Nanostructured intermetallics: from rational synthesis to energy electrocatalysis
Abstract
Intermetallics are a large family of structurally ordered alloys that combines a metal element with other metal/metalloid elements with a clearly defined stoichiometric ratio. Intermetallics possess abundant crystal structures and atomic packing motifs, giving rise to a great variety of electronic configurations and surface adsorption properties. The wide electronic and geometric diversity makes intermetallics a highly promising population for discovering advanced materials for various catalytic applications. This review presents recent advances in the reaction synthesis of intermetallic materials at the nanoscale and their energy-related electrocatalytic applications. Initially, we introduce general principles for the formation of stable intermetallic structures. Subsequently, we elaborate on common synthetic strategies of nanostructured intermetallics, such as thermal annealing, wet-chemical methods, metallothermic reduction, and template-directed synthesis. Furthermore, we discuss the wide employment of these intermetallic nanocatalysts in many different kinds of electrocatalytic applications, as well as highlight the theoretical and experimental evidence for establishing a reasonable relationship between atomic arrangement and catalytic activity. Finally, we propose some perspectives for future developments of intermetallic preparation and catalytic applications.
Keywords
INTRODUCTION
The chemical industry largely depends on heterogeneous catalysis, in which various metal materials are employed as attractive catalysts for the production of bulk chemicals, pharmaceuticals and agrochemicals[1-4]. For a given catalytic application, the catalysts should fulfill multiple criteria such as high activity, low cost, good selectivity and stability, which usually cannot be achieved by a single metal. Introducing another metal/metalloid component to form an alloy has long been used to drastically expand the property landscape and potentially meet these essential criteria[5,6]. The metallic alloys are typically separated into disordered structure (i.e., substitutional alloy) and ordered structure (i.e., intermetallic compound)[7,8]. While the substitutional alloy shows the crystal phase of one of constituting elements with varying composition and random atomic arrangement, the constituting elements in the intermetallic compounds have well-defined stoichiometry and highly ordered arrangement. At present, more than 25,000 intermetallic compounds are known since this concept was proposed by British metallurgists in 1914 [Figure 1]. These intermetallics possess complex crystal structures and unique chemical bonding, which are not easily obtained in random alloys and other compounds, providing novel patterns of electronic structures and great potential to discover new chemical properties, such as catalytic properties.
Early in the 1930s, the catalytic properties of intermetallics were investigated[9]. Among pioneering examples, Brown et al. reported intermetallic Tl2Pb and Cd3Cu2 with good catalytic activities toward vapor-phase reduction of nitrobenzene[9,10]. The electrocatalytic application of intermetallic compounds started already back in the 1970s, when the development of fuel cell technology drove the great requirement for new electrocatalysts[11-13]. Some Pt and Ni-based intermetallics (e.g., PtPd, Ti2Ni) were thus explored as potential fuel cell electrodes. Subsequently, the applications of intermetallic compounds expand to many electrocatalytic reactions such as water electrolysis, CO2 reduction, and nitrogen fixation[14-19]. The alloy catalysts with intermetallic structures present great success in achieving high activity, because strong chemical interactions between the alloying elements modify the electronic structure and surface chemisorption properties. The peculiar crystal structure and chemical bonding in some intermetallics produce the unexpected catalytic benefits previously rooted. In many cases, the intermetallic alloy catalysts possess more superior catalytic and structural stability than their disordered counterparts, and benefit from the ordered arrangement and strong interactions of involved metals/metalloids to restrain segregation or decomposition during catalytic reactions[20,21].
The catalytic performances of intermetallics depend on their composition, crystal phase, shape, and size. Conventionally, intermetallic compounds are prepared by solid-state methods under high temperature and pressure conditions, leading to uncontrollable morphology and large particle size, which limit the catalytic exploitation of intermetallic structures[22,23]. It is only in the last twenty years that the great progress in modern synthetic chemistry promotes the wide catalytic applications of intermetallics, especially in the energy-relevant electrocatalytic fields. The new synthetic strategies and design principles can control the growth of nanoparticulate intermetallic materials to yield different crystal phases, shapes and sizes[24-28]. Moreover, the developments of advanced characterization tools and computational chemistry enable the opportunity to reveal the geometric and electronic effects that determine catalytic performance by using the intermetallic materials with homogeneous surface chemical environments as model catalysts[29-31]. It is important to offer an overview of the latest progress in chemical syntheses of shape-/size-/composition-controlled intermetallics and their electrocatalytic applications.
In this work, the recent research advances made in the synthesis of various intermetallics for important electrocatalytic reactions are reviewed. The basic principles of the formation of stable intermetallic structures are introduced, with emphasis on Hume-Rothery rules, phase diagrams, kinetics and thermodynamic analyses. Then, mainstream strategies to obtain nanostructured intermetallic materials and control the composition, crystal phase, shape, and size are outlined. In the next section, the representative use of intermetallic nanocrystals as electrocatalysts is discussed for various reactions including the hydrogen evolution reaction (HER), the oxygen reduction reaction (ORR), the CO2 reduction reaction (CO2RR) and the nitrogen reduction reaction (NRR). The role of these intermetallic structures in the electrochemical reactions is highlighted, and several perspectives on future investigations of intermetallic catalysts are proposed.
GENERAL PRINCIPLES FOR INTERMETALLIC FORMATION
Hume-rothery rules
Even the simplest bimetallic systems can be complex due to their different states of the intermediate phase, which include immiscible mixtures and miscible systems. Miscible systems can be further classified into random alloys, in which the atomic positions of the metals are disordered, and intermetallic alloys, in which the atomic positions are ordered. In the 1920s and 1930s, British metallurgist William Hume-Rothery and his colleagues proposed the Hume-Rothery Rules, which is an empirical guideline for determining the intermediate phase state of binary alloy systems[32,33]. This rule is based on factors such as atomic radius, crystal structure, electronegativity, and electronic structure, and can be a useful tool for designing binary alloys.
According to the Hume-Rothery Rules, when the atomic radii of two elements differ by more than 15%, they are difficult to miscible. For example, atomic radii of Co and Ag are 135 pm and 160 pm, respectively. Regardless of the ratio or temperature of the two metals, it is not possible to obtain a miscible system. Instead, an immiscible solid mixture will form [Figure 2A]. In contrast, Ni (135 pm) has a similar atomic radius to Co, and Ni-Co can form a miscible disordered alloy [Figure 2B]. In addition to atomic radius, the electronegativity difference (Δχ) between the two metals also plays an important role in the formation of intermetallic compounds. A large electronegativity difference strengthens the chemical bond between the two metals, making it more thermodynamically inclined to generate an ordered structure. For example, Ni and Co have similar electronegativities (χNi = 1.92 and χCo = 1.88), but Pd has a higher electronegativity of 2.2. Pd and Co can form both random solid solutions and ordered intermetallic compounds, such as Pd3Co and PdCo, under specific conditions [Figure 2C].
Figure 2. (A-C) Phase diagrams of three binary alloy systems. (A) Co-Ag immiscible system; (B) Co-Ni disordered alloy system; (C)
When Cu family elements form alloys with polyvalent metal elements (such as Zn, Ga, and Ge), the atomic radius and electronegativity difference become secondary factors, and the electron concentration effects become dominant[34]. The specific phases can be predicted by the average number of itinerant electrons per atom (e/a), as shown in a corresponding between phase and e/a relationship in Figure 3. In other words, the maximum solubility of the solute element will decrease as the e/a increases to ensure that the e/a of alloy is at a specific value. For example, when Zn, Ga and Ge form α-phase alloys with Cu, respectively, the maximum solubility is 38%, 20% and 12%. The e/a of Zn0.38Cu0.62 is easily calculated to be 1.38, and the e/a of the remaining two systems is also about 1.4. Different from the first two empirical rules, the Hume-Rothery electron concentration effect rule has been further explained with the development of condensed matter physics. In 1936, Mott and Jones successfully explained this rule by using the nearly free-electron model in the first Brillouin region[35]. According to this model, the critical e/a [ (e/a)c ] of the system can be given by the spheroidal electron cloud tangent to the first Brillouin region [Figure 3]. For example, the (e/a)c values of α-, β- and γ-phase are 1.362, 1.480 and 1.538, which are basically consistent with the experimental results. The electron concentration effect is still widely studied today, for example, by using the total number of electrons as a new descriptor, and by trying to extend the rule to other transition metals.
Figure 3. Schematic of the relationship between electron concentration (e/a) and crystal phase structure, and the First Brillouin Zone and critical electron concentration (e/a)c values of typical crystal phase structures (α-, β- and γ-phase).
The Hume-Rothery rules are effective for determining the crystal structures of numerous intermetallic compounds. However, these rules have limitations and are not applicable to all binary systems. For instance, the 18-n rule can be employed to ascertain the bond formation of intermetallic compounds composed of transition metals and p-block elements[36,37]. Meanwhile, the Zintl phase, which is formed by alkali/alkaline earth metals and p-block elements, does not adhere to the Hume-Rothery rules. A typical example is NaTl, which exhibits properties of brittleness, diamagnetism, and low conductivity despite being comprised of two metals. These nonmetallic properties arise from the significant electronegativity difference between sodium and thallium. After thallium accepts an electron from sodium, it still fails to achieve an octet (only four electrons), resulting in the formation of a regular tetrahedral network similar to carbon atoms in diamonds. This is so-called the Zintl-Klemm counting scheme[38-40], in which the anion of the Zintl phase forms polyanionic substructures resembling elements with the same number of outermost electrons, such as white phosphorus (tetrahedral P4) and sulfur (circular S8). However, these compounds are infrequently utilized as electrocatalysts due to their poor electrical conductivity, and thus will not be elaborated upon in this review.
Thermodynamic analysis and phase diagram
The Hume-Rothery rules is an empirical guideline used to predict the conditions under which metallic alloys will exhibit miscibility. The Gibbs free energy of mixing is a key determinant in judging whether the system can spontaneously become miscible. We also take the binary system as a simple example, and the Gibbs free energy change in the mixing process (ΔGbulk) of the bulk system can be written as follows.

where ΔH is the enthalpy change of mixing, T is the absolute temperature, and ΔS is the entropy change of mixing. It is well established that the entropy of the system must increase during the mixing process, meaning that ΔS is always positive. The sign of ΔH depends on whether the formation of new bonds in the system is exothermic or endothermic. If the ΔH is negative, then the ΔG will be less than zero at any temperature leading to a miscible system, such as the Co-Ni system. However, when ΔH is positive, the effect of temperature should be considered [Figure 2D]. The influence of TΔS on the Gibbs free energy of the system is relatively small at lower temperatures, which is because the enthalpy change is generally 2-3 orders of magnitude larger than the entropy change. For example, in the Co-Ag immiscible system, the ΔH is considerably larger than zero, so the two metals are still not miscible at 2000 K. This formula can also be used to guide the transition process between disordered alloys and ordered intermetallic compounds. When ΔG = 0, the system is in a two-phase mixed equilibrium state, and the temperature is called critical phase-transition temperature (Tc)[7]. In general, the enthalpy change of the disorder-to-order transition is negative, as intermetallic compounds typically have higher bond energies, making the ordered system more thermodynamically stable. The entropy change of this process is also negative, as the atoms in the system become more ordered. Thus, low temperatures favor the formation of ordered metal compounds, while high temperatures favor the formation of disordered alloys [Figure 2E and F].
In practical systems, it is critical to take into account the effect of surface energy, which is not present in ideal bulk systems. The smaller the particle size, the larger the influence of surface energy on the overall energy. As a result, the equation for Gibbs free energy change in the mixing process needs to be modified to consider surface energy[41,42]:

where A is the surface area of the particle and Δγ is the change in surface energy. When bulk system and nanoparticle system reach critical phase-transition temperature, ΔGbulk and ΔGnano are zero. Combined with equations 1 and 2, the Tc of the two systems can be written as equation 3[28].

where ΔHv is volume-specific bulk enthalpy. In the disorder-to-order transition, it is clear that ΔHv is negative, the same sign as ΔH. And since the average bond energy is higher on ordered surfaces, Δγ is also negative. According to equation 3, as the particle size of nanoparticles decreases, the specific surface area (A/V) increases. The nanoparticle system should have a lower critical phase-transition temperature than the bulk system[43,44]. A typical example of this conclusion is the discovery by Alloyeau et al. that CoPt nanoparticles have a Tc of 175-325 ℃ lower than bulk materials[45]. Moreover, the exposed surface of the nanoparticles also influences the energy of the system, as the surface energy of different crystal faces can vary. In conclusion, the Gibbs free energy and the critical phase transition temperature of the system will be impacted by the composition, particle size, shape, and other factors.
Although thermodynamics at a given temperature can provide an accurate indication of whether a system is miscible at a given temperature, it is still not intuitive. The phase diagram can visualize the state of the reaction system and provide a preliminary reference for the stoichiometric ratio and temperature required for the synthesis of metal compounds. The Gibbs phase rule is the basis for phase diagrams, a formula introduced by Josiah Willard Gibbs in the 1870s[46]. This rule holds that the state of a system is entirely described by its thermodynamic parameters (e.g., temperature and pressure) at equilibrium.

where C is the composition fraction of the system, P is the number of phases, and F is the number of degrees of freedom, that is, the number of independent variations of the system. For a binary system with
H. W. Bakhuis Roozeboom applied this rule and produced the first iron-carbon phase diagram in 1900[47]. The phase diagram can intuitively show the equilibrium state of the system at any temperature. Additionally, the phase diagram can be used to predict the behavior of the high-temperature system during cooling. Moreover, intermetallic compounds with fixed composition are represented as narrow regions or vertical lines in the phase diagram. This can make it challenging to experimentally plot phase diagrams. By combining theoretical simulation and thermodynamic analysis, phase diagrams can be accurately mapped and the prediction of new intermetallic compounds becomes possible. The thermodynamic parameter, pressure, which is more difficult to control in experiments, can also be easily obtained from computational simulations. For example, Clarke et al. utilized DFT calculations to create the temperature-pressure phase diagram of the Cu-Bi binary system and successfully synthesized superconducting Cu11Bi7[48]. Theoretical prediction combined with high-throughput screening and machine learning methods has played an important role in predicting and characterizing the properties of intermetallic compounds[49,50].
Kinetics analysis
Thermodynamics and phase diagram can provide crucial guidance for the synthesis of metallic compounds, but a range of kinetic factors need to be considered in the actual reaction process. While thermodynamic conditions may be favorable during synthesis, the presence of kinetic energy barriers can result in the formation of metastable structures. In fact, the successful preparation of ordered intermetallic compounds is dependent on balancing the thermodynamic driving force and the kinetic energy barrier, and the system must cross the activation energy barrier to reach the most stable thermodynamic state[51] [Figure 4A].
Figure 4. (A) Schematic of the free energy of the disordered structure converted to the ordered structure; (B) Schematic of nucleation rate, diffusion rate and total reaction rate as a function of temperature.
The Johnson‐Mehl‐Avrami‐Kolmogorov (JMAK) theory describes in detail the dynamics during the formation of ordered phases[52-54]. Here we will examine the two fundamental processes in the transformation from disorder to order, the formation of new phases (nucleation) and the rearrangement of local atoms (diffusion). According to the Arrhenius equation, the reaction rates of the two processes are as follows.
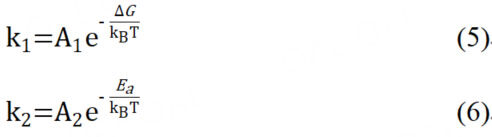
where A1 and A2 are the respective preexponential factors, kB is the Boltzmann constant, ΔG is the Gibbs free energy change in phase transition, Ea is the activation energy of atomic diffusion, and T is the absolute temperature. For the reaction to occur, ΔG must be negative, while the remaining parameters (A1, A2, kB, Ea, and T) are all positive. Therefore, k1 and k2 have diametrically opposite tendencies with respect to temperature. The reaction rate of k1 decreases while that of k2 increases with increasing temperature. The overall transition rate depends on the product of k1 and k2, so there exists an optimal transition temperature. In other words, the curve of the total rate as a function of temperature [Figure 4B] shows a volcanic pattern[55,56].
Particle size also influences the dynamics of the "disorder-to-order" process. Smaller particles will reduce the thermodynamic driving force and thus cause a decrease in k1, as described in Equation 2. However, diffusion is easier in small particles. Therefore, there may also be an optimal range of particle sizes that is most favorable for the formation of ordered intermetallic compounds. In addition, the hole concentration and the interatomic bond strength also influence the reaction rate. Generally speaking, increasing the concentration of vacancies effectively facilitates the phase transition process because the activation energy of vacancy diffusion is lower[57]. In summary, several factors including temperature, composition, particle size and shape, and hole concentration must be considered to balance the thermodynamic driving force and kinetic energy barrier during the synthesis of ordered intermetallic compounds[58-63].
SYNTHESIS OF INTERMETALLICS
This section mainly introduces some main methods for the synthesis of nanoscale intermetallic compounds, although there are some traditional methods, such as solid-state synthesis[64,65], arc melting[66,67], and high-energy ball milling[68,69], which are widely used to synthesize intermetallic compounds. However, these synthetic methods usually have uncontrollable crystallization process, large grain size, and easy-to-generate miscible phase. Therefore, these methods are not summarized in this review. In the following, we summarize several important strategies for nanoscale intermetallic compound synthesis.
Thermal annealing
Thermal annealing, which heats random alloys at high temperatures for a sufficiently long time, is the simplest and most straightforward way to generate intermetallic compounds [Figure 5A]. According to thermodynamic and kinetic analyses, random alloy structures are more easily generated than intermetallic compounds when the reaction temperature is maintained below the disordered-to-ordered transition temperature. At elevated temperatures, thermal vibration of metal atoms is promoted, facilitating the diffusion of the metal and making it easier for random alloys to cross the barrier to form ordered compounds[7,28]. Using this method, a variety of bimetallic and trimetallic compounds, as well as intermetallic semiconductors and superconducting nanocrystals, have been synthesized. However, the thermal annealing method also has its disadvantages: higher annealing temperature will promote Ostwald ripening and aggregation, which results in the increase of nanocrystal size and uneven size distribution[70]. At the same time, the catalytic performance of intermetallic compound nanocrystals is reduced. Therefore, it is necessary to control the annealing temperature and annealing time, and to select appropriate support and coating layers to prevent aggregation of nanocrystals at high temperatures.
Figure 5. Schematic of the main synthesis methods for intermetallic compounds, including (A) thermal annealing; (B) metallothermic reduction; (C) wet chemistry method; and (D) seed-mediated growth.
Direct annealing is currently the most widely used method to generate ordered intermetallic nanocrystals. In this process, random alloys obtained by the liquid phase method were used as primary intermediates, and then they were converted into atomically ordered intermetallic nanocrystals by heat treatment in an atmosphere or vacuum. Due to the relatively high kinetic barriers involved in atomic ordering, annealing at high temperatures (usually > 500 °C) and/or long times is required. For example, Abe et al. converted atomically disordered Pt3Ti nanoparticles into larger atomically ordered Pt3Ti nanoparticles by annealing above 500 °C[71]. The authors annealed Pt3Ti in vacuum at various temperatures and analyzed the PXRD profiles of the products, and they found that when the annealing temperature exceeds 550 °C, the PXRD of the annealed product shows the superlattice peak of Pt3Ti in the ordered Cu3Au-type structure. When the annealing temperature was further increased to 600 °C, the intensity of the above lattice peaks was improved. The results indicate that the annealing temperature is an important parameter in adjusting the order degree of the prepared nanocrystals. However, higher annealing temperatures inevitably lead to a sharp increase in particle size. Atomically disordered Pt3Ti nanoparticles with a particle size of 3 ± 0.4 nm were annealed in vacuum at 600 °C and ordered Pt3Ti nanoparticles with a particle size of 37 ± 23 nm were obtained. Chi et al. synthesized a series of Pt3Co nanocrystals with different atomic arrangements during in situ annealing and observed five distinct stages of element rearrangement on the surface of nanocrystals by scanning transmission electron microscopy[72]. They found that when the sample temperature was raised to 600 °C and held for 20 min, the ordered Pt3Co phase began to nucleate on {001}. By prolonging the annealing time at the same temperature, it is advantageous to form fully ordered phases with similar morphology. Therefore, careful selection of annealing temperature, annealing time, and annealing atmosphere plays a crucial role in promoting the degree of order, size, and shape of the final product.
Support-assisted annealing. Unlike direct annealing, the support-assisted annealing method deposits nanocrystals on stable supports with a larger surface area. The catalyst support can prevent unwanted aggregation of the active catalyst during thermal annealing[73]. In addition, electron rearrangement occurs at the interface between nanocrystals and support, which can increase the electron density of nanocrystals and improve the performance of catalysts. Carbon is one of the most common carriers widely used in fuel cells and batteries[74-76]. Yang et al. showed that Pt loading on sulfur-doped carbon support produced strong platinum-sulfur bonds, allowing small platinum alloy nanoparticles (< 5 nm in diameter) to be stabilized at temperatures up to 1000 °C[77]. They use sulfur-doped carbon as a support for synthesized intermetallic libraries of small nanoparticles consisting of 46 combinations of platinum with 16 other metal elements. Other carbon supports, such as porous carbon, porous oxide/carbon composite and graphene, are also commonly used to synthesize various intermetallic nanocrystals. Ji et al. modified the surface of ordered mesoporous carbon (OMC) with sulfur and used the surface-modified OMC-S as catalyst support to prepare OMC-PtBi nanocrystals embedded in the porous structure of OMC[78]. Because the OMC has a connected pore structure, a high pore density and a specific surface area, and the sulfur has a strong affinity for metals, the metal precursor in solution can be adsorbed and combined into the pores of OMC, and the particle growth can be limited in the reduction process, to obtain ultra-fine dispersed metal nanocrystals. The resulting nanocrystals have a size of about 2-3 nm and a uniform size distribution. In addition, they also synthesized other intermetallic PtM (M = Pb, Pd, Ru) catalysts using this method.
Alternatively, intermetallic can be prepared at the interface of metal and support by strong metal-support interactions[79]. Generally, noble metal nanocrystals are carried by oxide support and converted into intermetallic compounds by reduction at high temperatures. For example, Pd NPs supported on ZnO,
Coating-assisted annealing. By coating the nanocrystals with polymers, oxide protective layers (SiO2, MgO) and KCl substrates, the diffusion between nanocrystals can be eliminated, thus effectively alleviating agglomeration and sintering during thermal annealing and precisely controlling the size of nanocrystals[81]. Fujimori et al. coated the surface of disordered face-centered cubic (fcc)-FePt with SiO2 and subsequently thermally annealed to obtain ordered L10-FePt nanocrystals[82]. SiO2 can limit the diffusion of Fe and Pt atoms during annealing, and the resulting material has an average particle size of 6.4 nm. Kim et al. used a similar approach coating the disordered fcc-FePt with a MgO protective layer (FePt/MgO), and heat-treated it at 800 °C to obtain an intermetallic FePt with an ordered face-centered tetragonal (fct) structure[83]. Song et al. developed a small molecule-assisted impregnation approach to realize the general synthesis of a family of Pt-based intermetallics[84]. They utilized molecular additives containing functional groups as ligands to coordinate Pt (IV) in impregnation, and then thermally converted to intermetallic nanoparticles coated on a hybrid atom-doped graphene layer. The method realized the general synthesis of 18 binary intermetallics, including Pt3M (M = Ti, V, Cr, Mn, Fe, Co, Al, Ga, In, Ge, Sn), PtM (M = Mn, Fe, Co, Ni, Cu, Zn) and PtCu3. Other inorganic salts such as NaCl and KCl were also able to stabilize nanoparticles during high-temperature annealing. However, these salts do not form a tight shell around each nanoparticle and are less efficient in stabilizing nanoparticles to prevent aggregation/sintering[85,86].
Defect-assisted annealing. The complete transition from disorder to order can also be achieved by introducing defects to lower the atomic diffusion barrier and promote mutual diffusion between metal atoms[87]. At present, defects are mainly introduced into the crystal interior by the generation of oxygen vacancies or by the introduction of a third element[88]. Sun et al. prepared disordered fcc-FePt-Fe3O4 nanocrystals by controlling the decomposition of Fe(CO)5 and reduction of Pt(acac)2, and obtained fully ordered fct-FePt nanocrystals by subsequent thermal annealing[89]. In the synthesis process, fcc-FePt NPs were first synthesized and then oxidized in air to obtain dumbbell fcc-FePt-Fe3O4 NPs, which were surrounded by a polycrystalline Fe3O4 shell. Then defects are generated when Fe3O4 is reduced to Fe, and the dumbbell structure ensures that Fe/Pt is easy to diffuse to form a fully ordered fct structure[90]. Wang et al. used a bifunctional core/shell Pt/NiCoOx as a precursor system to prepare sub-6 nm monodisperse ordered L10-PtNi0.8Co0.2 nanocrystals. NiCoOx can provide abundant oxygen vacancies to promote the diffusion of Pt/Ni/Co atoms and act as a protective shell to inhibit the sintering of nanocrystalline during thermal annealing[91].
Metallothermic reduction
Thermal reduction is a method of producing intermetallic compounds by reducing a metal source at high temperatures with a reducing agent [Figure 5B]. Usually, metal oxides, halides, or sulfides are used as metal sources, reducing gases (H2, B2H6), and active metals (K, Mg, Al, Sn) as reducing agents. Due to the wide variety of reducing agents available in this method, many typical reactions have been developed, including solid-state metathesis reactions and metal thermal reduction reactions, to achieve the simple, rapid or universal synthesis of intermetallic compounds. Recently, our group has prepared a series of intermetallic borides and silicides by thermal reduction[92,93]. Our group employed the boron thermal reaction between boron powder and metal oxide under KCl-NaCl molten salt conditions to prepare phase-pure borides. Twelve single metals MB2 (M= Ti, V, Cr, Mn, Zr, Nb, Mo, Hf, Ta, W, Re and Ru) were prepared by this method at relatively low temperatures (900-1000 ℃)[94]. The twelve metal diborides adopt four types of crystal structures. Their conspicuous structural difference lies in the different two-dimensional covalent boron sheet subunits. Later, we developed a solid-state metathesis reaction using magnesium diboride
Wet chemistry method
Compared with the thermal annealing method, the wet chemical method is easier to control the morphology and size of metal compounds [Figure 5C]. In wet chemistry, intermetallic compounds are synthesized by direct reaction with the addition of precursors, reducing agents, capping agents, and surfactants in solution. Because high-temperature annealing is not required, particle agglomeration during annealing is avoided. However, due to the limitation of the boiling point of the solvent, the reaction can only be carried out at a low temperature, so it is necessary to adopt a suitable strategy to reduce the barrier of the transition from disordered alloys to ordered intermetallic compounds. The following two strategies can be adopted for the preparation of intermetallics[97].
(i) Adding halide ions (Cl−, Br−, I−) or strong reducing agents (ascorbic acid (AA), tetrabutylammonium bromide (TBAB), NaBH4) to the reaction solution to slow down the crystal growth rate. Rong et al. synthesized surface defect-rich Pt3Sn using Pt(acac)2 and SnCl2·2H2O as metal precursors, polyvinylpyrrolidone (PVP) as surfactant, and N, N-dimethylformamide (DMF) as solvent and reducing agent[98]. During synthesis, Cl− released from SnCl2 slows crystal growth and cooperates with O2 to etch the nanocrystals. According to the growth-etching synergetic growth mechanism reported by Tilley, the corrosion process proceeds synchronously with the growth process in the presence of higher concentrations of corrosive agents. At the beginning of the reaction, the etching process is slow because the concentration of Cl− in the solution is low. However, with Sn atoms reduced, more Cl− is released into the solution, which leads to a faster etching rate and more defect formation. The newly generated Sn atoms can diffuse from the surface into the Pt-rich lattice to form PtSn solid solution. Liao et al. synthesized novel PtBi nanoplatelets (NPLs) and PdBi nanowires (NWs) with controlled shape, size, and composition in the presence of oleylamine (OAm) and NH4Br[99]. Using a strong reducing agent, the simultaneous reduction of two metal precursors is also a method of synthesizing intermetallic compounds. Maksimuk et al. used TBAB as a reducing agent to simultaneously reduce Pt(acac)2 and Pb(acac)2 to synthesize ordered metal-interstitial PtPb nanorods[100]. Cable et al. reported the synthesis of a series of tin intergeneric compounds using NaBH4 as a strong reducing agent[101].
(ii) By seed-mediated synthesis, metal nanocrystals with well-defined size, shape and composition are first synthesized, and then the second metal is diffused into the synthesized seeds by diffusion growth[102] [Figure 5D]. This synthesis strategy was pioneered by Cable and Schaak, who first reported the generation of intermetallic M-Zn nanocrystals (M = Au, Cu, and Pd) by seed-mediated diffusion growth using zero-valent Zn precursors in a hot organic amine solvent. Samanta et al. synthesized ultra-small monodisperse intermetallic NiZn nanoparticles by converting Ni nanoparticles into an ordered alloy using diethylzinc [Zn(C2H5)2] as precursor[103]. During the transformation, the particles retained their morphology, and the estimated particle size of the NiZn NPs was ~4.5 nm. The seed-mediated growth approach enabled the preparation of a variety of noble (gold, silver, platinum, and palladium) and non-noble (tin, plumbum, and copper) intermetallic compounds, and can realize the control of morphology. An excellent example was demonstrated in the solution-phase synthesis of core/shell PbPt/Pt with PbPt core being in hexagonal structure[104]. The core/shell NPs were made by using Pt(acac)2 and Pb(acac)2 as the metal precursors, oleylamine (OAm)/octadecene (ODE) mixture as solvents and surfactants, and AA as the reducing agent. Under the reaction condition, Pb was first reduced and Pt was deposited on the Pb seeds, followed by controlled Pt diffusion into the Pb seeds. With the Pb/Pt ratio controlled at about 1:1, hexagonal PbPt was obtained. Meanwhile, the authors found that using AA as a reducing agent was the key to forming a well-organized Pt atomic layer, because AA can be used as a weak acid to remove Pb during synthesis, allowing Pt atoms to diffuse and rearrange at higher temperatures.
Using Au nanocrystals as precursors, Chen et al. prepared monodisperse CuAu and Cu3Au nanocrystals by seed diffusion method[105]. They prepared Cu3Au and CuAu using 6.3 nm and 8.5-9.5 nm Au seeds at different temperatures, respectively. According to powder X-ray diffraction (XRD) patterns of the product, Cu3Au intermetallic compounds of cubic order were formed at 300 °C when the Cu/Au atomic ratio was 3:1. The intermetallic compound CuAu was obtained at 280 °C when an equal molar amount of raw material was used. In addition, Clarysse et al. report the development of a colloidal synthesis method based on amalgamation of monometallic nanocrystal seeds with low melting point metals, and have used this method to obtain uniform intermetallic nanocrystals of Au-Ga, Ag-Ga, Cu-Ga, Ni-Ga, Pd-Ga, Pd-In and Pd-Zn compounds[106]. They showed that besides Ga, other low melting point metals (In and Zn) could be used to synthesize intermetallic compounds.
Although many methods have been used to synthesize intermetallics, they have sufficiently different advantages and disadvantages. For example, metallothermic reduction can synthesize highly-crystalline intermetallics that are difficult to obtain by wet chemical methods, such as silicates and borides, but the size of the as-synthesized products is still relatively large, dozens or hundreds of nanometers[93]. Wet chemical method can accurately control the morphology and size of intermetallics, but may involve some expensive metallic reagents [e.g., Pt(acac)2, Pd(acac)2] and auxiliary solvents (e.g., oleylamine, benzyl alcohol), resulting in high synthesis cost and difficulty in large-scale synthesis[97]. A significant trend for large-scale production of intermetallic catalysts is the development of green and low-cost synthesis of small-size intermetallic nanoparticles. In addition, the current synthesis of intermetallics mainly relies on trial‐and‐error approaches, which are inefficient and tedious. More research is needed on general methods and design principles for the synthesis of intermetallic phases.
ELECTROCATALYTIC APPLICATION OF INTERMETALLICS
The subsequent section mainly discusses important electrocatalytic reactions catalyzed by intermetallics, including HER, ORR, CO2RR, and NRR. Readers who may seek detailed catalytic mechanisms of these electrocatalytic reactions are advised to consult several comprehensive reviews published elsewhere[107-109]. In this section, we do not intend to reiterate them, but rather concentrate on the developments of novel intermetallic electrocatalysts and structure-activity relationships.
Intermetallic electrocatalysts for HER
Water electrolysis is one of the promising technologies that can produce clean fuel hydrogen on a large scale. As a key half-reaction in water electrolysis, the HER at cathode usually requires an efficient electrocatalyst to reduce the reaction barrier and energy consumption. Noble metal Pt is readily recognized as the most active HER catalyst, but its high cost and scarcity hinder the wide commercialization of water electrolysis technology[110]. Alloying Pt with non-noble metals is an effective way to reduce Pt content and improve the intrinsic activity of electrocatalysts. In recent years, researchers have developed a series of Pt-based intermetallics, such as Pt3Ti[111], PtFe[112], PtNi[113], Pt3Co[114], and so on, whose ligand effects and stress effects between Pt and metal elements can regulate the surface adsorption properties, thus achieving the purpose of enhancing HER activity. For example, Zhang et al. reported an IrMo intermetallics supported on carbon nanotubes by a hydrogel-freeze-drying method, whose specific activity for HER was 9.5 folds that of Pt/C[115]. The stably-adsorbed OH spectator on the Mo site optimized the surface chemical environment and electronic structure of the catalyst, resulting in superior hydrogen adsorption energy and accelerating the water dissociation kinetics.
Developing non-Pt HER catalysts with Pt-like activity has been an important research direction in recent years. Especially, some noble metal-based intermetallics, such as Ru (RuB2, RuB, RuSi, RuGe, etc.),[93,116,117] Pd (Pd2B, PdSi, etc.)[118,119], Rh (RhB, RhSi, etc.)[120] and Ir (IrMo, IrSi, etc.)[121-123] have attracted wide attention. Our group studied a series of light element (e.g., Si, B)-containing intermetallics (silicide and boride), and found that the hybridization between sp orbital of light elements and d orbital of transition metals dominates the electronic structure characteristics [Figure 6A][96,118,124]. This sp-d orbital hybridization can reduce the hydrogen adsorption energy of metal surface and regulate the catalytic activity for HER [Figure 6B]. Based on these understandings, several non-Pt HER electrocatalysts with Pt-like activity, such as RuB and RuSi, have been confirmed by experiments and theoretical calculations [Figure 6B][96,118,124]. Inspired by these works, the discovery of new synthesis methods and novel electrocatalysts based on borides and silicide intermetallics has become a hot topic in recent years[120,121,125,126].
Figure 6. (A) Calculated pDOS of Pd and Pd-B alloy models; (B) A volcano plot showing the ΔGH* values of Pd-B alloy models containing different subsurface B concentrations as a function of their surface d-band centers. The inset shows the structural model of Pd-B systems with different subsurface B concentrations; (C) Schematic illustration of ordered interstitial B atom in Pd2B electrocatalysts and the optimal near-surface electronic structure for HER[118]; (D) Schematic illustration of the synthesis of ordered mesoporous PtZnM trimetals[128]; (E) Scanning electron microscopy (SEM) image of ordered mesoporous PtZnCo trimetals.
Compared with the disordered alloys, the intermetallic structures would not only enhance the catalytic activity, but also possess greater structural stability during HER. A remarkable example is Pd-B alloys reported by our group [Figure 6C][118]. Controlled preparation of intermetallic Pd2B and disordered Pd-B alloys was achieved by temperature control in a metallothermic reduction reaction. Despite a similar stoichiometric ratio, the intermetallic Pd2B exhibits significantly higher catalytic activity for HER than the disordered Pd-B alloy, because the interstitial boron ordering of the former yields an optimal near-surface electronic structure. Moreover, the catalytic and structural stability of intermetallic Pd2B is significantly enhanced relative to the disordered Pd-B alloy. (i) The leaching amount of B from Pd2B (1.4%) is less than that of disordered Pd-B alloy (13.3%) during HER. (ii) Pd2B possesses excellent catalytic stability for more than 7 days, while the disordered Pd-B alloy suffers severe deactivation.
In order to further reduce the amounts of noble metals and optimize catalytic performance in noble metal-based intermetallics, several important strategies have been developed. One strategy is to load noble metal intermetallics onto a suitable support, for example, IrGa supported on nitrogen-doped reduced graphene oxide,[127] Pt3Ti nanoparticles supported on Ti3C2Tx[111]. In these supported catalysts, in addition to reducing the amount of noble metals, the support effects can improve HER catalytic performance through metal-support electronic interaction. Another effective strategy to promote the catalytic performance of intermetallics is accurate nanostructure design. For example, Liu et al. prepared a series of ordered mesoporous PtZnM trimetals (M= Sc, V, Cr, Mn, Fe, Co, Ni, Cu or Ga) through a concurrent template route [Figure 6D and E][128]. Among them, the PtZnCo trimetals display a higher mass activity (0.36 A mgpt-1 at -0.05 V) for HER than that of commercial Pt/C (0.22 A mgpt-1) and strong catalytic stability for 50000 cycles, attributing to the large specific surface area and abundant low coordination active sites.
The ultimate pursuit of HER electrocatalysts is to develop highly active, noble metal-free catalysts. Intermetallic electrocatalysts based on Mo, W, Fe, Co, Ni and other non-noble metals have been widely studied, such as Gd2Co17[129], Co7Mo6[130], NiZn[131], and Co3W[132]. Although an enormous number of non-noble metal intermetallic electrocatalysts have been reported, it should be pointed out that their intrinsic activities for HER are usually 1-2 orders of magnitude lower than those of Pt-based catalysts[133]. Their high catalytic activities are often achieved at high loadings or/and large surface areas of catalysts to compensate for relatively worse intrinsic activity. Moreover, these non-noble metal electrocatalysts face a larger challenge in long-term catalytic stability relative to Pt/C catalysts.
Intermetallic electrocatalysts for ORR
ORR is an important half-reaction in fuel cell technology. The reaction largely determines the overall efficiency of fuel cells, because of multiple proton-electron transfer and sluggish reaction kinetics[134]. Similar to HER electrocatalysis, noble metal Pt is traditionally the most active electrocatalyst for ORR[43]. Alloying is an effective strategy to reduce the amount of Pt and increase the catalytic activity of ORR[135]. The strain and ligand effects in Pt alloys can influence the electronic structure and surface adsorption behavior, leading to greater catalytic activity for ORR. However, Pt alloys often suffer from poor stability, which is assigned to corrosion and leaching of transition metals during the catalytic process. The dissolved metal cations hinder proton conduction and accelerate the decomposition of the membrane and catalytic surface. Unlike the disordered Pt-M alloys, Pt-based intermetallics exhibit enhanced chemical stability in operating conditions due to ordered atomic arrangement and strong bonding interactions. The Pt-based intermetallics are expected to be new-generation ORR catalysts to realize low cost, greater catalytic activity and long-term durability simultaneously.
The exploration of Pt-based intermetallics as ORR electrocatalysts has progressively covered Pt-transition metal alloys (e.g., Pt3Ni, Pt3Co, Pt3Mn, Pt3Cu),[136-138] Pt-rare earth alloys (e.g., Pt3Y, Pt5La)[139-141], Pt-alkaline earth alloys (e.g., Pt5Ca and Pt5Sr)[142] and so on. A typical class of Pt-based intermetallic catalysts for ORR is binary Pt3M (M = 3d-transition metals). In 2007, Stamenkovic et al. demonstrated a volcano-type relationship between the surface electronic structure and ORR activity for Pt-transition metal alloys Pt3M (M=Ni, Co, Fe, Ti, V)[143]. The Pt3Ni and Pt3Fe are found to be at the very top of the volcano plot, exhibiting better ORR activities compared with pure Pt. In 2014, Yang et al. synthesized hollow Pt3Ni nanoframes with open structures and high surface areas, which displayed 36 times higher mass activity than Pt/C catalysts[144]. Subsequently, some doping and heterostructure strategies are developed to realize the component and structure optimization of Pt3Ni catalysts for ORR, such as Mo-doped Pt3Ni octahedron, Ni3C@Pt3Ni core-shell nanoparticles[145].
Constructing of ultrathin Pt shell on the surface of Pt-based intermetallic nanoalloys is an important strategy to improve the ORR activity. The formation of core-shell structure (such as PtPb/Pt, PtCo@Pt, etc.) can regulate the d-band center of the surface Pt atoms, thus changing the binding strength between the electrocatalyst surface and oxygen intermediates[138,146,147]. Recently, the Yang group proposed a Co3O4-assisted structural evolution strategy [Figure 7A] to controllably synthesize sub-6 nm core-shell Pt1Co1 intermetallic compound(Pt1Co1@Pt)[148]. Experiments and theoretical calculations showed that the ordered arrangement of Pt-Co atoms reduced the d-band center of surface Pt relative to the pure Pt and enhanced the oxidation resistance of Pt/Co sites, thus improving the ORR activity and durability. The proton exchange membrane fuel cell (PEMFC) using Pt1Co1@Pt as cathode catalyst layers exhibited a record power density (2.30/1.23 W cm-2 under H2-O2/air conditions) and extraordinary stability, making it the highest performance fuel cell of the time.
Figure 7. (A) Schematic illustration of the synthesis of Pt1Co1@Pt[148]; (B) Schematic illustration of the general synthesis of 46 Pt-based intermetallic nanoparticles on porous sulfur-doped carbon supports[77]; (C) The transmission electron microscopy (TEM) image of intermetallic PtCo nanoparticles; (D) Schematic illustration of the template-directed synthesis of porous intermetallic Pd-M nanosheets[153]; (E) The TEM image of intermetallic Pd3Pb nanosheets.
The synthesis of Pt-based intermetallics often requires high-temperature annealing to overcome the kinetic energy barrier of atomic ordering rearrangement in the solid phase. However, high-temperature synthesis inevitably leads to serious sintering of metal particles and a decrease in surface area, which ultimately results in low overall activity. It is highly desirable yet challenging to develop synthetic methods for small-sized Pt-based intermetallic catalysts. The Liang group developed a high-temperature sulfur anchoring synthesis strategy to construct an intermetallic library composed of 46 small-sized Pt-based intermetallic nanoparticles (< 5 nm), including 20 binary and 26 multicomponent intermetallics on sulfur-doped carbon supports [Figure 7B and C][77]. The strong interaction between Pt atoms and S atoms on carbon supports inhibits metal sintering during high-temperature annealing. Among them, the PtCo and PtNi catalysts showed mass activities of 1.52 and 1.84 A mgPt−1, which was much higher than commercial Pt/C catalyst (0.29 A mgPt−1) in H2-O2 cell tests at 0.9 volts. Subsequently, the Liang group realized the grams scale production of Pt-intermetallic catalysts on commercial carbon black supports by a small molecule-assisted impregnation method[84,149]. This work is an important step towards the practical application of nano-sized Pt-intermetallic catalysts.
Pd is considered the most suitable alternative to Pt catalyst for ORR, because Pd has similar physical and electrochemical properties to Pt and is relatively inexpensive. The ORR properties of Pd-based intermetallics have been widely studied, such as PdCu,[150] Pd3Pb[151], and Pd6Ni[152]. Huang et al. reported a template-directed rapid synthesis method [Figure 7D and E] for a series of Pd-based ultra-thin porous intermetallic Pd-M nanosheets (M = Pb, Sn and Cd)[153]. The mass activity and specific activity of Pd3Pb catalysts are 6.8 times and 9.8 times that of commercial Pt/C in the alkaline medium for ORR, respectively. Recently, Hu et al. reported a novel technique for the synthesis of intermetallic Pd3Pb nanoparticles by rapid joule heating within 60 s[154]. The short heating time can prevent particle aggregation, thus realizing the loading of ultra-small Pd3Pb nanoparticles with a narrow size distribution (6 nm) on carbon fiber supports. The synthesized Pd3Pb nanoparticles showed greater electrocatalytic activity for ORR than traditionally heated Pd3Pb.
Intermetallic electrocatalysts for CO2RR
Electrochemical CO2RR provides an attractive approach for the reduction of greenhouse gas emissions and the production of high-value-added industrial products[155-157]. The CO2RR in H2O electrolyte can be described as multiple steps of proton-coupled electron transfer and yields a range of different products, such as formates, CO, and hydrocarbons[158]. Many metal catalysts for CO2RR have been explored, which can be classified into three categories according to their main products: CO (Ag, Au, Zn, etc.), formates (Bi, Sn, Hg, In, etc.) ,and hydrocarbons (Cu)[159]. However, most monometallic catalysts are undesirable for CO2RR in terms of efficiency and selectivity. Intermetallic alloys provide unique electronic structures and well-defined coordination environments for precise tuning of catalytic activity and selectivity of CO2RR[21,28,29,153,160]. A broad range of nanostructured intermetallic catalysts has been developed for electrochemical CO2RR[161].
Compared to monometallic catalysts, intermetallic alloys can improve the catalytic activity. For example, Sn catalysts can only exhibit low CO2RR selectivity within a narrow potential window for formate generation, due to the large energy barriers and slow kinetics of the oxygen-breaking hydrogenation and desorption steps[162,163]. Tan et al. reported an ordered nanoporous intermetallic SnTe, which has better electrochemical CO2 to formate reduction performance compared to monometallic Sn catalysts[164]. This SnTe maintains more than 90% Faraday efficiency (FE) over a voltage range of -1.0 to -1.3 V (vs RHE). Combining operando Raman spectroscopy analysis with DFT calculation, it is found that the strong orbital interaction between Sn and neighboring Te in the intermetallic SnTe promotes bond breaking between metal and oxygen, which significantly increases the yield of formate. In addition, Jiang et al. synthesized a self-supported nanoporous Cu-Sn hybrid electrode, which is an intermetallic Cu3Sn in situ formed on Cu skeleton (Cu3Sn/Cu)[165]. Due to the enhanced adsorption ability of CO on Cu atoms, the intrinsic activity of Cu3Sn is 80 times higher than that of monometallic Cu, with a FE of 91.5% and excellent selectivity at an overpotential of 0.59 V.
Besides catalytic activity, the product selectivity of CO2RR can be changed by the rational construction of intermetallic catalysts. For example, Yang et al. investigated the effect of disorder-to-order transformation for AuCu bimetallic nanoparticles on their CO2RR selectivity [Figure 8A][166]. While the intermetallic AuCu electrocatalysts selectively converted CO2 to CO with a high Faraday efficiency of 80%, the disordered counterpart was mainly catalytically active for HER [Figure 8B and C]. DFT calculation and X-ray absorption analysis further confirmed that the increased activity and CO selectivity of ordered AuCu nanoparticles are due to the formation of a compressive strain Au shell layer over the AuCu intermetallic core. Another example is intermetallic Pd3Bi nanocrystals and chemically disordered Pd3Bi nanoparticles
Figure 8. (A) TEM images of AuCu bimetallic nanoparticles with different ordering degrees[166]; (B) Electrochemical CO2 reduction activities of AuCu nanoparticles evaluated by Faraday efficiencies of CO and H2; (C) Calculated limiting potentials for CO2 reduction and H2 evolution; (D) Schematic synthetic procedures for intermetallic Pd3Bi and disordered Pd3Bi alloys[167]; (E) Site-dependent projected partial density of states of Pd-4d in intermetallic Pd3Bi. (F) Crystal structure of CuGa2[168]; (G) Comparison of normalized XANES spectra of Ga K-edge of CuGa2 and Cu9Ga4 with the standard sample.
For intermetallic catalysts, phase structures have a significant effect on the electrocatalytic performance of CO2RR. Recently, Peter et al. prepared two intermetallic phases (CuGa2 and Cu9Ga4) formed by copper and gallium through a high-temperature solid-state reaction for electrocatalytic CO2RR [Figure 8F and G][168]. While the CuGa2 achieved a selective reduction of CO2 to methanol at a very low potential of -0.3 V with a Faraday efficiency of 77.26%, the Cu9Ga4 showed a much lower Faraday efficiency (37.75%) to produce methanol. The higher catalytic performance of CuGa2 is found to attribute to its unique two-dimensional structure, which preserves surface and subsurface oxide species (Ga2O3) in the reducing atmosphere.
Intermetallic electrocatalysts for NRR
Industrial ammonia synthesis relies substantially on the Haber-Bosch reaction, which involves a massive energy input and emits large amounts of greenhouse gases[169]. The electrochemical NRR under ambient conditions provides an attractive route to realize green ammonia synthesis. Efficient NRR electrocatalysts are highly required to break the inert nonpolar N≡N triple bond of a N2 molecule and to inhibit severe competition from the HER in H2O electrolyte[170-172]. Intermetallic nanocrystals have been widely investigated in the NRR field to exhibit excellent catalytic properties and good stability. The synergistic atomic interaction in intermetallic compounds can modulate the adsorption and desorption of N-containing intermediates while suppressing the competing process of HER, resulting in a lower reaction energy barrier and superior catalytic activity[51].
Many theoretical computational studies have been reported to accelerate the discovery of intermetallic catalysts with desirable NRR properties[19,173]. Yuan et al. calculated the N adsorption behavior on the surface of 29 intermetallics with Cu3Au-type structures[174]. Among the calculated intermetallics, Pd3Mo was considered as an attractive catalyst with a low limiting potential of -0.31 V and the ability to suppress the competing HER. Some theoretical studies have suggested that some unique structures of intermetallic compounds can provide ideal single-atom nonmetallic sites for efficient NRR. For example, the Qiao group simulated molybdenum boride models (e.g., Mo2B, α-MoB and MoB2) to explore their NRR activities[175]. Electronic structure studies showed that the occupancy of the hybridized orbital between the p-orbital of the boron site and the antibonding π*-orbital of N2 determined the binding strength of N2, so that the filling of the pz-orbital could be accepted as a descriptor of N2 activation [Figure 9A and B]. With increased boron content, the filling of pz-orbital increased in different Mo-B phases. The pz-orbital of Mo2B phase containing isolated boron sites is less filled, thus promoting the activation of N2. Moreover, this conception of isolated boron site is equally applicable in other metal borides in the form of M2B (M=Ti, Cr, Mn, Fe, Co, Ni, Ta, W). Due to the moderately filled pz-orbital and the high adsorption strength of the reaction intermediates, Mo2B, Fe2B and Co2B can be potential catalyst candidates for NRR.
Figure 9. (A) Schematic illustrations of hybridization interactions between the boron p-orbital of Mo2B and the π*-orbital of the N2 molecule; (B) Correlation between pz-orbital filling with ICOHP and adsorption energy of N2[175]; (C) Schematic illustrations of single-boron sites confined in antiperovskite borides for NRR[176]; (D) Schematic illustration of the synthesis of nanoporous intermetallic
Very recently, our group simulated anti-perovskite boride models with metal-coordinating single boron sites (M3M'B) to reveal the effect of a boron-centered local environment [Figure 9C] on the catalysis of
Apart from theoretical studies, it was confirmed by experimental studies that some intermetallic compounds can work as highly active NRR catalysts[177-179]. Tan et al. prepared nanoporous intermetallic Pd3Bi with strong Pd-Bi coupling by transforming chemically etched PdBi2 [Figure 9D and E][180]. The Bi sites in Pd3Bi can adsorb N2 molecules and lower the energy barrier of *N2 [Figure 9F]. Simultaneously, the bicontinuous nanoporous structure of the Pd3Bi can accelerate the electron transfer in NRR and further improve NRR performance. As a result, the Pd3Bi can exhibit a high NH3 yield rate of 59 µg h-1 mgcat-1 and a high Faraday efficiency of 21.5% at -0.2 V. Additionally, Sun et al. reported the preparation of Mo3Si thin films on graphite paper by the magnetron sputtering technique[181]. Electrochemical tests demonstrated that Mo3Si is an efficient NRR catalyst with high structural stability and exceptional selectivity for NH3 production. DFT calculation indicated that the exposed Mo ions show high chemical activity to facilitate the activation and adsorption of N2.
Although great achievements have been made, several unresolved questions still should be addressed in the catalytic application of intermetallics. (i) Current electrocatalytic studies of intermetallics mainly focus on HER and ORR. There is still insufficient research on CO2RR and NRR electrocatalysis of intermetallic catalysts. (ii) Mechanistic investigations are scant and unsystematic for intermetallic catalysts, which generally possess complex surface structures and diverse active site motifs. Combining advanced characterizations with density functional theory (DFT) calculation, an in‐depth understanding of structure‐performance relationships at the atomic and electronic levels is highly required. (iii) Although a variety of intermetallic catalysts have been developed, their high activities are measured by three-electrode system, and have rarely translated into electrochemical devices such as fuel cells and water electrolyzers. Thus, establishing reasonable performance evaluation criteria is essential to bridge the gap between three-electrode and device tests.
CONCLUSION AND OUTLOOK
Intermetallic compounds exhibit superior activity and stability compared to traditional disordered alloy catalysts, owing to their ordered atomic arrangement which allows for precise regulation of electronic structure and generation of new active sites. As a result, intermetallic compounds offer a promising platform for studying the structure-property relationships, including the geometric effects and electronic effects. This paper provides a clear definition of intermetallic compounds, and comprehensively summarizes the fundamental principles, common synthesis methods, and various electrocatalytic applications of intermetallic compounds. Despite the significant progress achieved in the synthesis and catalysis of ordered intermetallic compounds as reported in this paper, certain challenges must be overcome to realize the full potential of this novel material and meet the requirements of sustainable development.
The relationship between the structure and properties of intermetallic compounds is of critical importance for understanding their superior stability and activity in catalysis. However, during electrocatalysis, several significant structural changes, such as oxidation, surface reconstruction, disordered transformation, etc., may occur, leading to alterations in the catalytic active site and affecting the properties of intermetallic compounds[182]. To obtain a complete understanding of these structural changes, advanced in situ methods such as in situ X-ray photoelectron spectroscopy (XPS), SEM, TEM, and extended X-ray absorption fine structure (EXAFS) are necessary to obtain spatial and temporal information on the atomic positions on the catalyst surface and bulk. The obtained information can support theoretical calculations and provide insights into the complete catalytic process. A comprehensive understanding of these steps is essential to elucidate the structure-property relationship, comprehend the impact of the ordered structure of intermetallic compounds on catalytic performance, and advance the catalytic applications of intermetallic compounds.
Developing more accurate, controllable, and rapid synthesis strategies for intermetallic compounds is crucial to fully leverage their potential in catalysis. Intermetallic compounds are known for their high thermodynamic stability due to their ordered structure, but the process of transitioning from a disordered to an ordered structure involves overcoming a high kinetic energy barrier, and therefore, synthesis conditions such as temperature, carrier, and precursor must be carefully regulated to minimize agglomeration caused by high temperatures. Alternatively, the synthesis temperature can be reduced by lowering the kinetic energy barrier. For example, introducing appropriate vacancies may also facilitate the rearrangement of atoms during the synthesis process. Meanwhile, intermetallic compounds may contain a series of crystalline phases with close stoichiometric ratios, such as Pd2B, Pd5B2, and Pd3B[183,184]. Even for a particular crystalline phase, intermetallic compounds usually give rise to multiple exposure surfaces. It is still a challenge to accurately obtain the crystalline phase of the pure phase and a specific exposure surface. This hinders the construction of model catalysts for in-depth analysis of structure-activity relationships, and it is urgent to develop more selective synthesis methods. Additionally, although Gibbs free energy and Arrhenius equation can provide guidance for the formation of intermetallic compounds, the mechanism and kinetics of the formation of ordered structures are not yet clear. Therefore, improving the fundamental understanding of the synthesis mechanism is necessary to precisely adjust the synthesis parameters and achieve optimal synthesis conditions.
Exploring more potential intermetallic compounds is essential to leverage their advantages in catalysis fully. Many binary intermetallic compounds have attracted extensive research interest; however, the current understanding of intermetallic compounds is limited, and the candidate space for these materials remains significantly unexplored. For example, developing new non-precious metals or polymetallic intermetallic compounds is of great significance to reduce the use of precious metals and enable large-scale energy conversion in the future. Moreover, combining emerging high-throughput and machine learning methods can accelerate the discovery of potential candidate intermetallic compounds and facilitate the screening of properties of existing intermetallic compounds. High-throughput methods involve the rapid screening of a large number of materials, allowing for the exploration of a wider range of materials. Machine learning algorithms can then be used to analyze the large amount of data generated by these methods to identify promising candidates and predict their properties. By leveraging these tools, researchers can accelerate the discovery of new intermetallic compounds with desirable properties for catalysis and other applications. This approach has the potential to significantly increase our understanding of intermetallic compounds and enable the development of more efficient and cost-effective catalysts for a range of industrial processes.
DECLARATIONS
Authors’ contributionsPrepared the manuscript: Zhang M, Liu Q, Sun W, Sun K, Shen Y, An W, Zhang L
Performed manuscript correcting: Chen H, Zou X
Availability of data and materialsNot applicable.
Financial support and sponsorshipThis work was supported by the National Key R&D Program of China (2021YFB4000200) and the National Natural Science Foundation of China (NSFC) (21922507, 22179046).
Conflicts of interestAll authors declared that there are no conflicts of interest.
Ethical approval and consent to participateNot applicable.
Consent for publicationNot applicable.
Copyright© The Author(s) 2023.
REFERENCES
1. Chen T, Foo C, Edman Tsang SC. Interstitial and substitutional light elements in transition metals for heterogeneous catalysis. Chem Sci 2020;12:517-32.
2. Chen H, Zhang B, Liang X, Zou X. Light alloying element-regulated noble metal catalysts for energy-related applications. Chinese J Catal 2022;43:611-35.
3. Chen H, Zhang M, Wang Y, et al. Crystal phase engineering of electrocatalysts for energy conversions. Nano Res 2022;15:10194-217.
4. Liang J, Ma F, Hwang S, et al. Atomic arrangement engineering of metallic nanocrystals for energy-conversion electrocatalysis. Joule 2019;3:956-91.
5. Chen H, Wu Q, Wang Y, et al. Correction: d-sp orbital hybridization: a strategy for activity improvement of transition metal catalysts. Chem Commun 2022;58:7730-40.
6. Nakaya Y, Furukawa S. Catalysis of alloys: classification, principles, and design for a variety of materials and reactions. Chem Rev 2023;123:5859-947.
7. Zhou M, Li C, Fang J. Noble-Metal based random alloy and intermetallic nanocrystals: syntheses and applications. Chem Rev 2021;121:736-95.
8. Furukawa S, Komatsu T, Shimizu K. Catalyst design concept based on a variety of alloy materials: a personal account and relevant studies. J Mater Chem A 2020;8:15620-45.
9. Brown OW, Borland JB, Johnston RA, Grills RC. Catalytic activity of intermetallic compounds in the gas phase reduction of nitrobenzene. J Phys Chem 1939;43:805-7.
10. Berk B, Brown OW. Catalytic activity of intermetallic compounds in the vapor-phase reduction of nitrobenzene. II. J Phys Chem 1942;46:964-8.
12. Huq AKMS, Rosenberg AJ, Makrides AC. Electrochemical behavior of nickel compounds: II. Anodic dissolution and oxygen reduction in perchlorate solutions. J Electrochem Soc 1964;111:278.
13. Justi EW, Ewe HH, Kalberlah AW, Saridakis NM, Schaefer MH. Electrocatalysis in the nickel titanium system. Energy Conversion 1970;10:183-7.
14. Walter C, Menezes PW, Driess M. Perspective on intermetallics towards efficient electrocatalytic water-splitting. Chem Sci 2021;12:8603-31.
15. Miles MH. Evaluation of electrocatalysts for water electrolysis in alkaline solutions. J Electroanal Chem Interf Electrochem 1975;60:89-96.
16. Jakšić M. Electrocatalysis of hydrogen evolution in the light of the brewer - engel theory for bonding in metals and intermetallic phases. Electrochimica Acta 1984;29:1539-50.
17. Lu PWT, Srinivasan S. Nickel-based alloys as electrocatalysts for oxygen evolution from alkaline solutions. J Electrochem Soc 1978;125:265-70.
18. Katoh A, Uchida H, Shibata M, Watanabe M. Design of electrocatalyst for CO2 reduction: V . effect of the microcrystalline structures of Cu-Sn and Cu-Zn alloys on the electrocatalysis of CO2 reduction. J Electrochem Soc 1994;141:2054-8.
19. Abghoui Y, Garden AL, Howalt JG, Vegge T, Skúlason E. Electroreduction of N2 to ammonia at ambient conditions on mononitrides of Zr, Nb, Cr, and V: A DFT Guide for experiments. ACS Catal 2016;6:635-46.
20. Armbrüster M. Intermetallic compounds in catalysis - a versatile class of materials meets interesting challenges. Sci Technol Adv Mater 2020;21:303-22.
21. Rößner L, Armbrüster M. Electrochemical energy conversion on intermetallic compounds: a review. ACS Catal 2019;9:2018-62.
22. Chen X, Liang C. Transition metal silicides: fundamentals, preparation and catalytic applications. Catal Sci Technol 2019;9:4785-820.
23. Jothi PR, Yubuta K, Fokwa BPT. A simple, general synthetic route toward nanoscale transition metal borides. Adv Mater 2018;30:e1704181.
25. Kumar A, Dutta S, Kim S, et al. Solid-State reaction synthesis of nanoscale materials: strategies and applications. Chem Rev 2022;122:12748-863.
26. Li J, Sun S. Intermetallic nanoparticles: synthetic control and their enhanced electrocatalysis. Acc Chem Res 2019;52:2015-25.
27. Xiao W, Lei W, Gong M, Xin HL, Wang D. Recent advances of structurally ordered intermetallic nanoparticles for electrocatalysis. ACS Catal 2018;8:3237-56.
28. Yan Y, Du JS, Gilroy KD, Yang D, Xia Y, Zhang H. Intermetallic nanocrystals: syntheses and catalytic applications. Adv Mater 2017;29:1605997.
29. Furukawa S, Komatsu T. Intermetallic compounds: promising inorganic materials for well-structured and electronically modified reaction environments for efficient catalysis. ACS Catal 2017;7:735-65.
30. Menezes PW, Walter C, Hausmann JN, et al. Boosting water oxidation through in situ electroconversion of manganese gallide: an intermetallic precursor approach. Angew Chem Int Ed Engl 2019;58:16569-74.
31. Hausmann JN, Beltrán-Suito R, Mebs S, et al. Evolving highly active oxidic iron(III) phase from corrosion of intermetallic iron silicide to master efficient electrocatalytic water oxidation and selective oxygenation of 5-Hydroxymethylfurfural. Adv Mater 2021;33:e2008823.
32. Hume-Rothery W. Researches on the nature, properties, and conditions of formation of intermetallic compounds, with special reference to certain compounds of tin. J Inst Met 1926; 35:295-361.
33. Hume-Rothery W, Mabbott G, W, Channel Evans K. The freezing points, melting points, and solid solubility limits of the alloys of sliver and copper with the elements of the b sub-groups. Phil Trans R Soc Lond A 1934;233:1-97.
34. Mizutani U. The hume-rothery rules for structurally complex alloy phases. Surface properties and engineering of complex intermetallics. World scientific; 2010. pp. 323-99.
36. Yannello VJ, Fredrickson DC. Generality of the 18-n rule: intermetallic structural chemistry explained through isolobal analogies to transition metal complexes. Inorg Chem 2015;54:11385-98.
37. Fredrickson DC. Parallels in structural chemistry between the molecular and metallic realms revealed by complex intermetallic phases. Acc Chem Res 2018;51:248-57.
39. Schütz M, Gemel C, Klein W, Fischer RA, Fässler TF. Intermetallic phases meet intermetalloid clusters. Chem Soc Rev 2021;50:8496-510.
40. Miller GJ, Schmidt MW, Wang F, You T. Quantitative advances in the Zintl-Klemm formalism. In: Fässler TF, editor. Zintl Phases. Berlin: Springer Berlin Heidelberg; 2011. pp. 1-55.
41. Wang Y, He J, Liu C, Chong WH, Chen H. thermodynamics versus kinetics in nanosynthesis. Angew Chem Int Ed Engl 2015;54:2022-51.
42. Xia Y, Xiong Y, Lim B, Skrabalak SE. Shape-controlled synthesis of metal nanocrystals: simple chemistry meets complex physics? Angew Chem Int Ed Engl 2009;48:60-103.
44. You H, Yang S, Ding B, Yang H. Synthesis of colloidal metal and metal alloy nanoparticles for electrochemical energy applications. Chem Soc Rev 2013;42:2880-904.
45. Alloyeau D, Ricolleau C, Mottet C, et al. Size and shape effects on the order-disorder phase transition in CoPt nanoparticles. Nat Mater 2009;8:940-6.
47. Kayser FX, Patterson JW. Sir William Chandler Roberts-Austen - His role in the development of binary diagrams and modern physical metallurgy. JPE 1998;19:11-8.
48. Clarke SM, Amsler M, Walsh JPS, et al. Creating binary Cu-Bi compounds via high-pressure synthesis: a combined experimental and theoretical study. Chem Mater 2017;29:5276-85.
49. Terayama K, Tamura R, Nose Y, et al. Efficient construction method for phase diagrams using uncertainty sampling. Phys Rev Materials 2019:3.
50. Oliynyk AO, Mar A. Discovery of intermetallic compounds from traditional to machine-learning approaches. Acc Chem Res 2018;51:59-68.
51. Kim HY, Joo SH. Recent advances in nanostructured intermetallic electrocatalysts for renewable energy conversion reactions. J Mater Chem A 2020;8:8195-217.
53. Avrami M. Kinetics of phase change. II transformation-time relations for random distribution of nuclei. J Chem Phys 1940;8:212-24.
54. Avrami M. Granulation, phase change, and microstructure kinetics of phase change. III. J Chem Phys 1941;9:177-84.
55. Bai J, Yang L, Jin Z, Ge J, Xing W. Advanced Pt-based intermetallic nanocrystals for the oxygen reduction reaction. Chinese J Catal 2022;43:1444-58.
56. Zhang J, Zhang L, Cui Z. Strategies to enhance the electrochemical performances of Pt-based intermetallic catalysts. Chem Commun 2021;57:11-26.
57. Zhang S, Guo S, Zhu H, Su D, Sun S. Structure-induced enhancement in electrooxidation of trimetallic FePtAu nanoparticles. J Am Chem Soc 2012;134:5060-3.
58. Zhang S, Qi W, Huang B. Size effect on order-disorder transition kinetics of FePt nanoparticles. J Chem Phys 2014;140:044328.
59. Tzitzios V, Basina G, Gjoka M, et al. The effect of Mn doping in FePt nanoparticles on the magnetic properties of the L10 phase. Nanotechnology 2006;17:4270-3.
60. Qi W, Li Y, Xiong S, Lee ST. Modeling size and shape effects on the order-disorder phase-transition temperature of CoPt nanoparticles. Small 2010;6:1996-9.
61. Oezaslan M, Heggen M, Strasser P. Size-dependent morphology of dealloyed bimetallic catalysts: linking the nano to the macro scale. J Am Chem Soc 2012;134:514-24.
62. Kim SI, Eom G, Kang M, et al. Composition-selective fabrication of ordered intermetallic Au-Cu nanowires and their application to nano-size electrochemical glucose detection. Nanotechnology 2015; 26:245702.
63. Dai ZR, Sun S, Wang ZL. Phase transformation, coalescence, and twinning of monodisperse fept nanocrystals. Nano Lett 2001;1:443-7.
64. Supansomboon S, Dowd A, Gentle A, van der Lingen E, Cortie M. Thin films of PtAl2 and AuAl2 by solid-state reactive synthesis. Thin Solid Films 2015;589:805-12.
65. Kondoh K, Oginuma H, Kimura A, Matsukawa S, Aizawa T.
66. Winiarski M, Griveau J, Colineau E, et al. Synthesis and properties of AxV2Al20 (A = Th, U, Np, Pu) ternary actinide aluminides. J Alloys Compd 2017; 696:1113-9.
67. Shablinskaya K, Murashova E, Tursina A, Kurenbaeva Z, Yaroslavtsev A, Seropegin Y. Intermetallics La9Ru4In5 and Ce9Ru4Ga5 with new types of structures. Synthesis, crystal structures, physical properties. Intermetallics 2012;23:106-10.
68. Fernandes BB, Ramos ECT, Silva G, Ramos AS. Preparation of Nb-25Si, Nb-37.5Si, Nb-66.6Si powders by high-energy ball milling and subsequent heat treatment. J Alloys Compd 2007;434-435:509-13.
69. Alanko GA, Jaques B, Bateman A, Butt DP. Mechanochemical synthesis and spark plasma sintering of the cerium silicides. J Alloys Compd 2014;616:306-11.
70. Wang Z, Liu J, Wu X, et al. Engineering ordered vacancies and atomic arrangement over the intermetallic PdM/CNT (M = Pb, Sn, In) nanocatalysts for synergistically promoting electrocatalysis N2 fixation. Appl. Catal. B Environ 2022;314:121465.
71. Abe H, Matsumoto F, Alden LR, Warren SC, Abruña HD, DiSalvo FJ. Electrocatalytic performance of fuel oxidation by Pt3Ti nanoparticles. J Am Chem Soc 2008;130:5452-8.
72. Chi M, Wang C, Lei Y, et al. Surface faceting and elemental diffusion behaviour at atomic scale for alloy nanoparticles during in situ annealing. Nat Commun 2015;6:8925.
73. Yu W, Zhang Y, Qin Y, et al. High‐Density frustrated lewis pair for high-performance hydrogen evolution. Adv. Energy Mater 2023;13:2203136.
74. Yoo TY, Yoo JM, Sinha AK, et al. Direct synthesis of intermetallic platinum-alloy nanoparticles highly loaded on carbon supports for efficient electrocatalysis. J Am Chem Soc 2020;142:14190-200.
75. Chen D, Li Z, Zhou Y, et al. Fe3Pt intermetallic nanoparticles anchored on N-doped mesoporous carbon for the highly efficient oxygen reduction reaction. Chem Commun 2020;56:4898-901.
76. Shen T, Gong M, Xiao D, et al. Engineering location and supports of atomically ordered L10-PdFe intermetallics for ultra-anticorrosion electrocatalysis. Adv Funct Materials 2022;32:2203921.
77. Yang CL, Wang LN, Yin P, et al. Sulfur-anchoring synthesis of platinum intermetallic nanoparticle catalysts for fuel cells. Science 2021;374:459-64.
78. Ji X, Lee KT, Holden R, et al. Nanocrystalline intermetallics on mesoporous carbon for direct formic acid fuel cell anodes. Nat Chem 2010;2:286-93.
79. He W, Zhang X, Zheng K, et al. Structural evolution of anatase-supported platinum nanoclusters into a platinum-titanium intermetallic containing platinum single atoms for enhanced catalytic co oxidation. Angew Chem Int Ed Engl 2023;62:e202213365.
80. Bernal S, Calvino J, Gatica J, Larese C, López-cartes C, Pérez-omil J. Nanostructural evolution of a Pt/CeO2Catalyst reduced at increasing temperatures (473-1223 k): a hrem study. J Catal 1997;169:510-5.
81. Maligal-ganesh RV, Xiao C, Goh TW, et al. A ship-in-a-bottle strategy to synthesize encapsulated intermetallic nanoparticle catalysts: exemplified for furfural hydrogenation. ACS Catal 2016;6:1754-63.
82. Takahashi Y, Kadono T, Yamamoto S, et al. Orbital magnetic moment and coercivity of SiO2 -coated FePt nanoparticles studied by x-ray magnetic circular dichroism. Phys Rev B 2014:90.
83. Kim J, Rong C, Liu JP, Sun S. Dispersible ferromagnetic fept nanoparticles. Adv Mater 2009;21:906-9.
84. Song TW, Xu C, Sheng ZT, et al. Small molecule-assisted synthesis of carbon supported platinum intermetallic fuel cell catalysts. Nat Commun 2022;13:6521.
85. Chen H, Yu Y, Xin HL, et al. Coalescence in the thermal annealing of nanoparticles: an in situ STEM study of the growth mechanisms of ordered Pt-Fe nanoparticles in a KCL matrix. Chem Mater 2013;25:1436-42.
86. Chen H, Wang D, Yu Y, et al. A surfactant-free strategy for synthesizing and processing intermetallic platinum-based nanoparticle catalysts. J Am Chem Soc 2012;134:18453-9.
87. Wang Z, Wu X, Liu J, et al. Ordered Vacancies on the body-centered cubic PdCu nanocatalysts. Nano Lett 2021;21:9580-6.
88. Meng C, Zhao G, Shi XR, Chen P, Liu Y, Lu Y. Oxygen-deficient metal oxides supported nano-intermetallic InNi3C(0.5) toward efficient CO2 hydrogenation to methanol. Sci Adv 2021;7:32.
89. Li Q, Wu L, Wu G, et al. New approach to fully ordered fct-FePt nanoparticles for much enhanced electrocatalysis in acid. Nano Lett 2015;15:2468-73.
90. Kim J, Rong C, Lee Y, Liu JP, Sun S. From core/shell structured FePt/Fe3O4 /MgO to ferromagnetic FePt nanoparticles. Chem Mater 2008;20:7242-5.
91. Wang T, Liang J, Zhao Z, et al. Sub-6 nm Fully Ordered L10-Pt-Ni-Co Nanoparticles enhance oxygen reduction via Co doping induced ferromagnetism enhancement and optimized surface strain. Adv Energy Mater 2019;9:1803771.
92. Chen H, Zhang M, Zhang K, et al. Screening and understanding lattice silicon-controlled catalytically active site motifs from a library of transition metal-silicon intermetallics. Small 2022;18:e2107371.
93. Chen H, Zou X. Intermetallic borides: structures, synthesis and applications in electrocatalysis. Inorg Chem Front 2020;7:2248-64.
94. Guo F, Wu Y, Ai X, et al. A class of metal diboride electrocatalysts synthesized by a molten salt-assisted reaction for the hydrogen evolution reaction. Chem Commun 2019;55:8627-30.
95. Li Q, Zou X, Ai X, Chen H, Sun L, Zou X. Revealing activity trends of metal diborides toward pH-universal hydrogen evolution electrocatalysts with Pt-like activity. Adv Energy Mater 2018;9:1803369.
96. Ai X, Zou X, Chen H, et al. Transition-Metal-Boron intermetallics with strong interatomic d-sp orbital hybridization for high-performance electrocatalysis. Angew Chem Int Ed Engl 2020;59:3961-5.
97. Yuan Y, Yang Z, Lai W, et al. Intermetallic compounds: liquid-phase synthesis and electrocatalytic applications. Chemistry 2021;27:16564-80.
98. Rong H, Mao J, Xin P, et al. Kinetically controlling surface structure to construct defect-rich intermetallic nanocrystals: effective and stable catalysts. Adv Mater 2016;28:2540-6.
99. Liao H, Zhu J, Hou Y. Synthesis and electrocatalytic properties of PtBi nanoplatelets and PdBi nanowires. Nanoscale 2014;6:1049-55.
100. Maksimuk S, Yang S, Peng Z, Yang H. Synthesis and characterization of ordered intermetallic PtPb nanorods. J Am Chem Soc 2007;129:8684-5.
101. Cable RE, Schaak RE. Low-Temperature solution synthesis of nanocrystalline binary intermetallic compounds using the polyol process. Chem Mater 2005;17:6835-41.
102. Guo J, Jiao S, Ya X, et al. Intermetallic nanocrystals: seed-mediated synthesis and applications in electrocatalytic reduction reactions. Chemistry 2022;28:e202202221.
103. Samanta A, Das S, Jana S. Ultra-small intermetallic NiZn nanoparticles: a non-precious metal catalyst for efficient electrocatalysis. Nanoscale Adv 2020;2:417-24.
104. Bu L, Zhang N, Guo S, et al. Biaxially strained PtPb/Pt core/shell nanoplate boosts oxygen reduction catalysis. Science 2016;354:1410-4.
105. Chen W, Yu R, Li L, Wang A, Peng Q, Li Y. A seed-based diffusion route to monodisperse intermetallic CuAu nanocrystals. Angew Chem Int Ed Engl 2010;49:2917-21.
106. Clarysse J, Moser A, Yarema O, Wood V, Yarema M. Size- and composition-controlled intermetallic nanocrystals via amalgamation seeded growth. Sci Adv 2021:7.
107. Chen H, Liang X, Liu Y, Ai X, Asefa T, Zou X. Active site engineering in porous electrocatalysts. Adv Mater 2020;32:e2002435.
108. Zhang M, Zhang K, Ai X, et al. Theory-guided electrocatalyst engineering: From mechanism analysis to structural design. Chinese J Catal 2022;43:2987-3018.
109. Seh ZW, Kibsgaard J, Dickens CF, Chorkendorff I, Nørskov JK, Jaramillo TF. Combining theory and experiment in electrocatalysis: insights into materials design. Science 2017; 355:eaad4998.
110. Chatenet M, Pollet BG, Dekel DR, et al. Water electrolysis: from textbook knowledge to the latest scientific strategies and industrial developments. Chem Soc Rev 2022;51:4583-762.
111. Li Z, Qi Z, Wang S, et al. In Situ Formed Pt3Ti Nanoparticles on a Two-Dimensional Transition Metal Carbide (MXene) used as efficient catalysts for hydrogen evolution reactions. Nano Lett 2019;19:5102-8.
112. Hu M, Cai Z, Yang S, et al. Direct growth of uniform bimetallic core-shell or intermetallic nanoparticles on carbon via a surface-confinement strategy for electrochemical hydrogen evolution reaction. Adv Funct Materials 2023;33:2212097.
113. Zhao P, Zhang B, Hao X, Yi W, Chen J, Cao Q. Rational design and synthesis of adjustable Pt and Pt-based 3D-nanoframeworks. ACS Appl Energy Mater 2022;5:942-50.
114. Lin C, Huang Z, Zhang Z, et al. Structurally ordered Pt3Co nanoparticles anchored on N-Doped graphene for highly efficient hydrogen evolution reaction. ACS Sustainable Chem Eng 2020;8:16938-45.
115. Zhang J, Zhang L, Liu J, et al. OH spectator at IrMo intermetallic narrowing activity gap between alkaline and acidic hydrogen evolution reaction. Nat Commun 2022;13:5497.
116. Zou X, Wang L, Ai X, Chen H, Zou X. Crystal phase-dependent electrocatalytic hydrogen evolution performance of ruthenium-boron intermetallics. Chem Commun 2020;56:3061-4.
117. Chen D, Zhu J, Pu Z, Mu S. Anion modulation of Pt-group metals and electrocatalysis applications. Chemistry 2021;27:12257-71.
118. Li Z, Xie Z, Chen H, et al. Realization of interstitial boron ordering and optimal near-surface electronic structure in Pd-B alloy electrocatalysts. J Chem Eng 2021;419:129568.
119. Ren Z, Jiang H, Yuan M, et al. Si regulation of hydrogen adsorption on nanoporous PdSi hybrids towards enhancing electrochemical hydrogen evolution activity. Inorg Chem Front 2023;10:1101-11.
120. Pu Z, Liu T, Zhang G, et al. General synthesis of Transition-Metal-Based Carbon-Group Intermetallic catalysts for efficient electrocatalytic hydrogen evolution in wide pH range. Adv. Energy Mater 2022;12:2200293.
121. Chen D, Pu Z, Wang P, et al. Mapping hydrogen evolution activity trends of intermetallic Pt-group silicides. ACS Catal 2022;12:2623-31.
122. Fu L, Li Y, Yao N, Yang F, Cheng G, Luo W. IrMo nanocatalysts for efficient alkaline hydrogen electrocatalysis. ACS Catal 2020;10:7322-7.
123. Chen L, Guo X, Shao R, et al. Structurally ordered intermetallic Ir3V electrocatalysts for alkaline hydrogen evolution reaction. Nano Energy 2021;81:105636.
124. Chen H, Ai X, Liu W, et al. Promoting subordinate, efficient ruthenium sites with interstitial silicon for Pt-like electrocatalytic activity. Angew Chem Int Ed Engl 2019;58:11409-13.
125. Shen S, Hu Z, Zhang H, et al. Highly active Si sites enabled by negative valent ru for electrocatalytic hydrogen evolution in LaRuSi. Angew Chem Int Ed Engl 2022;61:e202206460.
126. He Y, Wang TL, Zhang M, et al. Discovery and facile synthesis of a new silicon based family as efficient hydrogen evolution reaction catalysts: a computational and experimental investigation of metal monosilicides. Small 2021;17:e2006153.
127. Zhang H, Shi P, Ma X, et al. Construction of ordered atomic donor-acceptor architectures in bcc IrGa intermetallic compounds toward highly electroactive and stable overall water splitting. Adv. Energy Mater 2023;13:2202703.
128. Wang Y, Lv H, Sun L, Jia F, Liu B. Ordered mesoporous intermetallic trimetals for efficient and pH-Universal hydrogen evolution electrocatalysis. Adv. Energy Mate ;12:2201478.
129. Ji SJ, Xue HG, Suen NT. Lanthanide contraction regulates the HER activity of iron triad intermetallics in alkaline media. Chem Commun 2020;56:14303-6.
130. Song R, Han J, Okugawa M, et al. Ultrafine nanoporous intermetallic catalysts by high-temperature liquid metal dealloying for electrochemical hydrogen production. Nat Commun 2022;13:5157.
131. Zhou Q, Hao Q, Li Y, et al. Free-standing trimodal porous NiZn intermetallic and Ni heterojunction as highly efficient hydrogen evolution electrocatalyst in the alkaline electrolyte. Nano Energy 2021;89:106402.
132. Li Y, Lu W, Du Y, et al. Co3W intermetallic compound as an efficient hydrogen evolution electrocatalyst for water splitting and electrocoagulation in non-acidic media. J Chem Eng 2022;438:135517.
133. Kibsgaard J, Chorkendorff I. Considerations for the scaling-up of water splitting catalysts. Nat Energy 2019;4:430-3.
134. Shao M, Chang Q, Dodelet JP, Chenitz R. Recent advances in electrocatalysts for oxygen reduction reaction. Chem Rev 2016;116:3594-657.
135. Bing Y, Liu H, Zhang L, Ghosh D, Zhang J. Nanostructured Pt-alloy electrocatalysts for PEM fuel cell oxygen reduction reaction. Chem Soc Rev 2010;39:2184-202.
136. Lim J, Jung C, Hong D, et al. Atomically ordered Pt3Mn intermetallic electrocatalysts for the oxygen reduction reaction in fuel cells. J Mater Chem A 2022;10:7399-408.
137. Tetteh EB, Gyan-Barimah C, Lee HY, et al. Strained Pt(221)
138. Guan J, Yang S, Liu T, et al. Intermetallic FePt@PtBi core-shell nanoparticles for oxygen reduction electrocatalysis. Angew Chem Int Ed Engl 2021;60:21899-904.
139. Brown R, Vorokhta M, Khalakhan I, et al. Unraveling the surface chemistry and structure in highly active sputtered Pt3Y Catalyst films for the oxygen reduction reaction. ACS Appl Mater Interfaces 2020;12:4454-62.
140. Peera SG, Lee TG, Sahu AK. Pt-rare earth metal alloy/metal oxide catalysts for oxygen reduction and alcohol oxidation reactions: an overview. Sustain Energy Fuels 2019;3:1866-91.
141. Zhu S, Yang L, Bai J, et al. Ultra-stable Pt5La intermetallic compound towards highly efficient oxygen reduction reaction. Nano Res 2023;16:2035-40.
142. Vej-hansen UG, Escudero-escribano M, Velázquez-palenzuela A, et al. New platinum alloy catalysts for oxygen electroreduction based on alkaline earth metals. Electrocatalysis 2017;8:594-604.
143. Stamenkovic VR, Mun BS, Arenz M, et al. Trends in electrocatalysis on extended and nanoscale Pt-bimetallic alloy surfaces. Nat Mater 2007;6:241-7.
144. Chen C, Kang Y, Huo Z, et al. Highly crystalline multimetallic nanoframes with three-dimensional electrocatalytic surfaces. Science 2014;343:1339-43.
145. Ding H, Wang P, Su C, et al. Epitaxial growth of ultrathin highly crystalline Pt-Ni nanostructure on a metal carbide template for efficient oxygen reduction reaction. Adv Mater 2022;34:e2109188.
146. Weber P, Weber DJ, Dosche C, Oezaslan M. Highly durable Pt-based core-shell catalysts with metallic and oxidized Co species for boosting the oxygen reduction reaction. ACS Catal 2022;12:6394-408.
147. Shi W, Park A, Kwon Y. Scalable synthesis of (Pd,Cu)@Pt core-shell catalyst with high ORR activity and durability. J Electroanal Chem 2022;918:116451.
148. Cheng Q, Yang S, Fu C, et al. High-loaded sub-6 nm Pt1Co1 intermetallic compounds with highly efficient performance expression in PEMFCs. Energy Environ Sci 2022;15:278-86.
149. Zeng WJ, Wang C, Yan QQ, Yin P, Tong L, Liang HW. Phase diagrams guide synthesis of highly ordered intermetallic electrocatalysts: separating alloying and ordering stages. Nat Commun 2022;13:7654.
150. Wu Z, Shan S, Xie Z, et al. Revealing the role of phase structures of bimetallic nanocatalysts in the oxygen reduction reaction. ACS Catal 2018;8:11302-13.
151. Gamler JTL, Shin K, Ashberry HM, et al. Intermetallic Pd3Pb nanocubes with high selectivity for the 4-electron oxygen reduction reaction pathway. Nanoscale 2020;12:2532-41.
152. Feng Y, Shao Q, Ji Y, et al. Surface-modulated palladium-nickel icosahedra as high-performance non-platinum oxygen reduction electrocatalysts. Sci Adv 2018;4:eaap8817.
153. Guo J, Gao L, Tan X, et al. Template-Directed rapid synthesis of Pd-based ultrathin porous intermetallic nanosheets for efficient oxygen reduction. Angew Chem Int Ed Engl 2021;60:10942-9.
154. Cui M, Yang C, Hwang S, et al. Multi-principal elemental intermetallic nanoparticles synthesized via a disorder-to-order transition. Sci Adv 2022;8:eabm4322.
155. Birdja YY, Pérez-gallent E, Figueiredo MC, Göttle AJ, Calle-vallejo F, Koper MTM. Advances and challenges in understanding the electrocatalytic conversion of carbon dioxide to fuels. Nat Energy 2019;4:732-45.
156. Ross MB, De Luna P, Li Y, et al. Designing materials for electrochemical carbon dioxide recycling. Nat Catal 2019;2:648-58.
157. Gao D, Arán-ais RM, Jeon HS, Roldan Cuenya B. Rational catalyst and electrolyte design for CO2 electroreduction towards multicarbon products. Nat Catal 2019;2:198-210.
158. Nitopi S, Bertheussen E, Scott SB, et al. Progress and perspectives of electrochemical CO2 reduction on copper in aqueous electrolyte. Chem Rev 2019;119:7610-72.
159. Kim C, Dionigi F, Beermann V, Wang X, Möller T, Strasser P. Alloy Nanocatalysts for the electrochemical oxygen reduction (ORR) and the direct electrochemical carbon dioxide reduction reaction (CO2RR). Adv Mater 2019;31:e1805617.
160. Gamler JTL, Ashberry HM, Skrabalak SE, Koczkur KM. Random alloyed versus intermetallic nanoparticles: a comparison of electrocatalytic performance. Adv Mater 2018:e1801563.
161. Kortlever R, Peters I, Koper S, Koper MTM. Electrochemical CO2 reduction to formic acid at low overpotential and with high faradaic efficiency on carbon-supported bimetallic Pd-Pt Nanoparticles. ACS Catal 2015;5:3916-23.
162. Fan L, Xia C, Zhu P, Lu Y, Wang H. Electrochemical CO2 reduction to high-concentration pure formic acid solutions in an all-solid-state reactor. Nat Commun 2020;11:3633.
163. He B, Jia L, Cui Y, et al. SnSe2 nanorods on carbon cloth as a highly selective, active, and flexible electrocatalyst for electrochemical reduction of CO2 into formate. ACS Appl Energy Mater 2019;2:7655-62.
164. Yang Q, Zhao Y, Meng L, et al. Nanoporous intermetallic snte enables efficient electrochemical CO2 reduction into formate via promoting the fracture of metal-oxygen bonding. Small 2022;18:e2107968.
165. Wan WB, Zhou YT, Zeng SP, et al. Nanoporous intermetallic Cu3Sn/Cu Hybrid electrodes as efficient electrocatalysts for carbon dioxide reduction. Small 2021;17:e2100683.
166. Kim D, Xie C, Becknell N, et al. Electrochemical activation of CO2 through atomic ordering transformations of AuCu nanoparticles. J Am Chem Soc 2017;139:8329-36.
167. Jia L, Sun M, Xu J, et al. Phase-Dependent electrocatalytic CO2 reduction on Pd3Bi nanocrystals. Angew Chem Int Ed Engl 2021;60:21741-5.
168. Bagchi D, Raj J, Singh AK, et al. Structure-Tailored surface oxide on Cu-Ga intermetallics enhances CO2 reduction selectivity to methanol at ultralow potential. Adv Mater 2022;34:e2109426.
169. Liu D, Chen M, Du X, et al. Development of electrocatalysts for efficient nitrogen reduction reaction under ambient condition. Adv Funct Mater 2021;31:2008983.
170. Chen X, Guo Y, Du X, et al. Atomic structure modification for electrochemical nitrogen reduction to ammonia. Adv Energy Mater 2020;10:1903172.
171. Montoya JH, Tsai C, Vojvodic A, Nørskov JK. The Challenge of electrochemical ammonia synthesis: a new perspective on the role of nitrogen scaling relations. ChemSusChem 2015;8:2180-6.
172. Zhao J, Chen Z. Single mo atom supported on defective boron nitride monolayer as an efficient electrocatalyst for nitrogen fixation: a computational study. J Am Chem Soc 2017;139:12480-7.
173. Abghoui Y, Garden AL, Hlynsson VF, Björgvinsdóttir S, Ólafsdóttir H, Skúlason E. Enabling electrochemical reduction of nitrogen to ammonia at ambient conditions through rational catalyst design. Phys Chem Chem Phys 2015;17:4909-18.
174. Zhou J, Chen X, Guo M, Hu W, Huang B, Yuan D. Enhanced catalytic activity of bimetallic ordered catalysts for nitrogen reduction reaction by perturbation of scaling relations. ACS Catal 2023;13:2190-201.
175. Liu X, Jiao Y, Zheng Y, Qiao S. Isolated boron sites for electroreduction of dinitrogen to ammonia. ACS Catal 2020;10:1847-54.
176. Ai X, Chen H, Liang X, et al. Metal-coordinating single-boron sites confined in antiperovskite borides for N2-to-NH3 catalytic conversion. ACS Catal 2022;12:2967-78.
177. Guo J, Wang H, Xue F, et al. Tunable synthesis of multiply twinned intermetallic Pd3Pb nanowire networks toward efficient N2 to NH3 conversion. J Mater Chem A 2019;7:20247-53.
178. Tong W, Huang B, Wang P, Shao Q, Huang X. Exposed facet-controlled N2 electroreduction on distinct Pt3Fe nanostructures of nanocubes, nanorods and nanowires. Natl Sci Rev 2021;8:nwaa088.
179. Chu K, Gu W, Li Q, Liu Y, Tian Y, Liu W. Amorphization activated FeB2 porous nanosheets enable efficient electrocatalytic N2 fixation. J Energy Chem 2021;53:82-9.
180. Wang X, Luo M, Lan J, Peng M, Tan Y. Nanoporous intermetallic Pd3 Bi for efficient electrochemical nitrogen reduction. Adv Mater 2021;33:e2007733.
181. Wang T, Liu Q, Li T, et al. A magnetron sputtered Mo3Si thin film: an efficient electrocatalyst for N2 reduction under ambient conditions. J Mater Chem A 2021;9:884-8.
182. Li X, Zhao J, Su D. Structural Changes of intermetallic catalysts under reaction conditions. Small Structures 2021;2:2100011.
183. Li Z, Zhao L, Chen H, et al. Crystal phase-selective synthesis of intermetallic palladium borides and their phase-regulated (electro)catalytic properties. Catal Sci Technol 2022;12:1038-42.
Cite This Article
How to Cite
Download Citation
Export Citation File:
Type of Import
Tips on Downloading Citation
Citation Manager File Format
Type of Import
Direct Import: When the Direct Import option is selected (the default state), a dialogue box will give you the option to Save or Open the downloaded citation data. Choosing Open will either launch your citation manager or give you a choice of applications with which to use the metadata. The Save option saves the file locally for later use.
Indirect Import: When the Indirect Import option is selected, the metadata is displayed and may be copied and pasted as needed.
About This Article
Copyright
Author Biographies
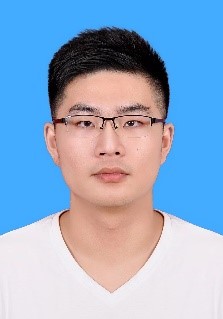
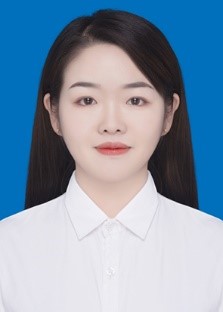
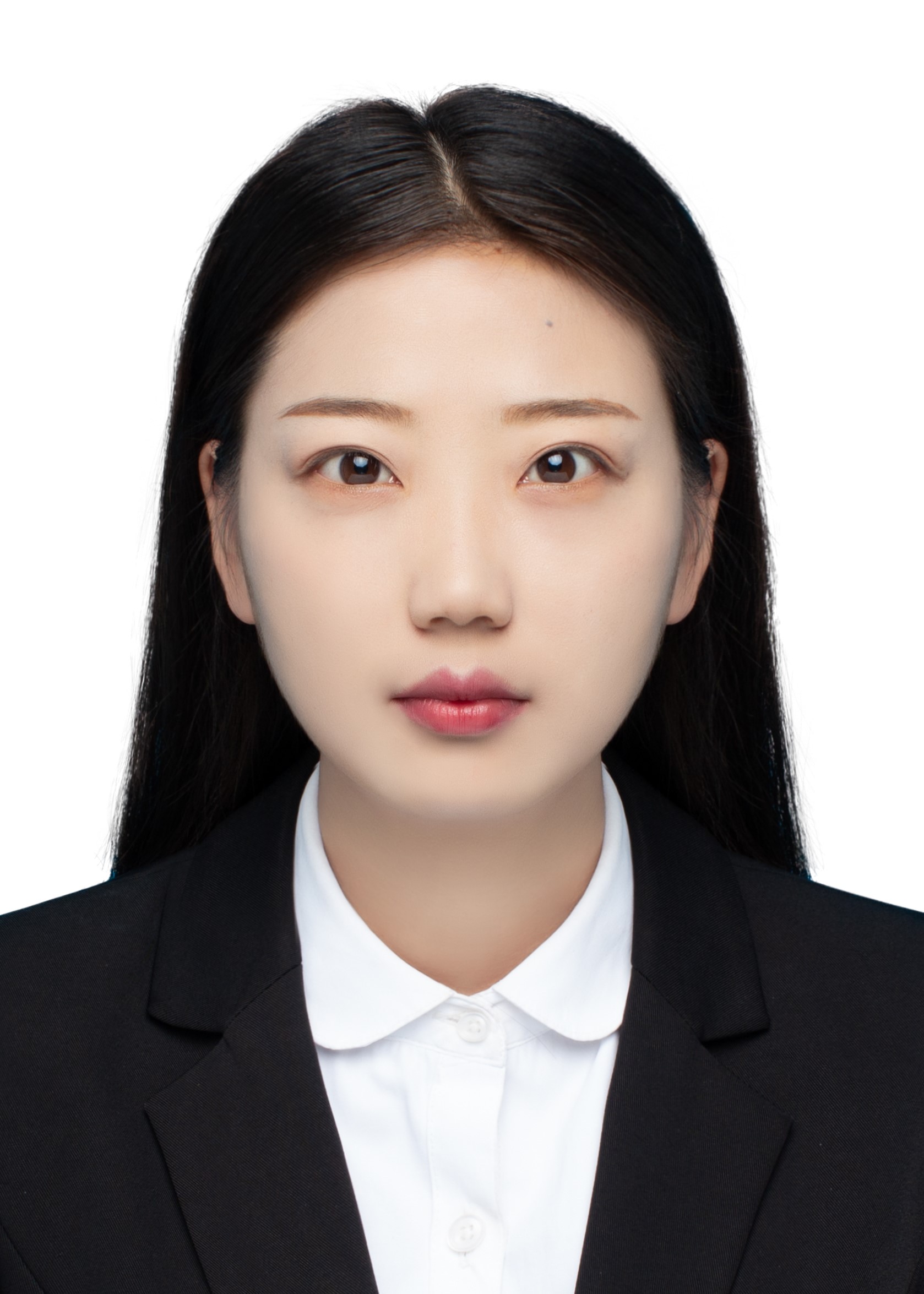
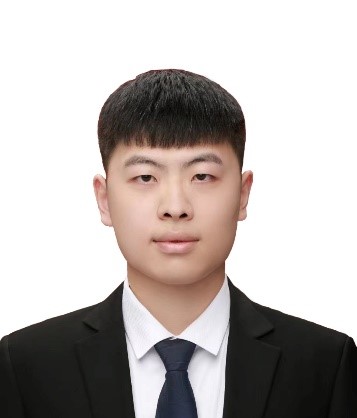
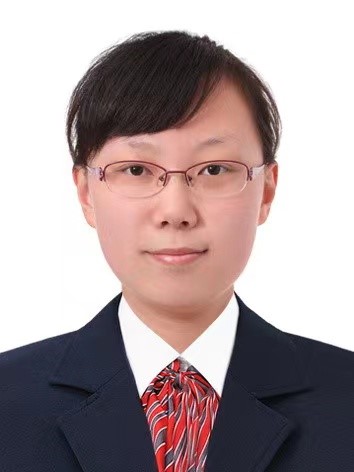
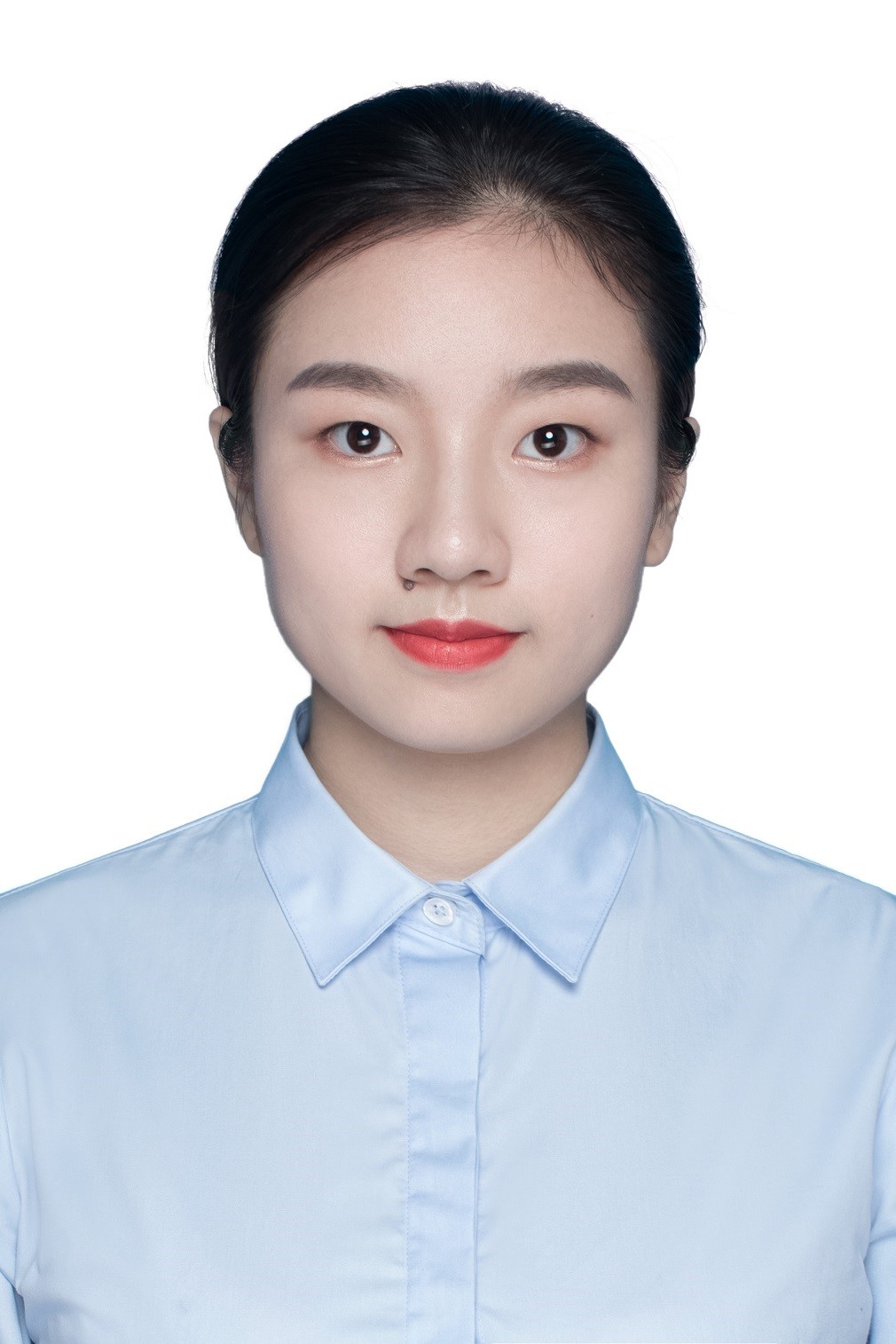
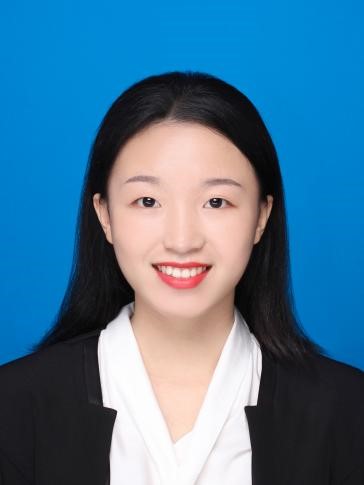
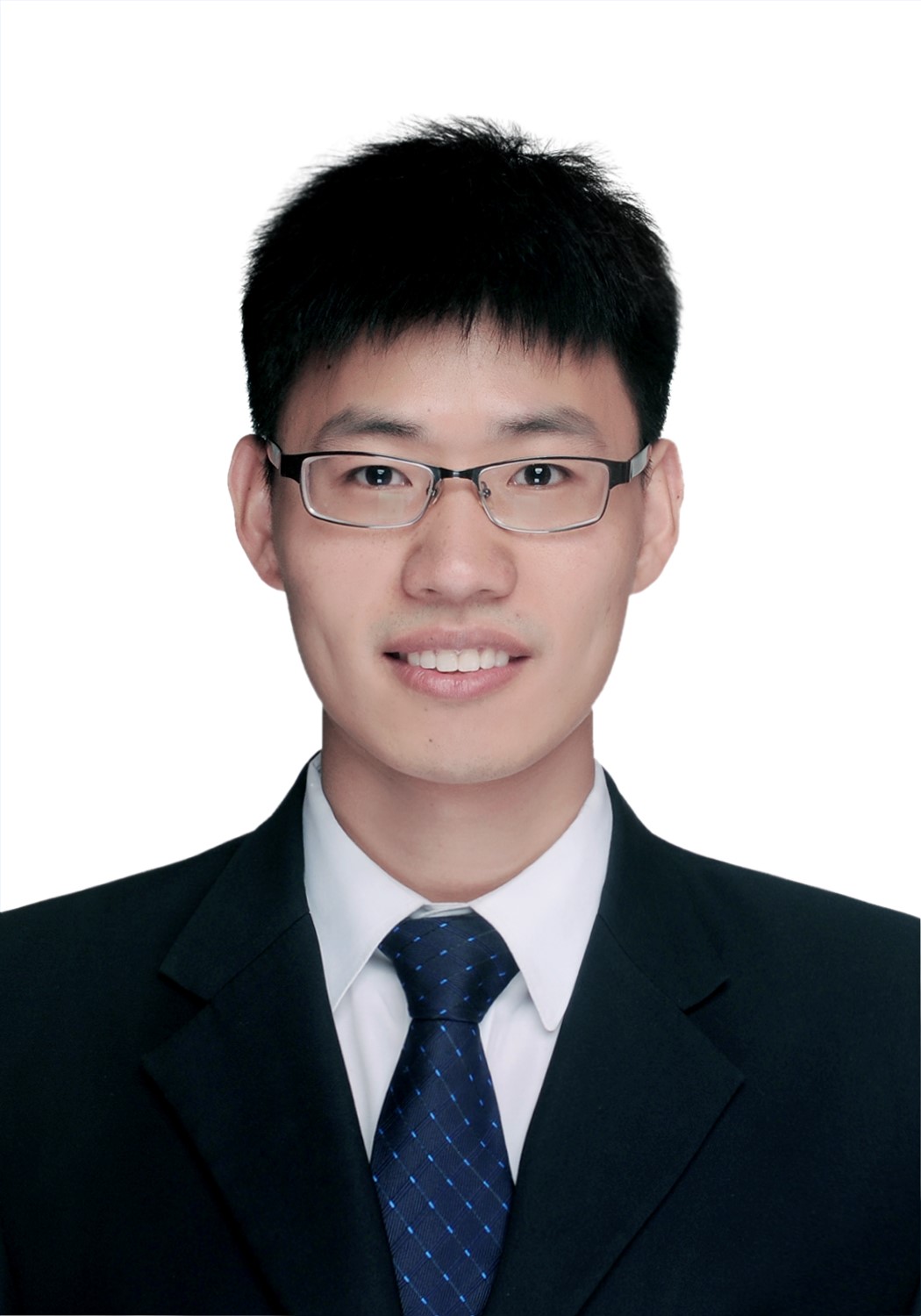
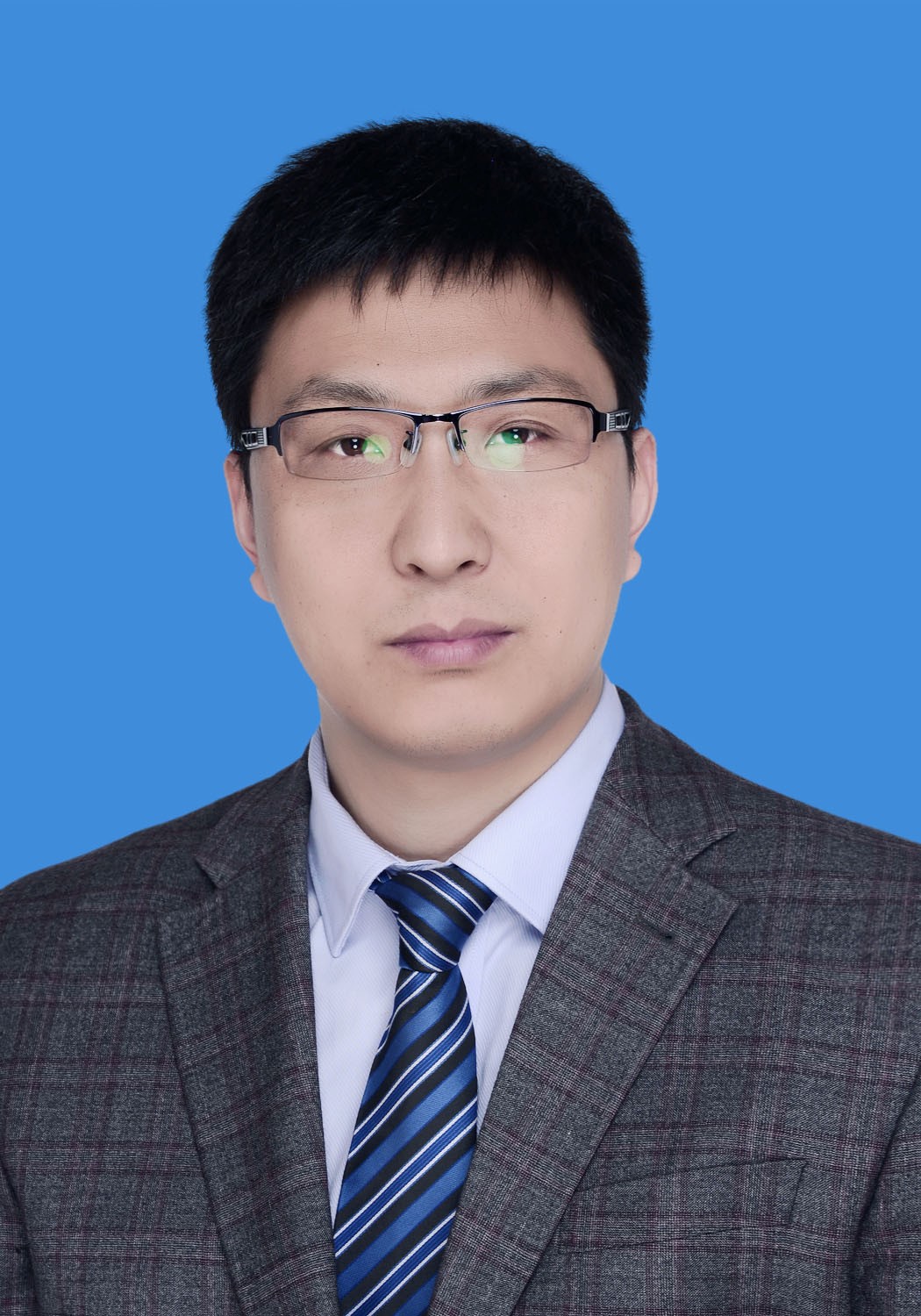
Comments
Comments must be written in English. Spam, offensive content, impersonation, and private information will not be permitted. If any comment is reported and identified as inappropriate content by OAE staff, the comment will be removed without notice. If you have any queries or need any help, please contact us at [email protected].