The synthesis, characterization and application of the binol-cages of R-/S-enantiomers
Abstract
In the past few years, significant efforts have been made to create and self-assemble covalent organic cages with increased complexity and functionality. However, although supramolecule cages have been widely recognized as probes to identify metal ions, the detection of mercury ions has not been fully developed. Here, we have designed and synthesized a pair of chiral cages with custom cavities based on the unique rigid structure of 1, 10-binaphthol (binol). Meanwhile, the supramolecular cage has excellent performance in high sensitivity and selectivity for detecting mercury ions. The UV titration results indicate that the binding ratio of the host to guest is 1:5. The titration curve conforms to the nonlinear fitting of the Hill function, which can obtain the binding constant K =
Keywords
INTRODUCTION
The global attention on environmental pollution caused by heavy metal ions persists due to their potential to inflict severe harm on both animals and humans[1]. Many highly sensitive and selective probes have been developed and successfully used in practical life in order to detect metal ions in the environment. For example, probes synthesized by Mohandoss et al. were widely used for detecting Fe3+, Al3+, Cu2+, and Ag+ ions[2-4]. As is well known, mercury is extremely harmful to organisms and the environment, especially to mammals. It can not only enter the human body directly through the skin, digestive system, or respiratory system but also accumulate in the environment, ultimately entering the human body through the food chain and causing serious damage to the central nervous and endocrine systems of the human body. In addition, all stable forms of Hg (i.e., metallic, inorganic, and organic) cause neurological poisoning in fish, wildlife, and humans. It causes several disorders in the liver, kidney, heart, stomach, brain, intestine, and immune system[5-7]. According to the United States Environmental Protection Agency (US EPA), the maximum possible limit of Hg2+ ions in drinking water is 2 ppb, but the amount of Hg2+ ions in drinking water excess to the limit may pose a risk to human health and the environment (Environmental Protection Agency, 2001). Despite these drawbacks, mercury and mercury salts still widely exist in nature in the form of elemental mercury, inorganic, and organic compounds. Human activities, industrial production, and natural phenomena can all lead to the production of various forms of mercury compounds[8-10]. Therefore, it is necessary to seek efficient, simple, and fast new ways to detect mercury ions in the atmosphere and wastewater in order to protect the Earth's environment[11-25].
In recent years, mercury ions have been detected by a large number of analytical methods, including cold vapor atomic fluorescence spectroscopy[26], inductively coupled plasma mass spectrometry (ICP-MS)[27], gas chromatography[28], neutron activation analysis (NAA)[29], and high-performance liquid chromatography[30]. However, these technologies often require cumbersome operations and expensive instruments. As a result, the development of more efficient and facile approaches for mercury ion detection is highly appealing yet challenging.
Supramolecular chemistry, as an interesting field, has successively attracted much attention due to its unique structures and extensive applications in sensing[31,32], catalysis[33,34], separation[35,36], gas adsorption[37,38], biological imitation[39], and so on[40,41]. The intriguing three-dimensional molecular cages have proven to be superior alternative architectures, garnering considerable interest owing to their outstanding structures, properties, applications, and the diverse array of charming skeletons successfully generated via coordinative self-assembly, such as octahedrons[42,43], tetrahedrons[44-47], spirals[48], capsules[49], etc. Additionally, subcomponent self-assembly, as the most powerful bottom-up strategy, has attracted great attention by preparing highly organized structures with different functions in an "order-out-of-chaos" and well-controlled manner[50-53]. Comparatively, the discrete supramolecular structures formed by covalent bonds remain underdeveloped, possibly due to the dynamic and unstable interactions[54,55]. In this context, molecular cages formed by imine condensation have developed rapidly[56-58] since the groundbreaking work of Quan et al. in 1991[59]. Despite the impressive progress made by chemists in this field, the involvement of multiple functional components in a single supramolecular system causes further difficulties for synthesis and its evocative biological heuristic process. Meanwhile, there are relatively few examples of detecting mercury ions as fluorescent probes, although covalent supramolecular cages have been widely reported as examples of fluorescent probes[60-64]. Therefore, it is crucial to create novel covalent supramolecular cages and use them for mercury ion detection.
Herein, we purposefully designed and constructed covalent organic cages with (R)- or (S)-binaphthyl skeletons as ligands and tri(2-aminoethyl)amine as vertices by amine aldehyde condensation-driven collaborative self-assembly. Notably, the ether chains were introduced on three symmetric binaphthyl ligands to form 20-crown-6. In addition, mercury ions were successfully recognized through fluorescence testing. To our delight, these covalent organic nanocages, which possess chemiluminescent properties, can serve as a fluorescence sensor to detect these ions. The successful synthesis of the cage had been demonstrated by NMR, mass spectrometry, Fourier transform infrared (FT-IR), and molecular simulations.
RESULTS AND DISCUSSION
As shown in Scheme 1, our investigation was commenced by using the compound 1 [3, 6, 9, 12-tetraoxatetradecane-1, 14-diol] and compound 3 (R)- or (S)-BINOL (binaphthol) as the starting substrates. Initially, (S)-4 was treated with potassium t-butoxide at 75 °C, and the resultant system was reacted with pentaethylene glycol di-p-toluenesulfonate 2 to afford the product (S)-5 in 40% yield. A Suzuki coupling reaction of bromide (S)-5 with 4-formylphenylboronic acid gave an important precursor (S)-7 in 30% yield, which has two aldehyde functionalities attached to the binaphthyl backbone. On the basis of the successful synthesis of (S)-7, covalent organic nanocage cage (S)-8 was prepared by co-self-assembly with tris(2-aminoethyl)amine in a yield of 55% as a yellow solid. The same method was used to synthesize (R)-8 for a 53% yield. The chemical structures of these chiral cages were adequately characterized by 1H NMR, mass spectrometry, and correlation spectroscopy (COSY) spectral information.
The desired covalent organic cages were successfully synthesized according to 1H NMR spectroscopy
Figure 1. Part of the 1H NMR (600 MHz, CDCl3, 22 oC) spectra of (S)-7 (A); (S)-8 (B); (R)-8 (C); (R)-7 (D) and experimental ESI-MS of the (S)-8 of [8+2H]2+ (blue) and simulated mass spectra (red) (E).
FT-IR characterization has also proven to be an important tool for detection of changes in functional groups [Figure 2]. The infrared spectrum of compound (S)-7 displayed a strong aldehyde stretching vibrational peak at 1,695 nm alongside a stretching vibration peak at 1,642 nm due to the imine formation.
The UV-Vis and fluorescence spectra also show the structural characteristics of the cage, as shown in Figure 3; the UV-Vis absorption spectra for the cage display two main peaks at 322 nm and 280 nm, which correspond to the n-π*transitions (C=N) and π-π* transitions (C=C). The UV-Vis absorption spectra for the ligand exhibited a unique peak at 337 nm, which was attributed to the existence of n-π* transitions (C=O). Furthermore, the emission wavelengths of (S)-8 and (S)-7 are 414 nm and 458 nm in the fluorescence spectra, respectively.
A simulated molecular model of cages was constructed due to many unsuccessful efforts to obtain the crystal structure of cages [Figure 4 and Supplementary Figure 24]. Analysis of the simulated cages showed the expected [2+3] structure, with two amine moieties connected by three side arms based on binol in the top and bottom views. The structure of cages had a C3 symmetric topology and three C2 axes perpendicular to the C3 axis.
Figure 4. The simulated crystal structures of (S)-8 (A) and (R)-8 (B). (N, blue, O, red, C, gray, and hydrogens are omitted for clarity).
The inherent chirality of imine cages was characterized by circular dichroism (CD) spectroscopy. As shown in Figure 5, (R)-8 and (S)-8 exhibited mirror CD spectra with highly pronounced Cotton effects in the wavelength range of 240 to 400 nm. The CD spectra of cages displayed one strong band at 286 nm, which indicated the absorption of naphthalene groups on the binaphthyl backbone. Therefore, we have successfully synthesized the covalent supramolecular cages through NMR, ESI-MS, molecular simulations, CD, and FT-IR characterization methods.
Figure 5. (A) The UV-Vis spectra of (R)-8 and (S)-8 in CDCl3 (c = 0.01 mM); (B) CD spectra of (R)-8 and (S)-8 in CDCl3 (c = 0.1 mM).
The heavy metal ion Hg2+ poses a significant threat to the environment due to its high affinity for thiol groups in proteins and enzymes. Additionally, it is important to note that mercury ions are not biodegradable. As a result, even at extremely low concentrations, they can have detrimental effects on the kidneys and brain, potentially leading to various diseases. The US EPA stipulates that the maximum concentration of mercury ions in drinking water is 10 μM. Consequently, in this work, the cage can be used as a fluorescence probe because adding mercury ions to the solution does not decompose the cage
Figure 6. Fluorescence titration of (R)-8 (10 µM) upon addition of Hg2+ ion up to 10 µM in CHCl3 (A) and the plot of fluorescence intensity versus Hg2+ concentration (B) (λex = 322 nm, Silt = 2, 2); (C) Mercury ion detection mechanism.
Figure 7. (A) Emission spectra and (B) bar graph of (R)-8 (10 µM) with various metal ions (10 µM) in CHCl3 (λex = 322 nm, slit width = 2, 2 nm).
In order to further explore the sensing ability of the cage for the Hg2+ ion, selective recognition experiments of the cage (0.2 mM) were also conducted by using different concentrations of Hg2+ (0.7, 1.4, 2.1 mM) in CHCl3 [Figure 8]. The results, as depicted in Figure 8, exhibit distinct changes in color that can be obviously observed by the naked eye. The color of the CHCl3 with spiked Hg2+ transformed from colorless to light yellow. The ability of cages to detect mercury ions in different pH solutions was also tested separately, and similar results were observed
CONCLUSIONS
In conclusion, we have demonstrated the formation of two chiral organic cages using chiral binaphthyl as the main building block. The structures of the chiral cages were characterized using 1H NMR, 2D NMR, mass spectroscopy, molecular simulation, CD, and FT-IR characterization analyses. Furthermore, the absorbance of cages exhibited a good linear correlation with Hg2+ concentrations with detection limits of
DECLARATIONS
Acknowledgments
We acknowledge the Molecular Scale Lab for mass spectrometry characterization.
Authors’ contributions
Designing the experiments, writing the manuscript, and being responsible for the whole work: Shi L, Guo Y, Song MP
Revising the first draft of the manuscript: Hao XQ
Performing the experiments and synthesizing the substrates: Li T, Ding L, Kang Y
Availability of data and materials
Please refer to the Supplementary Materials for the experimental procedures and characterization of the new compound. Other raw data that support the findings of this study are available from the corresponding author upon reasonable request.
Financial support and sponsorship
This research was funded by the National Natural Science Foundation of China (No. 22101267); the Natural Science Foundation of Henan Province (202300410477); the China Postdoctoral Science Foundation (No. 2021M692905).
Conflicts of interest
All authors declared that there are no conflicts of interest.
Ethical approval and consent to participate
Not applicable.
Consent for publication
Not applicable.
Copyright
© The Author(s) 2024.
Supplementary Materials
REFERENCES
1. Schwarzenbach RP, Escher BI, Fenner K, et al. The challenge of micropollutants in aquatic systems. Science 2006;313:1072-7.
2. Mohandoss S, Ganesan S, Palanisamy S, et al. Nitrogen, sulfur, and phosphorus Co-doped carbon dots-based ratiometric chemosensor for highly selective sequential detection of Al3+ and Fe3+ ions in logic gate, cell imaging, and real sample analysis. Chemosphere 2023;313:137444.
3. Mohandoss S, Palanisamy S, You S, Shim JJ, Lee YR. Rapid detection of silver ions based on luminescent carbon nanodots for multicolor patterning, smartphone sensors, and bioimaging applications. Anal Methods 2021;13:5719-26.
4. Sonaimuthu M, Ganesan S, Anand S, et al. Multiple heteroatom dopant carbon dots as a novel photoluminescent probe for the sensitive detection of Cu2+ and Fe3+ ions in living cells and environmental sample analysis. Environ Res 2023;219:115106.
5. Li X, Bian C, Meng X, Xiao F. Design and synthesis of an efficient nanoporous adsorbent for Hg2+ and Pb2+ ions in water. J Mater Chem A 2016;4:5999-6005.
6. Mao X, Wang L, Wang C, Lichtfouse E. Glutathione-functionalized melamine sponge, a mimic of a natural antidote, as a quick responsive adsorbent for efficient removal of Hg(II) from aqueous solutions. Environ Chem Lett 2018;16:1429-34.
7. Tajeu GS, Booth JN 3rd, Colantonio LD, et al. Incident cardiovascular disease among adults with blood pressure <140/90 mm Hg. Circulation 2017;136:798-812.
8. Wu P, Dou J, Xu Y, et al. Impact of engineering renovation on dynamic health risk assessment of mercury in a thermometer enterprise. Front Public Health 2022;10:1037915.
9. Recknagel S, Radant H, Kohlmeyer R. Survey of mercury, cadmium and lead content of household batteries. Waste Manag 2014;34:156-61.
10. Mielke HW, Gonzales C. Mercury (Hg) and lead (Pb) in interior and exterior New Orleans house paint films. Chemosphere 2008;72:882-5.
11. Ariya PA, Amyot M, Dastoor A, et al. Mercury physicochemical and biogeochemical transformation in the atmosphere and at atmospheric interfaces: a review and future directions. Chem Rev 2015;115:3760-802.
12. Awual MR, Hasan MM, Eldesoky GE, Khaleque MA, Rahman MM, Naushad M. Facile mercury detection and removal from aqueous media involving ligand impregnated conjugate nanomaterials. Chem Eng J 2016;290:243-51.
13. Chen GH, Chen WY, Yen YC, Wang CW, Chang HT, Chen CF. Detection of mercury(II) ions using colorimetric gold nanoparticles on paper-based analytical devices. Anal Chem 2014;86:6843-9.
14. Chen G, Guo Z, Zeng G, Tang L. Fluorescent and colorimetric sensors for environmental mercury detection. Analyst 2015;140:5400-43.
15. Ding Q, Li C, Wang H, Xu C, Kuang H. Electrochemical detection of heavy metal ions in water. Chem Commun 2021;57:7215-31.
16. Guo Y, Wang Z, Shao H, Jiang X. Hydrothermal synthesis of highly fluorescent carbon nanoparticles from sodium citrate and their use for the detection of mercury ions. Carbon 2013;52:583-9.
17. Gupta VK, Sethi B, Sharma R, Agarwal S, Bharti A. Mercury selective potentiometric sensor based on low rim functionalized thiacalix [4]-arene as a cationic receptor. J Mol Liq 2013;177:114-8.
18. Lin ZH, Zhu G, Zhou YS, et al. A self-powered triboelectric nanosensor for mercury ion detection. Angew Chem Int Ed Engl 2013;52:5065-9.
19. Shi Y, Li W, Feng X, et al. Sensing of mercury ions in porphyra by Copper @ gold nanoclusters based ratiometric fluorescent aptasensor. Food Chem 2021;344:128694.
20. Wang L, Peng X, Fu H, Huang C, Li Y, Liu Z. Recent advances in the development of electrochemical aptasensors for detection of heavy metals in food. Biosens Bioelectron 2020;147:111777.
21. Wang Y, Zhang L, Han X, Zhang L, Wang X, Chen L. Fluorescent probe for mercury ion imaging analysis: strategies and applications. Chem Eng J 2021;406:127166.
22. Du J, Jiang L, Shao Q, et al. Colorimetric detection of mercury ions based on plasmonic nanoparticles. Small 2013;9:1467-81.
23. Ali S, Chen X, Ahmad S, et al. Morphology-controllable bimetallic gold nanostructures for mercury detection: recent developments, challenges and prospects. Arab J Chem 2023;16:104997.
24. Bansod B, Kumar T, Thakur R, Rana S, Singh I. A review on various electrochemical techniques for heavy metal ions detection with different sensing platforms. Biosens Bioelectron 2017;94:443-55.
25. Hasan A, Nanakali NMQ, Salihi A, et al. Nanozyme-based sensing platforms for detection of toxic mercury ions: an alternative approach to conventional methods. Talanta 2020;215:120939.
26. Pierce CE, Furman OS, Nicholas SL, et al. Role of ester sulfate and organic disulfide in mercury methylation in peatland soils. Environ Sci Technol 2022;56:1433-44.
27. Miao J, Feng S, Dou S, et al. Association between mercury exposure and lung function in young adults: A prospective cohort study in Shandong, China. Sci Total Environ 2023;878:162759.
28. Yang Y, Tan Q, Lin Y, et al. Point discharge optical emission spectrometer as a gas chromatography (GC) detector for speciation analysis of mercury in human hair. Anal Chem 2018;90:11996-2003.
29. Kosta L, Byrne AR, Dermelj M. Trace elements in some human milk samples by radiochemical neutron activation analysis. Sci Total Environ 1983;29:261-8.
30. Song Y, Guo F, Zeng P, Liu J, Wang Y, Cheng H. Simultaneous measurements of Cr, Cd, Hg and Pb species in ng L-1 levels by interfacing high performance liquid chromatography and inductively coupled plasma mass spectrometry. Anal Chim Acta 2022;1212:339935.
31. Li W, Sun L, Liu C, et al. Supramolecular FeII4L4 cage for fast ammonia sensing. J Mater Chem C 2022;10:9216-21.
32. Zhang Z, Zhao Z, Wu L, et al. Emissive platinum(II) cages with reverse fluorescence resonance energy transfer for multiple sensing. J Am Chem Soc 2020;142:2592-600.
34. Ham R, Nielsen CJ, Pullen S, Reek JNH. Supramolecular coordination cages for artificial photosynthesis and synthetic photocatalysis. Chem Rev 2023;123:5225-61.
35. Bell DJ, Natrajan LS, Riddell IA. Design of lanthanide based metal-organic polyhedral cages for application in catalysis, sensing, separation and magnetism. Coordin Chem Rev 2022;472:214786.
36. Hou YJ, Wu K, Wei ZW, et al. Design and enantioresolution of homochiral Fe(II)-Pd(II) coordination cages from stereolabile metalloligands: stereochemical stability and enantioselective separation. J Am Chem Soc 2018;140:18183-91.
37. Giri N, Del Pópolo MG, Melaugh G, et al. Liquids with permanent porosity. Nature 2015;527:216-20.
38. Rimsza JM, Nenoff TM. Porous liquids: computational design for targeted gas adsorption. ACS Appl Mater Interfaces 2022;14:18005-15.
39. Kim T, Park JY, Hwang J, Seo G, Kim Y. Supramolecular two-dimensional systems and their biological applications. Adv Mater 2020;32:e2002405.
40. Shi L, Lu S. Precise carbon dots synthesis: building bridges between organic chemistry and inorganic chemistry. Sci Bull 2022;67:2369-71.
41. Shi L, Wang B, Lu S. Efficient bottom-up synthesis of graphene quantum dots at an atomically precise level. Matter 2023;6:728-60.
42. Han X, Guo C, Xu C, et al. Water-soluble metallo-supramolecular nanoreactors for mediating visible-light-promoted cross-dehydrogenative coupling reactions. ACS Nano 2023;17:3723-36.
43. Zheng S, Xu Y, Su P, et al. Anion-induced differential assembly and structural transformation of supramolecular coordination cages. Chinese Chem Lett 2023:108477.
44. Liu W, Liu G, Zhu X, et al. Tailored metal-organic tetrahedral nanocages with aggregation-induced emission for an anti-counterfeiting ink and stimulus-responsive luminescence. New J Chem 2022;46:8062-8.
45. Miao L, Zhu X, Liu G, et al. Tunable aggregation-induced fluorescent and pressure-responsive luminescence supramolecular cages achieved by subcomponent self-assembly. Chinese Chem Lett 2023;34:107921.
46. Wang QQ, Day VW, Bowman-James K. Chemistry and structure of a host-guest relationship: the power of NMR and X-ray diffraction in tandem. J Am Chem Soc 2013;135:392-9.
47. Zhang D, Ronson TK, Greenfield JL, et al. Enantiopure [Cs+/Xe⊂Cryptophane]⊂FeII4L4 hierarchical superstructures. J Am Chem Soc 2019;141:8339-45.
48. Liu X, Shi Z, Xie M, et al. Single-handed double helix and spiral platelet formed by racemate of dissymmetric cages. Angew Chem Int Ed Engl 2021;60:15080-6.
49. Fantozzi N, Pétuya R, Insuasty A, et al. Selective detection of choline in pseudophysiological medium with a fluorescent cage receptor. Org Lett 2023;25:2444-9.
50. Chakraborty S, Newkome GR. Terpyridine-based metallosupramolecular constructs: tailored monomers to precise 2D-motifs and 3D-metallocages. Chem Soc Rev 2018;47:3991-4016.
51. Chen Y, Wu G, Chen B, et al. Self-assembly of a purely covalent cage with homochirality by imine formation in water. Angew Chem Int Ed Engl 2021;60:18815-20.
52. Harris K, Fujita D, Fujita M. Giant hollow MnL2n spherical complexes: structure, functionalisation and applications. Chem Commun 2013;49:6703-12.
53. Yan LL, Yao LY, Ng M, Yam VW. Stimuli-responsive and structure-adaptive three-dimensional gold(I) cluster cages constructed via "de-aurophilic" interaction strategy. J Am Chem Soc 2021;143:19008-17.
55. Wilson A, Gasparini G, Matile S. Functional systems with orthogonal dynamic covalent bonds. Chem Soc Rev 2014;43:1948-62.
57. Montà-González G, Sancenón F, Martínez-Máñez R, Martí-Centelles V. Purely covalent molecular cages and containers for guest encapsulation. Chem Rev 2022;122:13636-708.
58. Wang H, Jin Y, Sun N, Zhang W, Jiang J. Post-synthetic modification of porous organic cages. Chem Soc Rev 2021;50:8874-86.
59. Quan MLC, Cram DJ. Constrictive binding of large guests by a hemicarcerand containing four portals. J Am Chem Soc 1991;113:2754-5.
60. Bravin C, Badetti E, Licini G, Zonta C. Tris(2-pyridylmethyl)amines as emerging scaffold in supramolecular chemistry. Coordin Chem Rev 2021;427:213558.
61. Fernández-Caro H, Lostalé-Seijo I, Martínez-Calvo M, Mosquera J, Mascareñas JL, Montenegro J. Supramolecular caging for cytosolic delivery of anionic probes. Chem Sci 2019;10:8930-8.
62. Hashikawa Y, Murata Y. Probing the regioselectivity with encapsulated H2: diels-alder reaction of an open-cage C60 derivative with anthracene. Chemistry 2019;25:2482-5.
63. Plessius R, Orth N, Ivanović-Burmazović I, Siegler MA, Reek JNH, van der Vlugt JI. Reversible multi-electron storage in dual-site redox-active supramolecular cages. Chem Commun 2019;55:12619-22.
64. Wu SY, Guo XQ, Zhou LP, Sun QF. Fine-tuned visible and near-infrared luminescence on self-assembled lanthanide-organic tetrahedral cages with triazole-based chelates. Inorg Chem 2019;58:7091-8.
65. Bhatta SR, Mondal B, Vijaykumar G, Thakur A. ICT-isomerization-induced turn-on fluorescence probe with a large emission shift for mercury ion: application in combinational molecular logic. Inorg Chem 2017;56:11577-90.
Cite This Article

How to Cite
Download Citation
Export Citation File:
Type of Import
Tips on Downloading Citation
Citation Manager File Format
Type of Import
Direct Import: When the Direct Import option is selected (the default state), a dialogue box will give you the option to Save or Open the downloaded citation data. Choosing Open will either launch your citation manager or give you a choice of applications with which to use the metadata. The Save option saves the file locally for later use.
Indirect Import: When the Indirect Import option is selected, the metadata is displayed and may be copied and pasted as needed.
About This Article
Copyright
Data & Comments
Data
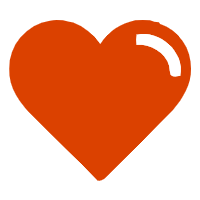
Comments
Comments must be written in English. Spam, offensive content, impersonation, and private information will not be permitted. If any comment is reported and identified as inappropriate content by OAE staff, the comment will be removed without notice. If you have any queries or need any help, please contact us at [email protected].