Crystalline phase-dependent cations migration in core-shell lanthanide-doped upconversion nanoparticles
Abstract
Core-shell structures are widely used to modulate upconversion emission or mitigate surface quenching and cross-relaxation in lanthanide-doped upconversion nanoparticles (UCNPs) to meet the requirements of various applications. Interfacial cation migration has been found recently to deteriorate the core-shell structures and thus affect their upconversion luminescence when they are subjected to annealing post-treatment. Herein, we demonstrate that the interfacial cation migration largely depends on the crystalline phase of the host lattice. Significant Er3+/Y3+(Yb3+) diffusion and migration occur across the core-shell interface when cubic NaREF4 (RE = Y, Yb, Er) core-shell or core-shell-shell UCNPs are annealed at 200 °C in the solid state, while the cation migration in hexagonal counterparts is negligible and the core-shell (core-shell-shell) structure can be well maintained in the same condition. The loosely packed atoms and large cubic void surrounded by eight F- ions in cubic NaREF4 lattice may facilitate cations diffusion and migration, enabling interfacial cations migration at relatively lower temperature. This finding may help us better understand temperature-dependent upconversion luminescent properties of core-shell UCNPs and better utilize various core-shell structured UCNPs according to different requirements of applications.
Keywords
INTRODUCTION
Different from downshifting emission often observed in conventional fluorophores and semiconductor quantum dots, lanthanide-doped upconversion nanoparticles (UCNPs) can emit high-energy photons via sequential absorption of two or multiple low-energy photons thanks to their abundant intermediate energy levels. In addition to their fascinating luminescent properties such as large anti-stoke shift, long lifetime, and sharp multiline emission ranging from UV to NIR, high chemical stability and low toxicity of lanthanide-doped UCNPs enable a broad range of applications, especially in background-free bio-sensing and biomedical domains[1-9]. Nevertheless, wider applications from bench to clinical practice are still severely hindered by their limited upconversion efficiencies and brightness.
Numerous strategies have been developed to enhance the upconversion luminescence (UCL) of lanthanide-doped UCNPs over the past two decades[10-16]. Among these, core-shell structures, represented by distinct distribution or separation of various lanthanide ions, have been widely adopted and demonstrated to be one of the most effective strategies for promoting energy transfer efficiency and modulating energy migration pathways[17-20]. Since the peculiar or highly efficient UCL of these core-shell UCNPs is largely dependent on the distribution of lanthanide ions within them, their structure integrality and invariability are significantly important for maintaining their UCL. Besides cation diffusion and intermixing demonstrated in single- and multi-shell UCNPs during epitaxial growth of the shell, leading to a solid-solution-like interface [21-23], cation migration and photoluminescent variation during the post-annealing process of core-shell UCNPs have recently attracted great attention. The diffusion and migration of Ce3+/Tb3+ across the core-shell interface were first validated after annealing of NaYF4: Ce@NaYF4: Tb core-shell nanoparticles in the solid state at temperatures higher than approximately 350 °C[24]. Subsequently, Zhang et al. found that the elemental migration occurred more easily during the post-annealing progress in an OA/ODE solution, and the migration efficiency was influenced by the relative lanthanide ion radius[25]. In our previous work, we demonstrated that the upconversion quantum yield of UCNPs could be significantly promoted in both cubic and hexagonal phase NaREF4 (RE = Y, Yb, Er) nanoparticles through core-shell structure engineering, where Er3+ activators and Yb3+ sensitizers are spatially separated[26]. However, the cubic and hexagonal core-shell-shell nanoparticles exhibit a discrepancy in temperature-dependent photoluminescence. Therefore, it is necessary to investigate the diffusion and migration of lanthanide ions across the neighboring layers in the two crystal phases for better utilization of these core-shell UCNPs.
In this work, we synthesize cubic and hexagonal phase single- and double-shell NaREF4 UCNPs via an epitaxial growth route and compare the cation migration across the interface of the neighboring layers in these two crystal phases. UCL performance and surface compositions of these UCNPs are collected before and after post-annealing to monitor the cation migration in the solid state. Our investigation reveals that the lanthanide cations are more prone to migrate across the interface in cubic nanoparticles compared to those in hexagonal counterparts.
EXPERIMENTAL
Materials
RE2O3 (RE=Y, Er, Yb; 99.99%), oleic acid (OA; 90%), 1-octadecene (ODE; 90%), yttrium chloride hexahydrate (YCl3·6H2O; 99.9%), erbium chloride hexahydrate (ErCl3·6H2O; 99.9%), ytterbium chloride hexahydrate (YbCl3·6H2O; 99.9%), sodium hydroxide (NaOH, 99.9%), and ammonium fluoride (NH4F, 98%) were purchased from Sigma-Aldrich. CF3COONa (NaTFA, 98%) was purchased from Alfa Aesar. Trifluoroacetic acid (99.5%) was purchased from Aladdin Inc. Ethanol, methanol, and cyclohexane were purchased from Sinopharm Chemical Reagent Co., China. All chemicals were used as received without any further purification.
Preparation of Lanthanide Trifluoroacetate (RETFA) Precursors
YTFA, ErTFA, and YbTFA (lanthanide trifluoroacetate (RETFA, RE=Y, Er, Yb)) were prepared by a literature method[27-28] with a slight modification. 50 mmol of RE2O3 was added in 50 mL trifluoroacetic acid aqueous solution. Then the mixture was heated to 95 °C under vigorous magnetic stirring until it became a transparent solution. The superfluous trifluoroacetic acid was evaporated, and the resultant solid RETFA was dissolved in 100 mL deionized water to prepare RETFA aqueous solution (1 M). 100 mmol of NaTFA was dissolved in 100 mL deionized water to acquire a stock solution (1 M).
Synthesis of α-NaYF4:10%Er and α-NaErF4 core nanoparticles
The cubic phase core nanoparticles were synthesized by the method we reported previously[26]. First, 1 mmol of NaTFA and RETFA solutions was mixed with 5 mL OA, 5 mL OM, and 10 mL ODE in a 50 mL three-necked flask. Next, the mixture was heated to 120 °C for 30 min with continuous magnetic stirring under an Ar atmosphere to remove water and oxygen. Then the transparent solution was quickly heated to 300 °C for 1 h before cooling to room temperature. After excessive ethanol was added to the solution, the resultant mixture was centrifuged at 10000 rpm for 10 min, collected and re-washed with a cyclohexane/ethanol mixed solution several times, and dispersed in 10 mL cyclohexane.
Synthesis of α-NaYF4:10%Er@NaYbF4 and α-NaErF4@NaYF4 nanoparticles
The α-core-shell nanoparticles were synthesized by an epitaxial growth protocol taking the core nanoparticles as seeds. In a typical procedure to synthesize NaErF4@NaYF4 nanoparticles, 3 mL of NaTFA solution, 3 mL of YTFA solution, and 3mL of core nanoparticle dispersion were mixed with 10 mL OA and 10 mL ODE in a 50 mL three-necked flask. The mixture was first heated to 90 °C for 30 min with continuous magnetic stirring under an Ar atmosphere to remove cyclohexane. Next, it was heated to 120 °C and kept for 30 min to evaporate water and oxygen. Subsequently, it was heated to 290 °C and kept for 30 min for epitaxial growth before cooling to room temperature. Finally, the resultant mixture was washed with cyclohexane/ethanol by a similar procedure to that utilized for washing the core nanoparticles.
Synthesis of α-NaYF4:10%Er@NaYbF4@NaYF4 nanoparticles
The procedure for synthesizing double-shell nanoparticles was identical to that for synthesizing single-shell ones, except for using single-shell UCNPs as the seeds and different RETFA precursors.
Synthesis of β-NaYF4:10%Er and β-NaErF4 core nanoparticles
The hexagonal phase core nanoparticles were synthesized via a protocol reported by Wang et al[29]. with a slight modification. In a typical procedure, 1 mmol of RECl3·6H2O was added into 6 mL OA and 15 mL ODE. Then the mixture was heated to 130 °C and kept for 30 min with continuous magnetic stirring under an Ar atmosphere to remove water until a clear lanthanide-oleate complexes solution formed. Subsequently, the solution was cooled to room temperature, and 6 mmol of NH4F (in 10 mL methanol) and 3 mmol of NaOH were added and stirred for 30 min. Afterward, the mixture was heated to 85 °C for 2 h to remove methanol, followed by being heated to 300 °C for an hour before cooling to room temperature. Finally, the resultant nanoparticles were washed with cyclohexane and ethanol via the same procedure as the cubic core nanoparticles.
Synthesis of β-core-shell nanoparticles
The synthesis procedure for β-singe/double-shell nanoparticles was identical to that utilized for synthesizing β-NaREF4 core nanoparticles, except for adding core or single-shell nanoparticles dispersed in cyclohexane along with methanol solution.
Annealing of UCNP films
The as-synthesized nanoparticles cyclohexane dispersions (0.02 mmol mL-1) were cast into homogeneous films on quartz plates [Supplementary Figure 1]. The UCNP films were quickly heated up to the setting temperature (heating rate: 30 °C min-1) for some time in a tubular furnace under a slow Ar flow (50 sccm) after degassing under a steady Ar flow (500 sccm) for half an hour (“sccm” is the abbreviation of “standard cubic centimeter per minute”). To avoid the combustion or carbonization of surface ligands, the films were firstly heated at 250 °C for 2h to remove surface ligands before they were heated to 400 °C, according to the TG curve of UCNPs [Supplementary Figure 2].
Nanoparticles characterization
The phase and crystal structure of the samples were characterized by using a powder X-ray diffractometer (XRD, PANalytical X'pert PRO-DY2198). The size and shape of the samples were observed via a transmission electron microscope (TEM, Jeol JEM 2100F) operated at an acceleration voltage of 200 kV. X-ray photoelectron spectroscopy (XPS) data were obtained using an ESCALab250 electron spectrometer from Thermo Fisher Scientific Corporation with monochromatic 150 W Al Kα radiation. The binding energies were referenced to the C1s line at 284.8 eV from adventitious carbon. The UCL properties were recorded on an Edinburgh FLS 980 spectrofluorometer in conjunction with a continuous-wave (CW) 980 nm diode laser. An Oxford Instrument Microstat HiRes2 was used to obtain the emission spectra within 298-498K. UCL lifetimes were measured with the same spectrofluorometer equipped with an optical parametric oscillator (OPO) as the excitation source (197-2750 nm, 20 Hz repetition rate, and about 3 ns pulse width).
RESULTS AND DISCUSSION
As a proof-of-concept experiment, we take the NaYF4:10%Er@NaYbF4@NaYF4 structured UCNPs as an example, which is demonstrated to render high upconversion efficiencies[26]. α/β-NaYF4:10%Er@NaYbF4@NaYF4 trilayered UCNPs were synthesized by using thermal decomposition and co-precipitation strategies, respectively. α/β-NaYF4 nanoparticles, single-doped with Er3+ (10%), were first synthesized and then served as cores for successive epitaxial growth of NaYbF4 shell (the first layer) and NaYF4 shell (the second layer) via the layer-by-layer growth process, which has been widely adopted by numerous groups[17-23]. As shown in Figure 1, the as-prepared core, single-shell, and double-shell nanoparticles exhibit highly uniform morphology and size for both the α and β phases. The average size of α-NaYF4 nanoparticles is determined to be about 11.1 ± 0.5 nm by analysis of over 100 particles from the TEM image [Figure 1A]. After epitaxial growth of approximately 8.5 nm NaYbF4 shell on the core nanoparticles, the as-synthesized α-NaYF4:10%Er@NaYbF4 core–shell nanoparticles are well dispersed with a sharp size distribution of 28.3 ± 1.0 nm [Figure 1B]. Subsequently, a final inert NaYF4 (about 2 nm thickness) is grown to suppress surface quenching, producing α-NaYF4:10%Er@NaYbF4@NaYF4 nanoparticles with 32.9 ± 1.0 nm [Figure 1C]. The average sizes of β-phase core (11.8 ± 0.5 nm), core-shell (28.1 ± 0.7 nm), and core-shell-shell UCNPs (32.5 ± 0.8 nm) are also precisely controlled to be nearly the same as those α-counterparts [Figure 1D-F]. The X-ray diffraction (XRD) patterns of these multilayered UCNPs can be well-indexed to cubic (JCPDS file number 77-2042) and hexagonal (JCPDS file number 16-0334) phases of NaYF4, respectively
Figure 1. TEM images of cubic (A-C, α-phase) and hexagonal (D-F, β-phase) NaYF4:10%Er core, NaYF4:10%Er @NaYbF4 core-shell; NaYF4:10%Er@NaYbF4@NaYF4 core-shell-shell nanoparticles. The size distribution is shown in the insets.
These nanoparticles were then cast into homogeneous films on a quartz plate, and temperature-dependent UCL spectra of α/β-NaYF4:10%Er@NaYbF4@NaYF4 UCNPs ranging from 298 to 498 K were collected, as shown in Figure 2. The upconversion emissions at 407, 540, and 651 nm of α/β-NaYF4:10%Er@NaYbF4@NaYF4 UCNPs correspond to 2H9/2→4I15/2, 4S3/2→4I15/2, and 4F9/2→4I15/2 transitions of Er3+, respectively. Different from the inert core-active shell nanoparticles[30], when the temperature increased, these upconversion emissions continuously decreased for either α or β-phase trilayered UCNPs as a result of the increasing multiphonon non-radiative relaxations[26,31-32]. Once the films were cooled to room temperature, β-NaYF4:10%Er@NaYbF4@NaYF4 UCNPs recovered their emissions intensity [Figure 2B], while the intensity of upconversion emission at 407, 540, and 651 nm of α-NaYF4:10%Er@NaYbF4@NaYF4 UCNPs dropped off about 45%, 39%, and 40%, respectively [Figure 2A]. This great discrepancy indicates structural integrity remains for β-phase UCNPs, and structural variation may have occurred for α-phase UCNPs during the heating process. To further amplify such discrepancy, we annealed α/β-NaYF4:10%Er@NaYbF4@ NaYF4 UCNP films at 200 °C for 4 h in a tubular furnace under a slow Ar flow.
Figure 2. In-situ temperature-dependent UCL spectrum of α- (A) and β- (B) NaYF4:10%Er@NaYbF4@NaYF4 UCNPs under 980-nm excitation.
XRD peaks of α/β-NaYF4:10%Er@NaYbF4@NaYF4 UCNPs remained unchanged [Supplementary Figure 3A and B] after annealing at 200 °C for 4 h, suggesting that the particle size and crystalline phase were well preserved. Thus recrystallization and growth of the crystalline grains can be excluded, consistent with the morphology and phase maintaining of NaYF4:Ce@NaYF4:Tb nanoparticles even after annealing at 350 °C[24]. As expected, the upconversion emissions of Er3+ at 407, 540, and 651 nm are significantly decreased by about 70%, 65%, and 63% for α-NaYF4:10%Er@NaYbF4@NaYF4 [Supplementary Figure 4], and the corresponding lifetimes changed from 0.42, 0.50, and 0.58 ms to 0.25, 0.28, and 0.36 ms, respectively [Figure 3]. Different from phase, the UCL and their corresponding decay times for β-NaYF4:10%Er@NaYbF4@NaYF4 UCNPs are well-maintained even after annealing for 4 h [Supplementary 5, Figure 4], suggesting the better structural integrality and invariability of β-core-shell-shell UCNPs compared with α-phase. Considering the size and crystalline phase of α-trilayerd UCNPs hardly changed after annealing, Yb3+/Er3+ migration across the core-shell and shell-shell interface may be responsible for the significant decrease of UC emission intensity and the corresponding lifetime since the surface quenching will occur in this case.
Figure 3. Decay curves at 407 (A); 540 (B); and 651 nm (C) for α-NaYF4:10%Er@NaYbF4@NaYF4 UCNPs before and after annealing at 200 °C for 4 h.
Figure 4. Decay curves at 408 (A); 541 (B); and 654 nm (C) for β-NaYF4:10%Er@NaYbF4@NaYF4 UCNPs before and after annealing at 200 °C for 4 h.
Because Ce3+/Tb3+ migration across the core-shell interface could be detected when β-NaYF4: Ce@NaYF4: Tb core-shell nanoparticles were annealed in the solid state at temperatures higher than about 350 °C[24], the
Figure 5. PL spectra (A) and decay curves of emissions at 407 (B); 540 (C); and 651 nm (D) for -NaYF4:10%Er@NaYbF4@NaYF4 UCNPs before and after annealing at 400 °C for 4 h (980-nm excitation).
Simplified core-shell nanoparticles were adopted to provide direct experimental evidence for such heating-induced ion migration for these trilayered nanoparticles. In this regard, we synthesized α/β-NaErF4@NaYF4 core-shell UCNPs and compared their structural variation, especially surface elemental compositions, after heating at 200 °C for 4 h. As shown in Figure 6, despite the elongated shape, the as-synthesized α-NaErF4@NaYF4 core-shell nanoparticles have similar core sizes (around 10.5 nm), shell thicknesses (about 6.5 nm, approximately 12.5 nm for the longer axis) to those of β-NaErF4@NaYF4 UCNPs. The high-resolution TEM (HRTEM) images in the insets [Figure 6B and D] demonstrate the high crystallinity of these core-shell nanoparticles, consistent with the XRD patterns without any significant impurity phases
Figure 6. TEM images of α- (A-B) and β- (C-D) NaErF4 core (A, C) and NaErF4@NaYF4 core-shell (B, D) nanoparticles. The size distribution histograms are shown in the insets. The size for elongated α-NaErF4@NaYF4 nanoparticles is represented by the shorter axes.
In addition to the UCL intensity decrease, the corresponding quantum yields for the above nanoparticles also degraded after annealing [Supplementary Table 1], further demonstrating the possible heating-induced ion migration.
EDS mapping was used for observation of such possible ion migration. Despite the relatively lower resolution, it seems that the element Er diffused into the shell after annealing the α-NaErF4@NaYF4 nanoparticles [Supplementary Figure 9]. In contrast, the distribution of Er for β-NaErF4@NaYF4 nanoparticles remained nearly unchanged after annealing. In order to acquire strong evidence, XPS techniques were also adopted for detecting interfacial ion migration. If Er3+/Y3+ migrated across the core-shell interface, in addition to Y3+ ions, Er3+ ions could also appear in the shell. Consequently, interfacial ion migration could be simply detected by surface composition analyses. As shown in the XPS spectra
Figure 7. XPS spectra of α- (A) and β- (C) NaErF4@NaYF4 UCNPs before and after annealing at 200 °C for 4 h, crystalline structure of α- (B) and β- (D) NaREF4.
These results verify that the cation ions (Er3+/Y3+) in the α-phase nanoparticles are much easier to diffuse and migrate than those in the β-ones. This discrepancy may be related to their crystalline structures. The -NaREF4 crystal has a high-symmetric fluorite structure similar to CaF2, with the Ca2+ sites randomly occupied by Na+ and RE3+ [Figure 7B]. By contrast, there are three types of inequivalent cation sites in the β-NaREF4
CONCLUSIONS
Cubic and hexagonal NaYF4:10%Er@NaYbF4@NaYF4 UCNPs were synthesized, and their temperature-dependent UCL was compared. In the temperature range of 298-498 K, an irreversible UCL variation was found in the former, while a reversible UCL change was observed in the latter. Unchanged crystalline phase and morphology after the two UCNP films were annealed at 200 °C, indicating their stable crystalline nature. At the same time, the change in surface compositions of cubic NaErF4@NaYF4 nanoparticles confirms that post-annealing resulted in Er3+/Y3+ migration across the core-shell interface. In contrast to cubic phase core-shell UCNPs, interfacial cation migration occurred at relatively higher temperatures for the hexagonal phase. We believe the loose packing atoms and the large cubic void consisting of eight F- ions in the cubic phase core-shell UCNPs benefit cations diffusion and migration, deteriorating their core-shell structural integrity and UCL properties. The densely packed hexagonal lattice may prohibit the interfacial cations diffusion, and the core-shell structure can be well maintained upon annealing at 200 °C. Our results suggest cation migration and structural invariability had better be considered if core-shell NaREF4 UCNPs are utilized in some practical applications.
DECLARATIONS
Acknowledgments
Here, the authors also want to thank the technical support from the Analytical and Testing Center at Huazhong University of Science and Technology.
Authors’ contributions
Planned the study, analyzed the data, and prepared the manuscript: Qin CY, Ma Y
Performed manuscript correcting: Gao JH, Xie XB, Zhai CP
Availability of data and materials
The TG curve, XRD patterns, PL spectra, and TEM images of some samples are published as Supplementary Materials in the journal.
Financial support and sponsorship
This work was supported by the National Natural Science Foundation of China (No. 21871099) and the Fundamental Research Funds for the Central Universities.
Conflicts of interest
All authors declared that there are no conflicts of interest.
Ethical approval and consent to participate
Not applicable.
Consent for publication
Not applicable.
Copyright
© The Author(s) 2023.
Supplementary Materials
REFERENCES
1. Liu Y, Lu Y, Yang X, et al. Amplified stimulated emission in upconversion nanoparticles for super-resolution nanoscopy. Nature 2017;543:229-33.
2. Tan M, Li F, Wang X, Fan R, Chen G. Temporal multilevel luminescence anticounterfeiting through scattering media. ACS Nano 2020;14:6532-8.
3. Nguyen NT, Huang K, Zeng H, et al. Nano-optogenetic engineering of CAR T cells for precision immunotherapy with enhanced safety. Nat Nanotechnol 2021;16:1424-34.
4. Fan Y, Wang P, Lu Y, et al. Lifetime-engineered NIR-II nanoparticles unlock multiplexed in vivo imaging. Nat Nanotechnol 2018;13:941-6.
5. Rabie H, Zhang Y, Pasquale N, Lagos MJ, Batson PE, Lee KB. NIR biosensing of neurotransmitters in stem cell-derived neural interface using advanced core-shell upconversion nanoparticles. Adv Mater 2019;31:e1806991.
6. Zuo J, Tu L, Li Q, et al. Near infrared light sensitive ultraviolet-blue nanophotoswitch for imaging-guided "off-on" therapy. ACS Nano 2018;12:3217-25.
7. Ren H, Huang F, Jiang J, Wang L, Zhang J. Development of photocatalyst based on NaYF4: Yb, Tm@NaYF4: Yb, Ce/NH2-MIL-101 (Cr): Doping Ce3+ ions to promote the efficient energy transfer between core and shell. Chem Eng J 2022;427:132023.
8. Huang F, Labrador-páez L, Ågren H, et al. Transient energy trapping as a size-conserving surface passivation strategy for producing bright ultrasmall upconversion nanoprobes. Nano Energy 2023;105:108015.
9. Pan Y, Liu X, Zhang W, et al. Bifunctional template-mediated synthesis of porous ordered g-C3N4 decorated with potassium and cyano groups for effective photocatalytic H2O2 evolution from dual-electron O2 reduction. Chem Eng J 2022;427:132032.
10. Hao J, Zhang Y, Wei X. Electric-induced enhancement and modulation of upconversion photoluminescence in epitaxial BaTiO3:Yb/Er thin films. Angew Chem Int Ed Engl 2011;50:6876-80.
11. Yin W, Zhao L, Zhou L, et al. Enhanced red emission from GdF3:Yb3+,Er3+ upconversion nanocrystals by Li+ doping and their application for bioimaging. Chemistry 2012;18:9239-45.
12. Zhao J, Jin D, Schartner EP, et al. Single-nanocrystal sensitivity achieved by enhanced upconversion luminescence. Nat Nanotechnol 2013;8:729-34.
13. Wang J, Deng R, MacDonald MA, et al. Enhancing multiphoton upconversion through energy clustering at sublattice level. Nat Mater 2014;13:157-62.
14. Zhou B, Yan L, Huang J, Liu X, Tao L, Zhang Q. NIR II-responsive photon upconversion through energy migration in an ytterbium sublattice. Nat Photonics 2020;14:760-6.
15. Hofmann CLM, Fischer S, Eriksen EH, et al. Experimental validation of a modeling framework for upconversion enhancement in 1D-photonic crystals. Nat Commun 2021;12:104.
16. Huang F, Bagheri N, Wang L, et al. Low-lying excited state energy trap induced by cross-relaxation-The main origin of concentration quenching in lanthanide upconversion nanoparticles. J Alloys Compd 2023;936:168149.
17. Zhong Y, Tian G, Gu Z, et al. Elimination of photon quenching by a transition layer to fabricate a quenching-shield sandwich structure for 800 nm excited upconversion luminescence of Nd3+-sensitized nanoparticles. Adv Mater 2014;26:2831-7.
18. Chen X, Jin L, Kong W, et al. Confining energy migration in upconversion nanoparticles towards deep ultraviolet lasing. Nat Commun 2016;7:10304.
19. Liu Q, Zhang Y, Peng CS, Yang T, Joubert LM, Chu S. Single upconversion nanoparticle imaging at sub-10 W cm-2 irradiance. Nat Photonics 2018;12:548-53.
20. Shi R, Brites CDS, Carlos LD. Understanding the shell passivation in Ln3+-doped luminescent nanocrystals. Small Structures 2022;3:2100194.
21. Dühnen S, Haase M. Study on the intermixing of core and shell in NaEuF4 /NaGdF4 core/shell nanocrystals. Chem Mater 2015;27:8375- 86.
22. Hudry D, Popescu R, Busko D, et al. Interface disorder in large single-and multi-shell upconverting nanocrystals. J Mater Chem C 2019;7:1164-72.
23. Bastian PU, Nacak S, Roddatis V, Kumke MU. Tracking the motion of lanthanide ions within core-shell-shell NaYF4 nanocrystals via resonance energy transfer. J Phys Chem C 2020;124:11229-38.
24. Chen B, Peng D, Chen X, Qiao X, Fan X, Wang F. Establishing the structural integrity of core-shell nanoparticles against elemental migration using luminescent lanthanide probes. Angew Chem Int Ed Engl 2015;54:12788-90.
25. Liu L, Li X, Fan Y, et al. Elemental migration in core/shell structured lanthanide doped nanoparticles. Chem Mater 2019;31:5608-15.
26. Zhou B, Tang B, Zhang C, et al. Enhancing multiphoton upconversion through interfacial energy transfer in multilayered nanoparticles. Nat Commun 2020;11:1174.
27. Roberts JE. Lanthanum and neodymium salts of trifluoroacetic acid. J Am Chem Soc 1961;83:1087-8.
28. Zhou B, Xu B, He H, et al. Enhanced green upconversion luminescence in tetrahedral LiYF4:Yb/Er nanoparticles by manganese(ii)-doping: the key role of the host lattice. Nanoscale 2018;10:2834-40.
29. Wang F, Deng R, Liu X. Preparation of core-shell NaGdF4 nanoparticles doped with luminescent lanthanide ions to be used as upconversion-based probes. Nat Protoc 2014;9:1634-44.
30. Zhou Y, Cheng Y, Xu J, Lin H, Wang Y. Thermo-enhanced upconversion luminescence in inert-core/active-shell UCNPs: the inert core matters. Nanoscale 2021;13:6569-76.
31. Wu X, Zhan S, Han J, Liu Y. Nanoscale ultrasensitive temperature sensing based on upconversion nanoparticles with lattice self-adaptation. Nano Lett 2021;21:272-8.
32. Wang Y, Zhou S, Hu P, Zhong W, Fu J. Temperature-sensitive lanthanide-doped core-multishell nanocrystals with excitation-wavelength-dependent bimodal luminescence thermo-behaviors and their application in dynamic anticounterfeiting. J Alloys Compd 2023;938:168442.
33. Uwamino Y, Tsuge A, Ishizuka T, Yamatera H. X-Ray photoelectron spectroscopy of rare earth halides. BCSJ 1986;59:2263-7.
34. Powell C. Elemental binding energies for X-ray photoelectron spectroscopy. Appl Surf Sci 1995;89:141-9.
35. Huang J, Li J, Zhang X, et al. Artificial Atomic vacancies tailor near-infrared II excited multiplexing upconversion in core-shell lanthanide nanoparticles. Nano Lett 2020;20:5236-42.
36. Johnson NJ, He S, Diao S, Chan EM, Dai H, Almutairi A. Direct evidence for coupled surface and concentration quenching dynamics in lanthanide-doped nanocrystals. J Am Chem Soc 2017;139:3275-82.
37. Chen Q, Xie X, Huang B, et al. Confining excitation energy in Er3+-sensitized upconversion nanocrystals through Tm3+-mediated transient energy trapping. Angew Chem Int Ed Engl 2017;56:7605-9.
38. Wang Y, Zhou S, Sun F, Hu P, Zhong W, Fu J. In-depth insight into the Yb3+ effect in NaErF4-based host sensitization upconversion: a double-edged sword. Nanoscale 2022;14:16156-69.
39. Zhou S, Wang Y, Hu P, et al. Cascaded photon confinement-mediated orthogonal RGB-switchable naerf4-cored upconversion nanoarchitectures for logicalized information encryption and multimodal luminescent anti-counterfeiting. Laser Photonics Rev 2023;17:2200531.
Cite This Article

How to Cite
Download Citation
Export Citation File:
Type of Import
Tips on Downloading Citation
Citation Manager File Format
Type of Import
Direct Import: When the Direct Import option is selected (the default state), a dialogue box will give you the option to Save or Open the downloaded citation data. Choosing Open will either launch your citation manager or give you a choice of applications with which to use the metadata. The Save option saves the file locally for later use.
Indirect Import: When the Indirect Import option is selected, the metadata is displayed and may be copied and pasted as needed.
About This Article
Copyright
Author Biographies
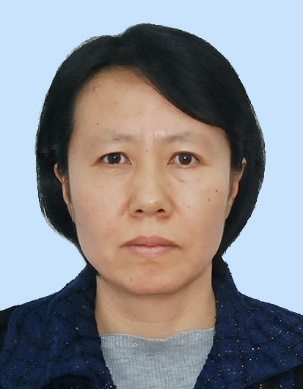
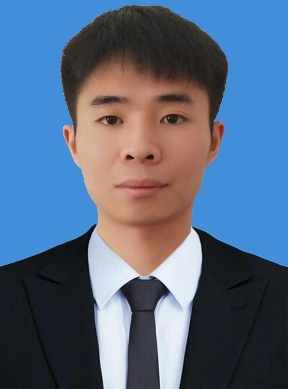
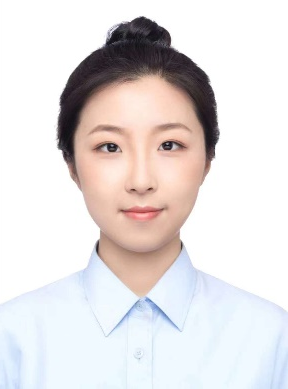
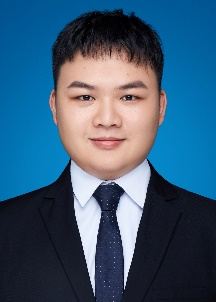
Comments
Comments must be written in English. Spam, offensive content, impersonation, and private information will not be permitted. If any comment is reported and identified as inappropriate content by OAE staff, the comment will be removed without notice. If you have any queries or need any help, please contact us at [email protected].