the activity of immobilized enzymes. The spatial grid structure of the support increased over time, restricting the access of more enzyme molecules[49]. Hence, an appropriate covalent bonding time was essential to maintain high enzyme activity. The effects of immobilization time and enzyme concentration on the relative activity of immobilized enzymes were shown in Figure 3A-D. With the increase of fixation time and enzyme concentration, the specific enzyme activity and protein load of the fixed enzyme tended to be stable. As shown in Supplementary Figure 3A, the immobilization yield and enzyme activity recovery of CpsADH@MSNs were measured. The enzyme activity recovery of CpsADH@MSNs decreased gradually with the increase of protein concentration, and the immobilization yield reached the maximum of 47.9% at 3 mg/mL. However, since the protein content of the laccase powder used was too low to accurately measure the immobilization yield, only the recovery of enzyme activity from laccase immobilization was tested. The enzyme activity recovery of laccase@MSNs peaked at 27.8% at
Figure 3. Effects of immobilization time on the specific activity (SA) and relative activity (RA) of immobilized enzyme and adsorption capacity (AC) of MSNs: CpsADH@MSNs (A); laccase@MSNs (B); Effects of protein concentration on the specific activity (SA) and relative activity (RA) of immobilized enzyme and adsorption capacity (AC) of MSNs: CpsADH@MSNs (C); laccase@MSNs (D).
Enzymatic properties of free and immobilized enzymes
A series of parameters, such as temperature, pH, storage time, and numbers for recycling, were studied to evaluate the stability of the immobilized enzymes. Both the free CpsADH and CpsADH@MSNs showed the optimal activity at 30 °C [Figure 4A]. The activity of free CpsADH decreased significantly no matter whether the temperature was below nor above 30 °C, while the CpsADH@MSNs exhibited high stability in a wide temperature range. Free CpsADH and CpsADH@MSNs showed the highest activity at pH = 8.5 [Figure 4B]. When the pH value was below or above 8.5, the activity of free CpsADH decreased significantly, while the CpsADH@MSNs showed high stability in a wide pH range. The optimum temperature and pH of free and immobilized laccase were confirmed to be 45 °C and 4.5, respectively. Similarly, the laccase@MSNs also exhibited higher stability than the free laccase [Figure 4C and D].
Figure 4. Optimal temperature of free CpsADH and CpsADH@MSNs (A); and free laccase and laccase@MSNs (C); Optimal pH of free CpsADH and CpsADH@MSNs (B); and free laccase and laccase@MSNs (D).
We also investigated the activity variation of free and immobilized enzymes after the incubation at 30 °C
Figure 5. Thermal stability of the free enzyme and immobilized enzyme at 30 °C: CpsADH@MSNs (A); laccase@MSNs (B); Thermal stability of the free enzyme and immobilized enzyme at 50 °C: CpsADH@MSNs (C); laccase@MSNs (D).
Catalytic performance of the immobilized enzymes
Having obtained and characterized the CpsADH@MSNs and laccase@MSNs, we turned our attention to investigating their catalytic performance using 1-phenylethanol as a model substrate. After the optimization of reaction conditions, pH = 8.5 and 40 °C were selected to be the optimal condition for CpsADH@MSNs
In a CpsADH@MSN catalytic system, a co-substrate method was applied to achieve cofactor regeneration, which is important for improving economic benefit. Using acetone as a co-substrate, the dosage of the cofactor (NAD+) could be reduced to 0.01 equivalent, and the reaction conversion was still up to 99%, albeit with increased reaction time [Supplementary Table 1, Entries 6-9]. According to the literature[54], the catalytic mechanism of laccase/TEMPO systems was proposed. First, laccase oxidizes the stable oxyl-radical form of TEMPO to the oxoammonium ion (the actual oxidant species), which can oxidize the alcohol to ketone (or aldehyde) through a hydrogen abstraction process while generating hydroxylamine (TEMP-OH). The generated TEMP-OH was then re-oxidized to TEMPO radical. Theoretically, only a catalytic amount of TEMPO can support this transformation, which was confirmed by experimental results. However, a low amount of TEMPO led to an extremely low reaction rate. For example, only 24% conversion was obtained after reacting 24 h if only using 0.2 equivalent of TEMPO. Increasing to TEMPO equivalent to 0.6, the conversion increased to 99% during the same reaction time [Supplementary Table 2, Entries 1-3]. Higher substrate concentrations were also tested for these two immobilized enzymes. The complete conversion can still be obtained by CpsADH@MSNs after reacting 36 h with a 100 mM scale, and laccase@MSNs gave 91% conversion after reacting four days with a 200 mM scale. We then examined the operational stability of the CpsADH@MSNs and laccase@MSNs using the catalytic oxidation of 1-phenylethanol as the model reaction. As shown in Supplementary Figure 4, CpsADN@MSNs still have good catalytic performance with relative activity of 52% after five cycles, and laccase@MSNs maintained 63% relative activity after five cycles. These results highlighted the potential of the immobilized enzymes for practical applications. Due to the fact that only negligible enzyme leakage (0.5% for CpsADH@MSNs) was detected after five reuse cycles
To demonstrate the general applicability of the immobilized enzymes, we explored their substrate scope under the optimized reaction conditions. As shown in Scheme 1, the substrate portfolio could be expanded to both electron-deficient and electron-rich secondary alcohols, and all gave high yields (91%-99%). In a CpsADH@MSNs catalytic system, a substituent effect of substrates on reaction rates was observed, but this phenomenon was not found for laccase@MSNs. Generally, the reaction rates of the ortho-, meta-, and the para-substituted substrates increased in turn. For example, the complete oxidation of ortho-, meta-, and the para-substituted alcohols required 24, 12, and 6 h, respectively. Both the CpsADH@MSNs and laccase@MSNs showed high activity and selectivity toward (hetero)aryl-alkyl alcohols (1-19b) and alkyl-alkyl alcohol (20b), except for laccase@MSNs towards 18b and 19b. No obvious substrate/product inhibition was found, and other reasons for the low activity of laccase@MSNs towards these two compounds are not clear. Laccase@MSNs also exhibited high activity in the oxidation of aryl alcohols
To further demonstrate the synthetic utility of the EAO, we investigated its application in multienzyme cascades for the enantioselective synthesis of high value-added synthons, such as chiral alcohols and amines[17]. The conversion of racemic alcohols to enantiopure alcohols and amines is of great importance in pharmaceutical manufacturing[55] because racemic alcohols are commercially available and easily accessible, and these enantiopure compounds are often useful and valuable pharmaceutical intermediates. Coupling non-enantioselective alcohol oxidation with highly enantioselective ketone reduction, an oxidation-reduction cascade can be successfully constructed to achieve deracemization of alcohols, converting racemic alcohols to their enantiopure counterparts[56,57]. By combining CpsADH@MSNs with ADH-A, a series of enantiopure α-benzyl alcohols were obtained in 91%-97% yields and 98%-99% ee from racemic alcohols [Scheme 2A]. The redox-neutral hydrogen-borrowing cascade catalyzed by ADHs and amine dehydrogenases (AmDHs) has become an elegant approach for synthesizing chiral amines from racemic alcohols, in which the ADH-catalyzed alcohol oxidation is accompanied by the consumption of NADH and generation of NAD+, while the AmDH-catalyzed ketone reductive amination leads to the conversion of NAD+ to NADH, creating a cofactor self-regeneration system[58,59]. Combining CpsADH@MSNs with an engineered Ja-AmDH, two pharmaceutically relevant chiral α-aryl primary amines were synthesized in high yields and enantioselectivity [Scheme 2B].
Enantioselective C-C bond-forming reactions have become a powerful tool for the synthesis of enantiopure compounds from readily available non-chiral substrates, such as the benzoin reaction. In this regard, a bienzymatic cascade combing laccase@MSNs and BAL was developed for the enantioselective synthesis of
CONCLUSIONS
In conclusion, the immobilization of CpsADH and laccase on MSNs was achieved for EAO. The specific enzyme activities of the optimized CpsADH@MSNs and laccase@MSNs were 41 and 67 U/gsupport, respectively. The immobilized enzymes showed higher stability than the free enzymes and exhibited high catalytic activity and selectivity in oxidation of various alcohols under mild conditions. As a versatile cascade module, the EAO was coupled with several other enzymatic transformations, achieving multienzyme cascades for alcohol deracemization, hydrogen-borrowing alcohol amination, enantioselective C-C bond formation, and relay oxidation of biomass-derived furfuryl alcohol. This work highlighted the application potential of the immobilized enzymes and EAO in synthetic chemistry.
DECLARATIONS
Acknowledgments
We sincerely thank all the team members who participated in this study.
Authors’ contributions
Carried out the catalyst preparation, characterization, and catalytic tests and prepared the draft manuscript: Fu K
Carried out the MSN preparation: Dong L, Liu P
Performed the TEM, SEM, and FT-IR characterization: Zhou L, Liu G, Gao J, Gao B
Planned the study, analyzed the data, and wrote the manuscript: Liu Y, Jiang Y
Availability of data and materials
Supplementary Materials are available online for this paper, and the data supporting the findings of this study are available within its supplementary materials.
Financial support and sponsorship
This research was supported by the National Key Research and Development Program of China (2021YFC2104100); The National Natural Science Foundation of China (22378096, 22078081, and 22178083); The Natural Science Foundation of Hebei Province (B2022202014 and B2020202021); The S&T Program of Hebei (21372805D, 21372804D, and 20372802D); The Natural Science Foundation of Tianjin (20JCYBJC00530); And the Science Technology Research Project of Higher Education of Hebei Province (QN2021045).
Conflicts of interest
All authors declared that there are no conflicts of interest.
Ethical approval and consent to participate
Not applicable.
Consent for publication
Not applicable.
Copyright
© The Author(s) 2024.
Supplementary Materials
REFERENCES
1. Asthana M, Syiemlieh I, Kumar A, Lal RA. Direct oxidation of alcohols catalysed by heterometallic complex [CuNi(bz)3(bpy)2] ClO4 to aldehydes and ketones mediated by hydrogen peroxide as a terminal oxidant. Inorganica Chimica Acta 2020;502:119286.
2. Shaabani A, Shaabani S, Afaridoun H. Highly selective aerobic oxidation of alkyl arenes and alcohols: cobalt supported on natural hydroxyapatite nanocrystals. RSC Adv 2016;6:48396-404.
3. Mallat T, Baiker A. Oxidation of alcohols with molecular oxygen on solid catalysts. Chem Rev 2004;104:3037-58.
4. Nakagawa K, Konaka R, Nakata T. Oxidation with Nickel Peroxide. I. oxidation of alcohols. J Org Chem 1962;27:1597-601.
5. Lou JD, Xu ZN. Selective oxidation of primary alcohols with chromium trioxide under solvent free conditions. Tetrahedron Lett 2002;43:6095-7.
7. Taylor RJ, Reid M, Foot J, Raw SA. Tandem oxidation processes using manganese dioxide: discovery, applications, and current studies. Acc Chem Res 2005;38:851-69.
8. Hasanpour B, Jafarpour M, Feizpour F, Rezaeifard A. Copper(II)-ethanolamine triazine complex on chitosan-functionalized nanomaghemite for catalytic aerobic oxidation of benzylic alcohols. Catal Lett 2021;151:45-55.
9. Martı́n SE, Suárez DF. Catalytic aerobic oxidation of alcohols by Fe(NO3)3-FeBr3. Tetrahedron Lett 2002;43:4475-9.
10. Qiu S, Li Y, Xu H, et al. Efficient catalytic oxidation of benzyl alcohol by tetrasubstituted cobalt phthalocyanine-MWCNTs composites. Solid State Sci 2022;129:106905.
11. Schultz MJ, Hamilton SS, Jensen DR, Sigman MS. Development and comparison of the substrate scope of Pd-catalysts for the aerobic oxidation of alcohols. J Org Chem 2005;70:3343-52.
12. Personick ML, Zugic B, Biener MM, Biener J, Madix RJ, Friend CM. Ozone-activated nanoporous gold: a stable and storable material for catalytic oxidation. ACS Catal 2015;5:4237-41.
13. Parmeggiani C, Cardona F. Transition metal based catalysts in the aerobic oxidation of alcohols. Green Chem 2012;14:547-64.
15. Shibuya M, Tomizawa M, Suzuki I, Iwabuchi Y. 2-azaadamantane N-oxyl (AZADO) and 1-Me-AZADO: highly efficient organocatalysts for oxidation of alcohols. J Am Chem Soc 2006;128:8412-3.
16. Punniyamurthy T, Velusamy S, Iqbal J. Recent advances in transition metal catalyzed oxidation of organic substrates with molecular oxygen. Chem Rev 2005;105:2329-63.
17. Liu J, Wu S, Li Z. Recent advances in enzymatic oxidation of alcohols. Curr Opin Chem Biol 2018;43:77-86.
18. Moa S, Himo F. Quantum chemical study of mechanism and stereoselectivity of secondary alcohol dehydrogenase. J Inorg Biochem 2017;175:259-66.
19. Kroutil W, Mang H, Edegger K, Faber K. Biocatalytic oxidation of primary and secondary alcohols. Adv Synth Catal 2004;346:125-42.
20. Tian K, Li Z. A simple biosystem for the high-yielding cascade conversion of racemic alcohols to enantiopure amines. Angew Chem Int Ed Engl 2020;59:21745-51.
21. Albarrán-velo J, Gotor-fernández V, Lavandera I. One-pot two-step chemoenzymatic deracemization of allylic alcohols using laccases and alcohol dehydrogenases. Mol Catal 2020;493:111087.
22. González-granda S, Méndez-sánchez D, Lavandera I, Gotor-fernández V. Laccase-mediated oxidations of propargylic alcohols. Application in the deracemization of 1-arylprop-2-yn-1-ols in combination with alcohol dehydrogenases. ChemCatChem 2020;12:520-7.
23. Martínez-montero L, Gotor V, Gotor-fernández V, Lavandera I. Stereoselective amination of racemic sec-alcohols through sequential application of laccases and transaminases. Green Chem 2017;19:474-80.
24. Méndez-sánchez D, Mangas-sánchez J, Lavandera I, Gotor V, Gotor-fernández V. Chemoenzymatic deracemization of secondary alcohols by using a TEMPO-Iodine-alcohol dehydrogenase system. ChemCatChem 2015;7:4016-20.
25. de Gonzalo G, Paul CE. Recent trends in synthetic enzymatic cascades promoted by alcohol dehydrogenases. Curr Opin Green Sust 2021;32:100548.
26. Schoemaker HE, Mink D, Wubbolts MG. Dispelling the myths--biocatalysis in industrial synthesis. Science 2003;299:1694-7.
27. Hartmann M, Kostrov X. Immobilization of enzymes on porous silicas--benefits and challenges. Chem Soc Rev 2013;42:6277-89.
28. Gao J, Kong W, Zhou L, et al. Monodisperse core-shell magnetic organosilica nanoflowers with radial wrinkle for lipase immobilization. Chem Eng J 2017;309:70-9.
29. Deng J, Wang H, Zhan H, et al. Catalyzed degradation of polycyclic aromatic hydrocarbons by recoverable magnetic chitosan immobilized laccase from Trametes versicolor. Chemosphere 2022;301:134753.
30. Zheng F, Cui BK, Wu XJ, Meng G, Liu HX, Si J. Immobilization of laccase onto chitosan beads to enhance its capability to degrade synthetic dyes. Int Biodeter Biodegr 2016;10:69-78.
31. Gao J, Wang Y, Du Y, et al. Construction of biocatalytic colloidosome using lipase-containing dendritic mesoporous silica nanospheres for enhanced enzyme catalysis. Chem Eng J 2017;317:175-86.
32. Videira-quintela D, Martin O, Montalvo G. Emerging opportunities of silica-based materials within the food industry. Microchem J 2021;167:106318.
33. Costantini A, Califano V. Lipase immobilization in mesoporous silica nanoparticles for biofuel production. Catalysts 2021;11:629.
34. Kalantari M, Yu M, Yang Y, et al. Tailoring mesoporous-silica nanoparticles for robust immobilization of lipase and biocatalysis. Nano Res 2017;10:605-17.
35. Cao G, Gao J, Zhou L, et al. Fabrication of Ni2+-nitrilotriacetic acid functionalized magnetic mesoporous silica nanoflowers for one pot purification and immobilization of His-tagged ω-transaminase. BioChem Eng J 2017;128:116-25.
36. Zhou L, Ouyang Y, Kong W, et al. One pot purification and co-immobilization of His-tagged old yellow enzyme and glucose dehydrogenase for asymmetric hydrogenation. Enzyme Microb Technol 2022;156:110001.
37. Li Y, Luan P, Dong L, et al. Asymmetric reduction of conjugated C=C bonds by immobilized fusion of old yellow enzyme and glucose dehydrogenase. Green Synth Catal 2022:online ahead of print.
38. Gao L, Wang Z, Liu Y, et al. Co-immobilization of metal and enzyme into hydrophobic nanopores for highly improved chemoenzymatic asymmetric synthesis. Chem Commun 2020;56:13547-50.
39. Kong W, Liu Y, Huang C, et al. Direct asymmetric reductive amination of alkyl (hetero)aryl ketones by an engineered amine dehydrogenase. Angewandte Chemie 2022;134:e202202264.
40. Demir A, Şeşenoglu Ö, Eren E, et al. Enantioselective synthesis of α-Hydroxy ketones via benzaldehyde lyase-catalyzed C−C bond formation reaction. Adv Synth Catal 2002;344:96-103.
41. Peng B, Ma CL, Zhang PQ, et al. An effective hybrid strategy for converting rice straw to furoic acid by tandem catalysis via Sn-sepiolite combined with recombinant E. coli whole cells harboring horse liver alcohol dehydrogenase. Green Chem 2019;21:5914-23.
42. Ghannadi S, Abdizadeh H, Miroliaei M, Saboury AA. Immobilization of alcohol dehydrogenase on titania nanoparticles to enhance enzyme stability and remove substrate inhibition in the reaction of formaldehyde to methanol. Ind Eng Chem Res 2019;58:9844-54.
43. Esmaeilnejad-ahranjani P, Kazemeini M, Singh G, Arpanaei A. Amine-functionalized magnetic nanocomposite particles for efficient immobilization of lipase: effects of functional molecule size on properties of the immobilized lipase. RSC Adv 2015;5:33313-27.
44. Zang L, Qiu J, Wu X, Zhang W, Sakai E, Wei Y. Preparation of magnetic chitosan nanoparticles as support for cellulase immobilization. Ind Eng Chem Res 2014;53:3448-54.
45. Barbosa O, Ortiz C, Berenguer-murcia Á, Torres R, Rodrigues RC, Fernandez-lafuente R. Glutaraldehyde in bio-catalysts design: a useful crosslinker and a versatile tool in enzyme immobilization. RSC Adv 2014;4:1583-600.
46. Songulashvili G, Jimenéz-Tobón GA, Jaspers C, Penninckx MJ. Immobilized laccase of Cerrena unicolor for elimination of endocrine disruptor micropollutants. Fungal Biol 2012;116:883-9.
47. Zhang J, Xu Z, Chen H, Zong Y. Removal of 2,4-dichlorophenol by chitosan-immobilized laccase from Coriolus versicolor. BioChem Eng J 2009;45:54-9.
48. Sathishkumar P, Kamala-kannan S, Cho M, et al. Laccase immobilization on cellulose nanofiber: the catalytic efficiency and recyclic application for simulated dye effluent treatment. J Mol Catal B-Enzym 2014;100:111-20.
49. Kašpar O, Tokárová V, Nyanhongo GS, Gübitz G, Štěpánek F. Effect of cross-linking method on the activity of spray-dried chitosan microparticles with immobilized laccase. Food Bioprod Process 2013;91:525-33.
50. Li X, Zhu H, Feng J, et al. One-pot polylol synthesis of graphene decorated with size- and density-tunable Fe3O4 nanoparticles for porcine pancreatic lipase immobilization. Carbon 2013;60:488-97.
51. Ida J, Matsuyama T, Yamamoto H. Immobilization of glucoamylase on ceramic membrane surfaces modified with a new method of treatment utilizing SPCP-CVD. Biochem Eng J 2000;5:179-84.
52. Grahame DAS, Bryksa BC, Yada RY. Improving and tailoring enzymes for food quality and functionality. Woodhead Publishing; 2015.pp.11-55.
53. Frankenberger W, Johanson J. Effect of pH on enzyme stability in soils. Soil Biol Biochem 1982;14:433-7.
54. Puetz H, Puchľová E, Vranková K, Hollmann F. Biocatalytic oxidation of alcohols. Catalysts 2020;10:952.
55. Lalonde J. Highly engineered biocatalysts for efficient small molecule pharmaceutical synthesis. Curr Opin Biotechnol 2016;42:152-8.
56. Paul CE, Lavandera I, Gotor‐Fernández V, Kroutil W, Gotor V. Escherichia coli /ADH-A: an all-inclusive catalyst for the selective biooxidation and deracemisation of secondary alcohols. ChemCatChem 2013;5:3875-81.
57. Musa MM, Hollmann F, Mutti FG. Synthesis of enantiomerically pure alcohols and amines via biocatalytic deracemisation methods. Catal Sci Technol 2019;9:5487-503.
58. Mutti FG, Knaus T, Scrutton NS, Breuer M, Turner NJ. Conversion of alcohols to enantiopure amines through dual-enzyme hydrogen-borrowing cascades. Science 2015;349:1525-9.
Cite This Article

How to Cite
Download Citation
Export Citation File:
Type of Import
Tips on Downloading Citation
Citation Manager File Format
Type of Import
Direct Import: When the Direct Import option is selected (the default state), a dialogue box will give you the option to Save or Open the downloaded citation data. Choosing Open will either launch your citation manager or give you a choice of applications with which to use the metadata. The Save option saves the file locally for later use.
Indirect Import: When the Indirect Import option is selected, the metadata is displayed and may be copied and pasted as needed.
About This Article
Copyright
Data & Comments
Data
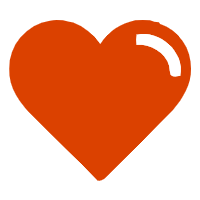
Comments
Comments must be written in English. Spam, offensive content, impersonation, and private information will not be permitted. If any comment is reported and identified as inappropriate content by OAE staff, the comment will be removed without notice. If you have any queries or need any help, please contact us at [email protected].