Biotransformation studies on bioactive compounds: 25 years of interesting research at the ICCBS
Abstract
Biotransformation of natural, synthetic, and semi-synthetic compounds has emerged as a frontier branch of chemical sciences that is progressively being applied in numerous fields. In the present review, we have summarized our biotransformation studies on bioactive compounds from 1997 to 2022. Various microbial and plant cell cultures were used for biocatalytic structural transformations. We present here an overview of biotransformation of 53 compounds belonging to various classes of natural, synthetic, and semi-synthetic compounds, published in several leading journals. The structures of the resulting metabolites have been elucidated by detailed spectroscopic studies. Oxidation, reduction, dehydrogenation, chlorination, aromatization, methylation, demethylation, rearrangements, etc. were the main reactions that occurred during the biotransformation processes. Many of the biotransformed products exhibited interesting biological activities. Structural transformations in some cases have also led to improved pharmacokinetic profiles. This review is aimed to provide a focused account of extensive work carried out in our laboratories in this field, as well as the immense potential of biocatalytic transformations in organic chemistry.
Keywords
BIOTRANSFORMATION- AN EFFICIENT GREEN CHEMISTRY APPROACH
Biotransformation techniques are among the most efficient approaches for structural transformation of various classes of organic compounds. These techniques involve the use of low-cost, eco-friendly, and non-toxic biocatalysts. They are normally conducted under ambient reaction conditions and involve oxidation, dehydrogenation, chlorination, reduction, aromatization, methylation, demethylation, rearrangements, etc. Biotransformation procedures can also reduce the total number of reaction steps towards the desired products. They do not require the use of toxic chemicals and harsh conditions that may otherwise be necessary for the functional group activation, protection, and deprotection steps, resulting in less production of wastes as compared to the desired products. These techniques have effectively been employed in drug discovery and development, affording libraries of compounds around core structures. These libraries are then evaluated for various biological activities in the drug discovery phase. The most significant aspect of biocatalytic transformations is the conservation of the original carbon skeleton of the starting material after transformation[1-10].
Biotransformation techniques are catalyzed by various biological systems, such as actinomycetes, algae, fungi, bacteria, yeasts, and plants and animals cell cultures, as well as by pure enzymes, affording compounds with high stereo-, regio-, and chemo-selectivity. They may be applied on potentially important scaffolds for the design, discovery, and development of new bioactive multi-functional compounds, including pharmaceuticals. Fungi have been widely used in whole-cell biocatalysis of organic compounds due to the presence of cytochrome P450 systems. In addition, fungi have higher metabolic and multiplication rates, thus serving as an excellent source for whole-cell biocatalysis. Pure enzyme-catalyzed biotransformation reactions often produce single and specific metabolites. Biotransformation reactions with whole colonies of microorganisms or plant/animal cell cultures may, however, produce more than one metabolite due to the involvement of a range of enzymes. Whole-cell biocatalysts are cost-effective, easy to handle, and usually stable in the long term. Moreover, biotransformation reactions by whole-cell colonies do not require co-factors[11-20].
BIOTRANSFORMATION STUDIES ON BIOACTIVE COMPOUNDS
Bioactive compounds are extra-nutritional constituents that usually occur in small quantities in plants/foods. They have been extensively evaluated for their effects on human health. These bioactive compounds have diverse structures and distributions in nature. At present, several bioactive compounds have been derivatized through bio-catalysis with applications in the field of medicine. Bio-catalytic transformation of bioactive compounds has emerged as a frontier field of chemical sciences that is being extensively employed in numerous other fields. Several natural products, e.g., monoterpenes, sesquiterpenes, diterpenes, sesterterpenes, triterpenes, anabolic, contraceptive, and anti-cancer steroids, steroidal alkaloids, and flavonoids, as well as other bioactive compounds of synthetic origin, have been structurally transformed using biocatalytic approaches in our laboratories since 1997. In the present review, we have compiled the results of these biotransformation studies.
For the biotransformation studies, media was prepared by mixing specific media ingredients, transferred into flasks, cotton plugged, autoclaved, inoculated with microbial/plant cell cultures under sterilized conditions, and placed on a rotary shaker (2-4 days). After the maximum growth of microbial/plant cell cultures, substrates/drugs were dissolved in water-miscible solvents, such as methanol, DMSO, and acetone, and fed in flasks containing microbial/plant cell cultures. The material was placed again on a rotary shaker for 2 to 15 days. Oxidation, reduction, dehydrogenation, chlorination, aromatization, methylation, demethylation, and rearrangements were the main reactions observed during the biotransformation studies. The reaction was terminated by adding water-immiscible solvents, such as dichloromethane (DCM) or ethyl acetate (EtOAc), filtered to separate biomass, extracted thrice with DCM/EtOAc, and evaporated using a rotary evaporator.
The crude materials were initially fractionated by column chromatography by using hexanes-ethyl acetate or hexanes-acetone solvent systems. The fractions were finally purified by reverse phase or/and normal phase HPLC, followed by thin layer chromatography (TLC). The structures of purified derivatives were established by using 1D-, and 2D-NMR (Nuclear Magnetic Resonance), HREI-MS (High-Resolution Electron Ionization Mass Spectrometry), HRESI-MS (High-Resolution Electrospray Ionization Mass Spectrometry), HRFAB-MS (High-Resolution Fast Atom Bombardment Mass Spectrometry), IR (Infrared), and UV (Ultraviolet–visible) spectral data, and single-crystal X-ray diffraction analyses. Fully purified compounds were evaluated for different biological activities.
In certain cases, solid phase fermentation was also employed to increase the yields and diversity of metabolites.
Spectroscopy is the investigation and measurement of spectral data produced by the interaction of samples with electromagnetic radiation. NMR spectroscopy plays a major role in the structure determination of organic molecules, and other biological macromolecules. Chemical shifts are accurately measured by NMR parameters as sensitive probes of molecular structures. HSQC spectroscopy determines the correlations between two different types of nuclei (1H with 13C or 1H with 15N), which are separated by one bond. HMBC spectroscopy correlates 1H and 13C nuclei through two, three, or sometimes four bonds. COSY (¹H-¹H Correlation) Spectroscopy shows the correlation between hydrogens that are coupled to each other in the ¹H-NMR spectrum. NOESY is frequently used to determine the spatial structure of organic molecules. The CD (Circular dichroism) is the difference in absorption of left and right circularly polarized light. Only chiral molecules display CD, and enantiomers have CD of equal magnitude but opposite sign.
KEY OBJECTIVES
The main objectives of our work on biotransformation studies were as follows: 1: To synthesize libraries of new and novel analogues of natural, synthetic, and semisynthetic compounds through eco-friendly and cost-effective biotransformation techniques with the aim of improving their pharmacodynamic profiles; 2: To produce potentially interesting regio-, stereo-, enantio-selective compounds without the use of toxic chemicals and harsh conditions; 3: To evaluate the resultant transformed products for different biological activities, e.g., enzyme inhibition, anti-inflammatory, anti-cancer, anti-bacterial, etc.
BIOTRANSFORMATION OF MONOTERPENES
Biotransformation of (-)-α-(1), and β-pinene (6)
α-(1), and β-pinene (6) are well-known monoterpenes having a range of pharmacological activities, such as anti-microbial, anti-inflammatory, anti-oxidant, anti-malarial, and anti-leishmanial. They are the major constituents of many aromatic plants. Biotransformation of (-)-α-pinene (1) with the fungal culture of Botrytis cinerea, afforded three new hydroxylated metabolites, 3-hydroxy-(-)-β-pinene (2) (16.5%), 9-hydroxy-(-)-α-pinene (3) (13.5%), and 4-hydroxy-(-)-α-pinene-6-one (4) (20%), along with a known metabolite verbenone (5) (28%)[21] [Figure 1].
Similarly, biotransformation of (-)-β-pinene (6), a structural isomer of (-)-α-pinene, with the fungal culture of Botrytis cinerea yielded four new hydroxylated metabolites, (-)-6α-hydroxy-β-pinene (7) (22%), (-)-4β,5β-dihydroxy-β-pinene (8) (10%), (-)-2β,3β-dihydroxypinane (9) (12%), and (-)-4β-hydroxy-β-pinene-6-one (10) (9%)[22] [Figure 2].
Biotransformation of terpinolene (11)
Two new metabolites, 2,3-dihydro-3β,6β-dihydroxy-terpinolene (12) (39%), and 2,3-dihydro-1α,3α-dihydroxy-terpinolene (13) (20%) of the anti-bacterial and anti-fungal monoterpene terpinolene (11) were synthesized through biotransformation of compound 11 with the fungal culture of Botrytis cinerea. These derivatives 12 and 13 showed no activity against the plant pathogenic fungus Cladosporium herbarum, as compared to the substrate 11[23] [Figure 3].
Biotransformation of (-)-menthol (14), and (+)-menthol (21)
Menthol, a commercially used constituent of the mint plant, is used to treat minor pains in the muscles and joints. Biotransformation of (-)-menthol (14) by the fungal culture of Cephalosporium aphidicola yielded four new metabolites, 10-acetoxymenthol (15) (3%), 4α-hydroxymenthol (16) (7.5%), 3α-hydroxymenthol (17) (2.3%), and 10-hydroxymenthol (18) (21.5%), along with the two known metabolites, 7-hydroxymenthol (19) (4.8%), and 9-hydroxymenthol (20) (14%)[24] [Figure 4].
Similarly, biotransformation of (+)-menthol (21), with the fungal culture of Macrophomina phaseolina afforded five new hydroxylated metabolites, 2β, 8-dihydroxymenthol (22) (1%), 8, 9-dihydroxymenthol (23) (0.8%), 6β, 8-dihydroxymenthol (24) (1.3%), 1β, 8-dihydroxymenthol (25) (1.1%), and 7, 8-dihydroxymenthol (26) (1.5%), along with four known metabolites, 8-hydroxymenthol (27) (7.4%), 6β-hydroxymenthol (28) (2.5%), 1β-hydroxymenthol (29) (5.5%), and 9-hydroxymenthol (30) (2.2%)[25] [Figure 5].
Biotransformation of thymoquinone (31)
Biotransformation of a major constituent of black seeds, Nigella sativa, thymoquinone (31) with the plant pathogenic fungus Aspergillus niter afforded a new seven-membered lactone derivative, 5-isopropyl-2-methyloxepin-1-one (32) (2%) [Proposed Supplementary Figure 1], along with two known metabolites, 3-hydroxy-5-isopropyl-2-methylcyclohexa-2,5-diene-1,4-dione (33) (8%), and 5-isopropyl-2-methylbenzene-1,4-diol (34) (28%)[26] [Figure 6] [Proposed Supplementary Figure 2].
BIOTRANSFORMATION OF SESQUITERPENES
Biotransformation of (+)-isolongifolen-4-one (35)
Five new oxidative derivatives, 12-hydroxyisolongifolen-4-one (36), 13-hydroxyisolongi-folen-4-one (37), 11-hydroxyisolongifolen-4-one (38), 10-hydroxyisolongifolen-4-one (39), and 9-hydroxyisolongifolen-4-one (40) were synthesized through the microbial transformation of (+)-isolongifolen-4-one (35)[27] [Figure 7]. Compound 35 is used in the perfumery industry. Derivatives 37 (IC50 = 9.64 ± 0.0008 μM) and 40 (IC50 = 6.68 ± 0.0096 μM) were identified as potent inhibitors of tyrosinase, an important enzyme for melanin biosynthesis, as compared to substrate 35 (IC50 = 51.91 ± 0.0245 μM). Metabolite 36 showed moderate inhibitory activity (IC50 = 101.01 ± 0.1978 μM).
Biotransformation of (-)-isolongifolol (41)
Biotransformation of (-)-isolongifolol (41) with Fusarium lini resulted in three new polar oxygenated derivatives, 10-oxoisolongifolol (42) (1.2%), 10α-hydroxyisolongifolol (43) (16%), and 9α-hydroxyisolongifolol (44) (22%). The presence of an α-OH at C-10 in the metabolite 42 increased its inhibitory potential against butyrylcholinesterase, an enzyme whose inhibition reduces memory deficiency in Alzheimer’s patients, with the IC50 value of 13.6 μM, while the presence of an α-OH at C-11 in the metabolite 43 decreased its activity (IC50 = 299.5 μM)[28] [Figure 8].
Biotransformation of 5α-hydroxycaryophylla-4(12), 8(13)-diene (45)
Macrophomina phaseolina-mediated structural transformation of the naturally occurring sesquiterpene, 5α-hydroxycaryophylla-4(12), 8(13)-diene (45) resulted in three new metabolites, 4β-methoxycaryophyllene-5α, 14-diol (46) (3.5%), 4β-methoxycaryophyllene-5α, 15-diol (47) (7.6%), and caryophyllene-5α, 15-diol (48) (2.7%) [Figure 9]. The transformed products 46 (IC50 = 3.09 ± 2.61 μg/mL), 47 (IC50 = 0.72 ± 0.17 μg/mL), and 48 (IC50 = 1.35 ± 0.43 μg/mL) showed significant anti-malarial activity, in comparison to the standard, chloroquine diphosphate (IC50 = 0.025 ± 0.01 μg/mL) by using parasite lactate dehydrogenase assay in vitro[29].
Biotransformation of (-)-caryophyllene oxide (49)
Biotransformation of another sesquiterpene (-)-caryophyllene oxide (49), a common constituent of essential oils, with the cell suspension culture of medicinal plant Catharanthus roseus afforded two new derivatives, 2β-hydroxycaryophyllene oxide (50) (7.5%), and 2-hydroxy-4, 5- epoxycaryophyllan-13-ol (51) (4.6%), along with two known derivatives, 15-hydroxycaryophyllene oxide (52) (2%), and 4β, 5α-dihydroxycaryophyll-8 (13) -ene (53) (6%)[30] [Figure 10].
In addition, biotransformation of (-)-caryophyllene oxide (49) with the fungal cell culture Cephalosporium aphidicola yielded two known metabolites, 4β, 5α-dihydroxycaryophyll-8 (13) -ene (53) (2.7%) and clovane-5,9-diol (54) (1.3%) [Figure 11] [Proposed Supplementary Figure 3]. Similarly, fungal transformation of 49 with Macrophomina phaseolina also yielded two known transformed products, 15-hydroxycaryophyllene oxide (52) (3.2%), and 4, 5-epoxycaryophyllan-8 (13) -en-14-ol (55) (4.6%)[31] [Figure 11]. Moreover, four new derivatives, 4, 5-epoxy-13-norcaryophyllan-8-one (56) (2.5%), caryolane-5, 8, 13-triol (57) (2.3%), clovane-5, 9, 12-triol (58) (2.1%), and 4, 5-epoxycaryophyllan-3, 13-diol (59) (2.1%), were also synthesized by our research group through the biotransformation of (-)-caryophyllene oxide (49) with Rhizopus stolonifer, Aspergillus niger, Gibberella fujikuroi, and Fusarium lini, respectively[31] [Figure 12].
Figure 11. Microbial transformation of (-)-caryophyllene oxide (49) with Cephalosporium aphidicola, and Macrophomina phaseolina.
Figure 12. Microbial transformation of (-)-caryophyllene oxide (49) with Rhizopus stolonifer,Aspergillus niger, Gibberella fujikuroi, and Fusarium lini.
Derivatives 52 (IC50 = 44.0 ± 0.2 μM), 53 (IC50 = 455.8 ± 0.1 μM), 54 (IC50 = 189.5 ± 0.2 μM), 55 (IC50 = 10.9 ± 0.2 μM), 56 (IC50 = 458.7 ± 0.5 μM), 57 (IC50 = 23.6 ± 0.1 μM), 58 (IC50 = 43.6 ± 0.3 μM), and 59 (IC50 = 154.6 ± 0.3 μM) showed a moderate to significant inhibitory potential against butyrylcholinesterase, as compared to the substrate 49 (IC50 = 208.4 ± 0.8 μM).
Biotransformation of (+)-cycloisolongifol-5β-ol (60)
Cunninghamella elegans-mediated transformation of a cyclic sesquiterpene (+)-cycloisolongifol-5β-ol ((60) afforded three new metabolites, cycloisolongifol-3β,5β-diol (61) (3.7%), cycloisolongifol-5β-ol-11-one (62) (4%), and cycloisolongifol-3β, 5β, 11α-triol (63) (3%)[32] [Figure 13].
Biotransformation of (-)-ambrox (64)
Fungal transformation of another perfumery sesquiterpene, (-)-ambrox (64) with Fusarium lini yielded four compounds, ambrox-1α-ol (65) (2.7%), ambrox-1α,11α-diol (66) (1.3%), ambrox-1α,6α-diol (67) (3.2%), and ambrox-1α,6α,11α-triol (68) (4.6%) [Figure 14]. Similarly, four more derivatives, ambrox-3-one (69) (1.3%), ambrox-3β-ol (70) (1%), ambrox-3β,6β-diol (71) (1.9%), and tetranorlabdane-3, 8, 12-triol (72) (4.7%) of substrate 64 were also synthesized through its biotransformation with Rhizopus stolonifer[33] [Figure 15]. Derivatives 65, 67-69, ambrox-1α, 3β-diol (73) (10.1%), and ambrox-1α, 6β-diol (74) (15.7%) were also obtained via incubation of (-)-ambrox (64) with Actinidia deliciosa (Kiwi fruit)[34] [Figure 16].
Figure 16. Microbial transformation of (-)-ambrox (64) with Actinidia deliciosa, and Macrophomina phaseolina.
Moreover, biotransformation of (-)-ambrox (64) with Macrophomina phaseolina afforded a new compound, 1α-hydroxy-3-oxoambrox (75) (3.5%), along with four known compounds 70, and 72-74[35] [Figure 16]. Three more derivatives, 69, ambrox-2α-ol (76) (2.5%), and ambrox-2α,3β-diol (77) (3%), were also synthesized through biotransformation of (-)-ambrox (64) by using cell suspension culture of plant Peganum harmala[35] [Figure 17]. Some of the resulting metabolites exhibited exotic aroma, different from the substrate 64.
Biotransformation of artemether (78)
Biotransformation of an anti-malarial sesquiterpenoid drug artemether (78) with plant cell suspension culture of Azadirachta indica afforded two derivatives, 9α-acetoxy, 10β-methoxyartemethin (79) (1.2%), and 3α-hydroxy, 12β-methoxyartemethin (80) (2.5%) [Figure 18]. Three derivatives 79, 80, and 3α-12β-dihydroxyartemethin (81) (3.5%) were obtained from Macrophomina-phaseolina-mediated transformation of 78 [Figure 18] [Supplementary Figure 4]. In addition, two new derivatives, peroxy-linkage, 9α-hydroxyartemethin (82) (2.8%), and 10β-hydroxyartemethin (83) (4.6%), were obtained via the biotransformation of artemether (78) with Fusarium lini[36] [Figure 18] [Supplementary Figure 4]. Compounds 79-83 showed no anti-malarial activity (Plasmodium falciparum, 3D7 strain) in vitro.
Biotransformation of sclareolide (84)
Curvularia lunata-catalyzed transformation of a sesquiterpene lactone sclareolide (84) afforded a new derivative, 1α, 3β-dihydroxysclareolide (85) (16%), along with four derivatives, 3-ketosclareolide (86) (8.7%), 1β-hydroxysclareolide (87) (9.3%), 3β-hydroxysclareolide (88) (12.2%), and 1β,3β-dihydroxysclareolide (89) (7%). Biotransformation of 84 with Aspergillus niger also yielded metabolites 85-89. Transformed products 85-88 were also obtained by the transformation of 84 with Gibberella fujikuroii, while fermentation of 84 with Fusarium lini produced metabolites 87 and 88[37] [Figure 19]. Similarly, biotransformation of sclareolide (84) with Cunninghamella elegans afforded six derivatives 86, 88, 89, 2α-hydroxysclareolide (90) (5.3%), 2α, 3β-dihydroxysclareolide (91) (23.6%), 1α, 3β-dihydroxysclareolide (92) (2.7%), and 3β-hydroxy-8-episclareolide (93) (1.3%)[33] [Figure 20]. Structural transformations in derivatives 86 (100%), 88 (87.5%), 89 (72.3%), 90 (82.7%), and 92 (75%) have increased their phytotoxicity against Lemna minor L., in comparison to the substrate 84 (62.5%), while derivative 91 (50%) showed weak phytotoxicity at 100 μg/mL.
Biotransformation of (-)-guaiol (94)
Biotransformation of a sesquiterpene (-)-guaiol (94) with Rhizopus stolonifer afforded a new compound, 1-guaiene-9α,11-diol (95) (1.6%) [Figure 21]. Similarly, Cunninghamella elegans-assisted transformation of 94 yielded compounds, 1-guaiene-3α,11-diol (96) (1.8%), and 1(5)-guaiene-3α,9,11-triol (97) (1.8%) [Figure 21], while Macrophomina phaseolina-catalyzed biotransformation of 94 resulted in two derivatives, 1(5)-guaien-11-ol-6-one (98) (2.1%), and 1-guaien-11-ol-3-one (99) (2%)[38] [Figure 21].
BIOTRANSFORMATION OF DITERPENES
Biotransformation of sclareol (100)
Two new derivatives, 1β-hydroxysclareol (101) (1.3%) and 12-hydroxysclareol (102) (1%), were synthesized through the biotransformation of the diterpene, sclareol (100) with Fusarium lini [Figure 22]. Similarly, two new derivatives, 3β-hydroxysclareol (103) (2.7%) and 18-hydroxysclareol (104) (1.3%), and the two known metabolites, 6α,18-dihydroxysclareol (105) (3.2%) and 11,18-dihydroxysclareol (106) (4.6%), were also synthesized through the biotransformation of sclareol (100) with Rhizopus stolonifer[39] [Figure 23].
Biotransformation of andrographolide (107)
Biotransformation of andrographolide (107) with Cephalosporium aphidicola and Cunninghamella elegans yielded two derivatives, andropanolide (108) (1.7%) and 14-deoxy-11, 12-didehydroandrographolide (109) (1.6%) [Figure 24], respectively[40].
Biotransformation of dehydroabietic acid (110)
Three new derivatives, 1β-hydroxydehydroabietic acid (111) (0.8%), 15-hydroxy dehydroabietic acid (112) (1.1%), and 16-hydroxy dehydroabietic acid (113) (1.4%), were obtained via microbial transformation of the diterpene dehydroabietic acid (110)[41] [Figure 25]. Compounds 110 (IC50 = 11 ± 01 μM), 111 (IC50 = 130 ± 15 μM), 112 (IC50 = 99 ± 43 μM), and 113 (IC50 = 81 ± 90 μM) showed a potent α-glucosidase inhibitory activity, as compared to the standard acarbose (IC50 = 780 ± 20 μM). α-Glucosidase inhibitors delay the digestion of carbohydrates, resulting in a reduction of post-prandial sugar levels in diabetic patients.
BIOTRANSFORMATION OF SESTERTERPENE
BIOTRANSFORMATION OF TRITERPENE
Biotransformation of oleanolic acid (117)
Biotransformation of a pentacyclic triterpene, oleanolic acid (117) with Fusarium lini yielded a new compound, 2α, 3β, 11β-trihydroxyolean-12-en-28-oic acid (118) (4%), and the known metabolite, 2α,3β-dihydroxyolean-12-en-28-oic acid (119) (3%)[43] [Figure 27]. Compounds 117 (IC50 = 12.8 ± 0.00 μM), 118 (IC50 = 444.0 ± 8.0 μM), and 119 (IC50 = 666.0 ± 20.0 μM) showed a significant α-glucosidase inhibitory activity, as compared to the standard, drug acarbose (IC50 = 780.0 ± 0.28 μM).
Biotransformation of 18-glycyrrhetinic acid (120)
Biotransformation of 18-glycyrrhetinic acid (120) with Cunninghamella elegans yielded the metabolite, 3, 7-dihydroxy-11-oxo-olean-12-en-30-oic acid (121) (4%), while fermentation of 120 with Fusarium lini afforded the metabolite, 3, 11-dioxoolean-12-en-30-oic acid (122) (2.5%)[44] [Figure 28]. Compounds 120 (IC50 = 225.1 ± 0.5 μM), 121 (IC50 ≥ 300 μM), and 122 (IC50 = 144.2 ± 0.2 μM) exhibited good lipoxygenase (LOX) inhibitory activity, as compared to the standard drug, baicalein (IC50 = 22.4 ± 0.5 μM). LOX inhibitors are used for the treatment of various diseases, i.e., asthma, inflammation, cancer, and some autoimmune diseases.
BIOTRANSFORMATION OF ANABOLIC-ANDROGENIC STEROIDS
Biotransformation of testosterone (123)
Biotransformation of the male steroidal hormone testosterone (123) with Curvularia lunata yielded 17-dehydrotestosterone (124) (2.1%), while Pleurotus ostreatus-assisted transformation of 123 afforded 15α-hydroxytestosterone (125) (2.9%)[45] [Figure 29]. Four derivatives, testolactone (126) (4.6%) (anti-cancer drug), 11α-hydoxytestolactone (127) (0.5%), 11α-hydroxyandrost-4-en-3, 17-dione (128) (0.3%), and 17β-hydroxy-androst-4-en-3, 6-dione (129) (1%) were synthesized through the biotransformation of 123 with Rhizopus stolonifer[46] [Figure 29]. Similarly, biotransformation of 123 with Fusarium lini afforded four derivatives, 17β,11α-dihydroxy-androst-4-en-3-one (130) (0.6%), 17β,11α-dihydroxy-androst-1, 4-dien-3-one (131) (1.2%), 11α-hydroxy-androst-1, 4-dien-3,17-dione (132) (3.1%), and androst-1, 4-dien-3, 17-dione (133) (2.5%)[46] [Figure 29] [Proposed Supplementary Figure 6].
Biotransformation of dihydrotestosterone (134)
Four derivatives, 1α-hydroxy-androst-1, 4-dien-3, 17-dione (132) (2.2%), and androst-1, 4-dien-3, 17-dione (133) (2.4%), 17β-hydroxyandrosta-1, 4-dien-3-one (135) (1.9%) [Supplementary Figure 5], and 7α, 17β-di-hydroxyandrosta-1, 4-dien-3-one (136) (1.6%), were synthesized via Fusarium oxysporum-mediated transformation of the male steroidal hormone dihydrotestosterone (134)[47] [Figure 30] [Proposed Supplementary Figure 6].
Biotransformation of androsterone (137)
Trichothecium roseum-catalyzed biotransformation of another steroidal hormone androsterone (137) afforded 3α, 17β-dihydroxyandrostane (138) (21.5%)[48] [Figure 31] [Supplementary Figure 6].
Biotransformation of trans-androsterone (139)
Transformed product 3β, 7β-dihydroxy-5α-androstan17-one (140) (5.1%) was obtained from the fermentation of steroidal hormone trans-androsterone (139) with the Rhizopus stolonifer fungal culture, while two derivatives 6β-hydroxy-5α-androstan-3, 17-dione (141) (0.5%) and 3β, 6β-dihydroxy-5α-androstan-17-one (142) (0.5%) were isolated from the transformation of substrate 139 with the fungal culture of Fusarium lini[49] [Figure 32].
Biotransformation of dehydroepiandrosterone (143)
Fermentation of dehydroepiandrosterone (DHEA) (143) with the fungal cell culture of Cephalosporium aphidicola resulted in two derivatives, 3β-hydroxy androst-4-en-17-one (144) (14%), and 3β, 4β-dihydroxyandrost-5-en-17-one (145) (17.3%)[48] [Figure 33]. Similarly, biotransformation of DHEA (143) with another fungal cell culture Rhizopus stolonifer yielded seven metabolites, 3β, 17β-dihydroxyandrost-5-ene (146) (20%), 3β, 17β-dihydroxyandrost-4-ene (147) (12%), 17β-hydroxyandrost-4-ene-3-one (148) (34%), 3β, 11β-dihydroxyandrost-4-ene-17-one (149) (15%), 3β, 7α-dihydroandrost-5-ene-17-one (150) (12%), 3β, 7α, 17β-trihydroxyandrost-5-ene (151) (20%), and 11β-hydroxyandrost-4, 6-diene-3, 17-dione (152) (15%)[50] [Figure 33]. The metabolites, 5β-androstane-3, 17-dione (153), 5α-androstane-3, 17-dione (154), androst-4-ene-3, 17-dione (155), and 17β-hydroxyandrost-4-en-3-one (156) were isolated through the biotransformation of 143 with plant cell culture Codiaeum variegatum[51] [Figure 34].
Figure 33. Microbial transformation of DHEA (143) with Rhizopus stolonifer, and Cephalosporium aphidicola. DHEA: dehydroepiandrosterone.
Figure 34. Biotransformation of DHEA (143) with Cephalosporium aphidicola, Rhizopus stolonifer, and Codiaeum variegatum. DHEA: dehydroepiandrosterone.
In addition, M. phaseolina-catalyzed transformation of DHEA (143) resulted in the synthesis of eight metabolites 146, 150, 154-156, 17β-hydroxy-androst-4,6-diene-3-one (157), 3β-hydroxy-androst-4-en-6, 17-dione (158), and 3β, 7β, 17β-trihydroxy-androst-4-en (159)[52] [Figure 34]. Compounds 143 (IC50 = 77.9 ± 1.95 μM), 150 (IC50 = 373.5 ± 9.57 μM), 152 (IC50 = 430 ± 7.13 μM), 154 (IC50 = 221.6 ± 12.5 μM), and 155 (IC50 = 191.4 ± 1.17 μM) showed significant activity against β-glucuronidase enzyme, while metabolites 146, 150, 155, and 157 were found to be inactive. β-Glucuronidase is an enzyme that catalyzes the hydrolysis of glucuronides, and generates free toxins. As a result, endogenous exposure of the organs to carcinogens increases. Its inhibition, therefore, has therapeutic significance against diseases such as colorectal carcinoma, GI tract infections, etc.
Biotransformation of boldione (160)
Biotransformation of the steroidal anabolic compound boldione (160) with the fungus Cephalosporium aphidicola afforded six metabolites, androst-4-ene-3, 17-dione (161) (2.0%), 17β-hydroxyandrosta-1, 4-diene-3-one (162) (4.1%), 11α-hydroxyandrosta-1, 4-diene-3, 17-dione (163) (3.1%), 11α-hydroxyandrost-4-ene-3, 17-dione (164) (2.5%), 11α, 17β-dihydroxyandrost-4-ene-3-one (165) (5.3%), and 11α, 17β-dihydroxyandrosta-1, 4-diene-3-one (166) (2.5%) [Figure 35]. Metabolites 161–166 were also obtained via the fermentation of 160 with Fusarium lini[53].
Biotransformation of adrenosterone (167)
Microbial transformation of adrenosterone (167) with Cephalosporium aphidicola yielded three metabolites, androsta-1, 4-diene-3, 11, 17-trione (168) (11.2%), 17β-hydroxyandrost-4-ene-3, 11-dione (169) (8.1%), and 17β-hydroxyandrosta-1, 4-diene-3, 11-dione (170) (36.8%)[54] [Figure 36]. In addition, three new compounds, 9α-hydroxy-androsta-1-ene-3, 11, 17-trione (171) (3.3%), 9α, 17β-dihydroxy-androsta-1-ene-3, 11-dione (172) (12.4%), and 6β, 17β-dihydroxy-androsta-1-ene-3, 11-dione (173) (13.7%), along with the known compound 6β-hydroxy-androsta-1-ene-3, 11, 17-trione (174) (4.2%) were also synthesized via the biotransformation of 167 with Cunninghamella elegans[55] [Figure 36].
Biotransformation of nandrolone (175)
Rhizopus stolonifer-assisted transformation of nandrolone (175) yielded the new compound 6α, 17β-dihydroxy-19-norandrost-1, 4-dien-3-one (176) (20%), along with the known compound, 19-norandrost-4-en-3, 17-dione (177) (34%)[56] [Figure 37].
Figure 37. Microbial transformation of nandrolone (175) with Cunninghamella blakesleeana, Cunninghamella echinulata, and Rhizopus stolonifer.
Three new derivatives, 10β, 12β, 17β-trihydroxy-19-nor-4-androsten-3-one (178) (0.2%), 10β, 16α, 17β-trihydroxy-19-nor-4-androsten-3-one (179) (1.5%), and 6β, 10β, 17β-trihydroxy-19-nor-4-androsten-3-one (180) (0.5%), along with four known metabolites, 10β, 17β-dihydroxy-19-nor-4-androsten-3-one (181) (0.25%), 10β-hydroxy-19-nor-4-androsten-3, 17-dione (182) (0.91%), and 16β, 17β-dihydroxy-19-nor-4-androsten-3-one (183) (0.83%)[57] [Figure 37]. Compounds 175 (IC50 = 32.0 ± 0.5 μM), 178 (IC50 ≥ 100 μM), 179 (IC50 = 77.39 ± 5.52 μM), 180 (IC50 = 70.90 ± 1.16 μM), 181 (IC50 = 54.94 ± 1.01 μM), 182 (IC50 = 80.23 ± 3.39 μM), and 183 (IC50 = 61.12 ± 1.39 μM) exhibited significant anti-leishmanial activity in vitro against Leishmania major. Leishmaniasis is a parasitic neglected tropical disease (NTD) affecting millions of people in over 80 countries in the global south. It causes self-healing lesions to be single and large skin ulcers.
Biotransformation of oxandrolone (184)
Rhizopus stolonifer-assisted transformation of the steroidal lactone, oxandrolone (184) yielded three new metabolites, 11α,17β-dihydroxy-2-oxa-androstan-3-one (185) (25%), 6α, 17β-dihydroxy-2-oxa-androstan-3-one (186) (5.0%), and 9α, 17β-dihydroxy-2-oxa-androstan-3-one (187) (8.0%) [Figure 38]. Compounds 185 (IC50 = 190.3 ± 1.18 μM) and 187 (IC50 = 482.66 ± 6.86 μM) showed weak inhibition of β-glucuronidase, as compared to the standard inhibitor, D-saccharic acid 1, 4 lactone (IC50 = 48.4 ± 1.25 μM)[58].
Figure 38. Microbial transformation of oxandrolone (184) with Cunninghamella blakesleeana, M. phaseolina, Glomerella fusarioides, and Rhizopus stolonifer.
A new metabolite, 12β,17β-dihydroxy-17α-methyl-2-oxa-5α-androstan-3-one (188) (3.4%), was synthesized via the transformation of substrate 184 with Cunninghamella blakesleeana[59] [Figure 38]. Similarly, structural transformation of oxandrolone (184) with Macrophomina phaseolina afforded four new metabolites, 11β, 17β-dihydroxy-17α-(hydroxymethyl)-2-oxa-5α-androstan-3-one (189) (2.5%), 5α, 11β, 17β-trihydroxy-17α-methyl-2-oxa-androstan-3-one (190) (1.0%), 17β-hydroxy-17α-methyl-2-oxa-5α-androstan-3, 11-dione (191) (1.5%), and 11β, 17β-dihydroxy-17α-methyl-2-oxa-5α-androstan-3-one (192) (3.0%)[59] [Figure 38]. Glomerella fusarioides-mediated transformation of oxandrolone (184) also yielded a new compound, 17β, 11α-dihydroxy-17α-methylandrosta-2-oxa-4-ene-3-one (193) (3.8%)[60] [Figure 38]. The new metabolite 193 showed a remarkable aromatase inhibitory activity with an IC50 = 0.6 ± 0.005 µM, as compared to the substrate 184 (IC50 = 0.808 ± 0.07 µM), and standard anti-breast cancer drug exemestane (IC50 = 0.232 ± 0.031 µM)[60]. Aromatase is an enzyme that catalyzes the production of estrogen through the aromatization of steroidal ring-A, and thus helps in the proliferation of breast cancer cells. Its inhibition is the standard treatment of ER+ breast cancers.
Biotransformation of mesterolone (194)
Mesterolone (194) is a steroidal anabolic-androgenic drug used for the treatment of disorders in men where their bodies cannot produce enough natural androgens. Eight metabolites, 1α-methylandrostane-3, 17-dione (195) (1.2%), 1-methylandrost-1-en-3, 17-dione (196) (0.25%), 6α, 17β-dihydroxy-1α-methylandrosta-3-one (197) (0.36%), 7α, 17β-dihydroxy-1α-methylandrosta-3-one (198) (0.60%), 11α, 17β-dihydroxy-1α-methylandrosta-3-one (199) (0.55%), 15α-hydroxy-1α-methylandrosta-3, 17-dione (200) (1.05%), 15α, 17β-dihydroxy-1α-methylandrosta-3-one (201) (0.86%), 15α, 17β-dihydroxy-1-methylandrosta-1en-3-one (202) (0.37%), and 3β, 17β-dihydroxy-1α-methylandrostane (203) (0.65%) of mesterolone (194) were synthesized by using Cephalosporium aphidicola, Fusarium lini, and Rhizopus stolonifer fungal cell cultures. Metabolites 197-202 were found to be new[61] [Figure 39]. Compounds 194, 196, 199, 200, 201, and 202 showed significant anti-inflammatory activity with the IC50 values of 202, 117, 250, 183, 295, 50, and 127 μM, respectively, as compared to the standard drug prednisolone (IC50 = 83 μM). Metabolite 195 was found to be inactive[61].
Figure 39. Microbial transformation of mesterolone (194) with Cephalosporium aphidicola, Rhizopus stolonifer, and Fusarium lini.
Similarly, Cunninghamella blakesleeana-mediated fermentation of mesterolone (194) (0.60%) yielded seven new compounds, 1α-methyl-11β, 14α, 17β-trihydroxy-5α-androstan-3-one (204), 1α-methyl-7β, 17β-dihydroxy-5α-androstan-3-one (205) (0.70%), 1α-methyl-17β-hydroxy-5α-androstan-3, 7-dione (206) (1.0%)[62] [Figure 40], 1α-methyl-1β, 11α, 17β-trihydroxy-5α-androstan-3-one (207) (0.90%), 1α-methyl-7α, 11β,17β-trihydroxy-5α-androstan-3-one (208) (0.40%), 1α-methyl-1β, 6α, 17β-trihydroxy-5α-androstan-3-one (209) (0.40%), and 1α-methyl-1β, 11β, 17β-trihydroxy-5α-androstan-3-one (210) (0.50%), along with three known metabolites, 197, 198, and 199[63] [Figure 40]. Fermentation of mesterolone (194) with Macrophomina phaseolina afforded the new compound, 1α-methyl, 17β-hydroxy-5α-androstan-3, 6-dione (211) (0.40%)[63] [Figure 40].
Biotransformation of mibolerone (212)
Mibolerone (212) is a potent synthetic anabolic steroid, marketed by Upjohn Company (USA) under the brand name Cheque Drops, for the treatment of estrous (heat) in female dogs. Biotransformation of mibolerone (212) was carried out at room temperature using Cunninghamella echinulata, and Macrophomina phaseolina. This afforded six new metabolites, 10β, 17β-dihydroxy-7α, 17α-dimethylestr-4-en-3-one (213) (4.0%), 6β, 17β-dihydroxy-7α, 17α-dimethylestr-4-en-3-one (214) (2.0%), 6β, 10β, 17β-trihydroxy-7α, 17α-dimethylestr-4-en-3-one (215) (30.0%), 11β, 17β, 20-trihydroxy-7α, 17α-dimethylestr-4-en-3-one (216) (0.4%), 1α, 17β-dihydroxy-7α, 17α-dimethylestr-4-en-3-one (217) (0.24%), 1α, 11β, 17β-trihydroxy-7α, 17α-dimethylestr-4-en-3-one (218) (0.34%), and a known metabolite, 11β, 17β-dihydroxy-7α, 17α-dimethylestr-4-ene-3-one (219) (3.0%)[64] [Figure 41].
Biotransformation of metenolone acetate (220)
Metenolone acetate (220) is another synthetic steroidal anabolic drug, sold under the brand names Nibal and Primobolan Depot, for the treatment of anemia. Drug 220 is also used by athletes, and for sports animals, to enhance their muscular strength and physical performances. Microbial transformation of metenolone acetate (220) was carried out under ambient reaction conditions using Rhizopus stolonifer, Fusarium lini, Cunninghamella elegans, and Aspergillus alliaceous. This afforded fourteen transformed products, 6α-hydroxy-1-methyl-3-oxo-5α-androst-1-en-17-yl acetate (221) (1.1%), 6α, 17β-dihydroxy-1-methyl-3-oxo-5α-androst-1-en (222) (1.0%), 7β-hydroxy-1-methyl-3-oxo-5α-androst-1-en-17-yl acetate (223) (0.50%), 15β, 20-dihydroxy-1-methyl-3-oxo-5α-androst-1-en-17-yl acetate (224) (2.6%), 15β-hydroxy-1-methyl-3-oxo-5α-androst-1-en-17-yl acetate (225) (1.0%), 17β-hydroxy-1-methyl-3-oxoandrosta-1,4-dien (226) (0.40%) [Figure 42], 17β-hydroxy-1-methyl-3-oxo-5α-androst-1-en (227) (7.1%)[65], 17β-hydroxy-1-methyl-3-oxo-5β-androst-1-en (228) (0.84%), 1-methyl-5β-androst-1-en-3,17-dione (229) (0.30%), 12β, 17β-dihydroxy-1-methyl-3-oxoandrosta-1, 4-dien (230) (0.70%), 1-methyl-androsta-1, 4-dien-3, 17-dione (231) (24.4%), 17β-hydroxy-1α-methyl-5α-androstan-3-one (232) (0.45%), 17β, 15α-dihydroxy-1α-methyl-5α-androstan-3-one (233) (1.0%), and 7β, 15β, 17β-trihydroxy-1-methyl-3-oxo-5α-androst-1-en (234) (0.54%). Among them, compounds 221-225, 230, and 234 were identified as new[65] [Figure 43], Compounds 220 (62.5% ± 4.4%), 221 (73.4% ± 0.6 %), 224 (81.0% ± 2.5 %), 227 (69.7% ± 1.4%), 229 (73.2% ± 0.3%), 232 (60.1% ± 3.3%), and 233 (71.0% ± 7.2%) showed good inhibition of cytokine (TNF-α) production. Compounds 223 (53.7% ± 1.4 %), 226 (46.6% ± 5.2 %), and 234 (52.9% ± 2.4%) showed moderate activity, compounds 222 (33.5% ± 6.6%), and 225 (37.8% ± 1.1%) showed a weak activity, while metabolites 228, 234, and 235 were found inactive. Compounds 222 (IC50 = 4.4 ± 0.01 µg/mL), and 224 (IC50 = 10.2 ± 0.01 µg/mL) showed significant activity against T-cells proliferation, in contrast to the standard drug, prednisolone (IC50 = 3.51 ± 0.03 µg/mL) in vitro[65]. TNF- α, and T-cells are essential components of innate inflammatory cascade, and their inhibition is used for the treatment of chronic inflammations.
Figure 42. Microbial transformation of metenolone acetate (220) with Aspergillus alliaceous, and Rhizopus stolonifer.
Biotransformation of metenolone enanthate (235)
Four new derivatives of steroidal anabolic drug, metenolone enanthate (235), namely 17β-hydroxy-1-methyl-5α-androst-1-ene-3, 16-dione (236), 15β, 17β -dihydroxy-1-methyl-5α-androstan-1-ene-3-one (237), 12β, 17β -dihydroxy-1-methyl-5α-androstan-1-ene-3-one (238), and 16β, 17β-dihydroxy-1-methyl-5α-androstan-1-ene-3-one (239), were obtained through biotransformation of drug 235 with Aspergillus niger[66] [Figure 44]. The metabolites 236 and 237 showed potent inhibition of ROS production by whole blood with the IC50 values of 8.60 ± 1.0 and 7.05 ± 1.3 μg/mL, respectively, while drug 235 was found to be inactive. Compounds 236 and 237 also showed potent activity against isolated polymorphonuclear leukocytes (PMNs) with the IC50 values of 14.0 ± 1.7 and 4.70 ± 0.5 μg/mL, respectively.
Biotransformation of danazol (240)
Cunninghamella blakesleeana-catalyzed transformation of the anabolic steroidal drug, danazol (240), afforded three new metabolites, 15β, 17β-dihydroxy-2-(hydroxymethyl)-17α-pregn-4-en-20-yn-3-one (241) (1.0%), 1α, 17β-dihydroxy-17α-pregna-2, 4-dien-20-yno-[2, 3-d]-isoxazole (242) (1.2%), 6β, 17β-dihydroxy-17α-pregna-2, 4-dien-20-yno-[2,3-d]-isoxazole (243) (0.8%), along with the known metabolite, 17β-hydroxy-2-(hydroxymethyl)-17α-pregn-1, 4-dien-20-yn-3-one (244) (1.2%)[67] [Figure 45]. Compound 241 showed potent cytotoxicity against HeLa (cervical) cancer cell line with the IC50 = 0.283 ± 0.013 μM, as compared to the standard anti-cancer drug, doxorubicin (IC50 = 0.506 ± 0.015 μM), where compound 242 was identified as significantly active (IC50 = 13.42 ± 0.819 μM)[67].
Biotransformation of dianabol (245)
Biotransformation of another anabolic steroidal drug dianabol (245) with Cunninghamella elegans afforded five new metabolites, 6β, 17β-dihydroxy-17α-methylandrost-1, 4-dien-3-one (246) (3.7%), 15α, 17β-dihydroxy-17α-methylandrost-1, 4-dien-3-one (247) (18.6%), 11α, 17β-dihydroxy-17α-methylandrost-1, 4-dien-3-one (248) (13.0%), 6β, 12β, 17β-trihydroxy-17α-methylandrost-1, 4-dien-3-one (249) (4.0%), 6β, 15α, 17β-trihydroxy-17α-methylandrost-1, 4-dien-3-one (250) (3.2%)[68] [Figure 46]. Three new metabolites, 17β-hydroxy-17α-methylandrost-1,4-dien-3,6-dione (251) (1.2%), 7β, 17β-dihydroxy-17α-methylandrost-1, 4-dien-3-one (252) (11.0%), and 15β, 17β-dihydroxy-17α-methylandrost-1, 4-dien-3-one (253) (3.0%), along with the known metabolite, 11β, 17β-dihydroxy-17α-methylandrost-1, 4-dien-3-one (254) (1.7%) were synthesized by Macrophomina phaseolina-assisted biotransformation of drug 245. The metabolite 247 showed a remarkable β-glucuronidase inhibitory activity (IC50 = 60.7 μM), as compared to the standard, D-saccharic acid-1, 4-lactone (IC50 = 48.4 μM)[68].
Biotransformation of methasterone (255)
Five new derivatives, 7α, 17β-dihydroxy-2α, 17α-dimethyl-5α-androstane-3-one (256) (2.0%), 7α, 16β, 17β-triihydroxy-2α, 17α-dimethyl-5α-androstane-3-one (257) (0.7%), 5α, 12β, 17β-trihydroxy-2α, 17α-dimethyl-5α-androstane-3-one (258) (1.0%), 7α, 12β, 17β-triihydroxy-2α, 17α-dimethyl-5α-androstane-3-one (259) (1.5%), and 7α, 9α, 17β-triihydroxy-2α, 17α-dimethyl-5α-androstane-3-one (260) (0.5%) were isolated through biotransformation of steroidal anabolic drug, methasterone (255) with Cunninghamella blakesleeana. Likewise, six new derivatives, 6β, 17β-dihydroxy-2, 17α-dimethylandrosta-1, 4, 14-triene-3-one (261) (1.0%), 15α, 17β-dihydroxy-2, 17α-dimethylandrosta-1, 4-diene-3-one (262) (0.6%), 6β,17β-dihydroxy-2, 17α-dimethylandrosta-1, 4-diene-3-one (263) (0.4%), 14α, 15α-dihydroxy-2, 17-dimethylandrosta-1, 4, 16-triene-3-one (264) (0.3%), 17β-hydroxy-2, 17α-dimethylandrosta-1, 4-diene-3, 6-dione (265) (0.3%), 17β-hydroxy-2, 17α-dimethylandrosta-1, 4-diene-3-one (266) (1.0%) were synthesized by Fusarium lini transformation of drug 255[69] [Figure 47]. The metabolite 259 showed a potent inhibition against TNF-α production with the IC50 value of 8.1 ± 0.9 μg/mL, as compared to the standard drug, pentoxifylline (IC50 = 94.8 ± 2.1 μg/mL). Derivatives 259 (86.7% ± 2.3%) and 266 (62.5% ± 1.5%) also showed an excellent inhibition of NO proliferation, as compared to the standard NG-monomethyl-l-arginine acetate (65.6% ± 1.1%)[69].
Figure 47. Biotransformation of methasterone (255) with Cunninghamella blakesleeana and Fusarium lini.
Further, a new compound, 6β, 9α, 17β-trihydroxy-2α, 17α-dimethyl-5α-androstane-3-one (267), was obtained through the biotransformation of compound 255 with Macrophomina phaseolina[69]. While four new compounds, 6β, 7β, 17β-trihydroxy-2α, 17α-dimethyl-5α-androstane-3-one (268) (0.54%), 6β, 7α, 17β-trihydroxy-2α, 17α-dimethyl-5α-androstane-3-one (269) (0.53%), 6α, 17β-dihydroxy-2α, 17α-dimethyl-5α-androstane-3, 7-dione (270) (0.51%), and 3β, 6β, 17β-trihydroxy-2α, 17α-dimethyl-5α-androstane-7-one (271) (0.53%) were synthesized by biotransformation of drug 255 with Cunninghamella blakesleeana[70] [Figure 48]. Metabolites 267 and 268 showed moderate inhibition of NO production with the IC50 values of 40.2 ± 3.3, and 38.1 ± 0.5 μg·mL−1, respectively.
BIOTRANSFORMATION OF CONTRACEPTIVE STEROIDS
Biotransformation of desogestrel (272)
Whole-cell bio-catalytic conversion of steroidal contraceptive drug desogestrel (272) by Cunninghamella blakesleeana yielded three new metabolites, 13-ethyl-11-methylene-18, 19-dinor-17α-pregn-4-en-20-yn-6β, 15β, 17β-triol (273) (2.5%), 13-ethyl-11-methylene-18, 19-dinor-17α-pregn-4-en-20-yn-3β, 6β, 17β-triol (274) (15.2%), and 13-ethyl-11-methylene-18, 19-dinor-17α-pregn-20-yn-3α, 5α, 6β, 17β-tetraol (275) (1.9%), along with the known metabolite, 13-ethyl-11-methylene-18, 19-dinor-17α-pregn-4-en-20-yn-6β, 17β-dihydroxy-3-one (276) (9.2%)[71] [Figure 49]. Compounds 272 and 273 showed potent activity against Staphylococcus aureus EMRSA-17, Staphylococcus aureus NCTC 13277 (MRSA-252), and Staphylococcus aureus NCTC 13143, and clinically isolated Pakistani strain of Staphylococcus aureus in an in-vitro MABA (Microplate Alamar Blue) assay.
Biotransformation of ethisterone (277)
Cunninghamella elegans-assisted transformation of another steroidal contraceptive compound, ethisterone (277), afforded a new derivative, 17α-ethynyl-11α, 17β-dihydroxyandrost-4-en-3-one (278) (5.5%)[72] [Figure 50]. Biotransformation of the drug 277 with Cunninghamella blakesleeana afforded two new metabolites, 17α-ethynyl-6β, 15β, 17β-trihydroxyandrost-4-en-3-one (279) (0.80%) and 17α-ethynyl-7β, 15β, 17β-trihydroxyandrost-4-en-3-one (280) (0.30%), while fermentation of ethisterone (277) with Aspergillus niger yielded a new metabolite, 17α-ethynyl-6α, 17β-dihydroxyandrost-4-en-3-one (281) (0.60%), along with the known metabolite, 17α-ethynyl-11α, 17β-dihydroxyandrost-4-en-3-one (282) (0.30%)[73] [Figure 50].
Figure 50. Microbial transformation of ethisterone (277) Cunninghamella blakesleeana,Cunninghamella elegans and Macrophomina phaseolina.
Two new derivatives, 17α-ethyl-11α, 17β-dihydroxyandrost-4-en-3-one (284) (0.80%), and 17α-ethyl-6α, 17β-dihydroxy-5α-androstan-3-one (285) (60.0%) were also synthesized through the biotransformation of 17α-ethyl-17β-hydroxyandrost-4-en-3-one (283) with C. elegans[72] [Figure 51]. Derivatives 278 (IC50 = 5.95 ± 0.00078 μM), 284 (IC50 = 3.46 ± 0.01046 μM), and 285 (IC50 = 1.72 ± 0.00089 μM) showed remarkable tyrosinase inhibitory activity, as compared to the substrates 277 (IC50 = 2.61 ± 0.037328 μM), 283 (IC50 = 1.53 ± 0.001088 μM)[72]. Tyrosinase is an enzyme involved in the melanin biosynthesis; therefore, its inhibitors are used in the prevention of excessive production dermal melanin.
Biotransformation of mestranol (286)
Biotransformation of an oral steroidal contraceptive drug, mestranol (286), with the fungal culture of Cunninghamella elegans afforded a new compound, 6β, 12β-dihydroxymestranol (287) (3.6%), and the known derivative, 6β-hydroxymestranol (288) (2.7%)[74] [Figure 52].
Biotransformation of methyloestrenolone (289)
Six transformed products, 17α-methyl-6β,17β-dihydroxyestr-4-en-3-one (290) (1.8%), 17α-methyl-11β, 17β, 20-trihydroxyestr-4-en-3-one (291) (0.8%), 17α-methyl-2α, 11β, 17β-trihydroxyestr-4-en-3-one (292) (0.6%), 17α-methyl-1β, 17β-dihydroxyestr-4-en-3-one (293) (4.5%), 17α-methyl-11α, 17β-dihydroxyestr-4-en-3-one (294) (0.45%), and 17α-methyl-11β, 17β-dihydroxyestr-4-en-3-one (295) (1.4%) were synthesized via biotransformation of a steroidal contraceptive drug, methyloestrenolone (289) with Macrophomina phaseolina. Compounds 290-295 were found to be new compounds[75] [Figure 53]. Two known derivatives, 17α-methyl-10β, 17β-dihydroxyestr-4-en-3-one (296) (0.8%), and 17α-methyl-17β, 20-dihydroxyestr-4-en-3-one (297) (1.2%) were obtained through biocatalysis of 289 with Aspergillus niger[75] [Figure 54].
Biotransformation of drospirenone (298)
Drospirenone (298) is an orally active contraceptive drug, marketed under the brands Sylnd and Yasmin. Cunninghamella elegans-catalyzed transformation of drospirenone (298) afforded four new metabolites, 6β, 7β, 15β, 16β-dimethylene-3-oxo-14α-hydroxy-17α-pregn-4-ene-21, 17-carbolactone (299) (3.1%), 6β,7β,15β,16β-dimethylene-3, 11-dioxo-17α-pregn-4-ene-21, 17-carbolactone (300) (4.2%), 6β, 7β, 15β, 16β-dimethylene-3, 12-dioxo-17α-pregn-4-ene-21, 17-carbolactone (301) (2.9%), and 6β, 7β, 15β, 16β-dimethylene-3-oxo-11β, 14α-dihydroxy-17α-pregn-4-ene-21, 17-carbolactone (302) (6.2%), along with the known metabolite, 6β, 7β, 15β, 16β-dimethylene-3-oxo-11α-dihydroxy-17α-pregn-4-ene-21, 17-carbolactone (303)[76] [Figure 55].
Biotransformation of etonogestrel (304)
Biotransformation of another contraceptive drug (brands: Nexplanon and Implanon) etonogestrel (304) with Cunninghamella blakesleeana afforded three new metabolites, 6β-hydroxy-11, 22-epoxy-etonogestrel (305) (3.2%), 11, 22-epoxy-etonogestrel (306) (1.5%), 10β-hydroxy-etonogestrel (307) (4.6%), and two known compounds, 6β-hydroxy-etonogestrel (308) (3.4%), and 14α-hydroxy-etonogestrel (309) (2.7%). Compounds 305, 307, and 309 were also obtained from the fermentation of 304 with Cunninghamella echinulata[77] [Figure 56]. Derivative 308 was found to be significantly active against β-glucuronidase enzyme with the IC50 value of 13.97 ± 0.12 μM, as compared to the standard drug, D-saccharic acid 1,4-lactone (IC50 = 45.75 ± 2.16 μM)[77].
Biotransformation of ethynodiol diacetate (310)
Biotransformation of another synthetic steroidal contraceptive drug, ethynodiol diacetate (310), with the fungus Cunninghamella elegans yielded three new derivatives, 17α-ethynylestr-4-en-3β, 17β-diacetoxy-6α-ol (311) (0.5%), 17α-ethynylestr-4-en-3β, 17β-diacetoxy-6β-ol (312) (1.0%), and 17α-ethynylestr-4-en-3β, 17β-diacetoxy-10β-ol (313) (0.5%), and the known metabolite, 17α-ethynyl-17β-acetoxyestr-4-en-3-one (314) (1.4%). In addition, four known metabolites, 314, 17α-ethynyl-17β-hydroxyestr-4-en-3-one (315) (3.3%), 17α-ethynyl-3β-hydroxy-17β-acetoxyestr-4-ene (316) (0.58%), and 17α-ethynyl-5α, 17β-dihydroxyestr-3-ene (317) (0.45%) were also obtained by the biotransformation of drug 310 with the plant cell culture of Ocimum basilicum[78] [Figure 57].
BIOTRANSFORMATION OF ANTI-CANCER STEROIDS
Biotransformation of exemestane (318)
Exemestane (318) is a steroidal-based aromatase inhibitor for the treatment of estrogen-dependent (ER+) breast cancers. Drug 318 is marketed under the brand name Aromasin. Microbial transformation of exemestane (318) afforded three new derivatives, 11α-hydroxy-6-methylene-androsta-1,4-diene-3,17-dione (319) (0.4%), 16β,17β-dihydroxy-6-methylene-androsta-1,4-diene-3-one (320) (1.0%), and 17β-hydroxy-6-methylene-androsta-1,4-diene-3, 16-dione (321) (0.5%), and the known metabolite, 17β-hydroxy-6-methylene-androsta-1, 4-diene-3-one (322) (0.6%)[79] [Figure 58]. The metabolite 319 showed moderate cytotoxicity against PC-3 (prostrate) (IC50= 16.83 ± 0.96 μM), and HeLa (cervical) (IC50 = 24.87 ± 0.72 μM) cancer cell lines.
Figure 58. Microbial transformation of exemestane (318) with Macrophomina phaseolina, and Fusarium lini.
Six metabolites, 6-methylene-5α-androstane-3β, 16β, 17β-triol (323), 17β-hydroxy-6-methyleneandrosta-4-ene-3-one (324), 6α-spiroxirandrost-4-ene-3, 17-dione (325), 6-methyleneandrosta-4-ene-3, 17-dione (326), 6β,17β-dihydroxyandrost-4-en-3-one (327), and 17β-hydroxy-6α-spiroxirandrost-1, 4-diene-3-one (328), were obtained from the Cunninghamella blakesleeana-catalyzed transformation of drug 318[79] [Figure 59]. Two derivatives, 17β-hydroxy-6α-hydroxymethylandrosta-1, 4-dien-3-one (329) and 6α-hydroxymethylandrosta-1, 4-diene-3,17-dione (330), were synthesized via the fermentation of 318 with Curvularia lunata[80] [Figure 60]. 17β-Hydroxy-6-methyleneandrosta-1, 4-diene-3, 16-dione (331) was obtained by the biotransformation of 318 with Aspergillus niger[80] [Figure 60]. Fermentation with Gibberella fujikuroi afforded two derivatives, 6α-hydroxy-4-androstene-3, 17-dione (332) and 6α-hydroxymethylandrost-4-ene-3, 17-dione (333). Metabolites 324 and 330 were found to be new compounds[80] [Figure 60]. The derivative 325 showed moderate cytotoxicity against MCF-7 breast cancer cell line with an IC50 of 33.43 ± 4.01 μM, in comparison to the standard anti-cancer drug, doxorubicin (IC50 = 0.92 ± 0.1 μM)[80].
Biotransformation of drostanolone enanthate (334)
Five new transformed products, 2α-methyl-3α,14α,17β-trihydroxy-5α-androstane (335) (0.8%), 2-methylandrosta-11α-hydroxy-1, 4-diene-3,17-dione (336) (0.9%), 2-methylandrosta-14α-hydroxy-1, 4-diene-3, 17-dione (337) (1.2%), 2α-methyl-7α-hydroxy-5α-androstan-3, 17-dione (340) (1.1%), and 2-methyl-5α-androsta-7α-hydroxy-1-ene-3, 17-dione (341) (0.75%), along with three known metabolites, 2α-methyl-3α, 17β-dihydroxy-5α-androstane (338) (3.5%), 2-methylandrosta-1, 4-diene-3, 17-dione (339) (4.2%), and 2α-methyl-5α-androsta-17β-hydroxy-3-one (342) (0.5%) were produced by the biotransformation of the anti-cancer drug, drostanolone enanthate (334), with Cephalosporium aphidicola and Fusarium lini[81] [Figure 61].
Figure 61. Microbial transformation of drostanolone enanthate (334) with Cephalosporium aphidicola, and Fusarium lini.
Metabolite 341 (IC50 = 19.6 ± 1.4 µM) exhibited potent activity against HeLa (cervical) cancer cells, in contrast to the parent drug 334 (IC50 = 54.7 ± 1.6 µM), and the standard drug, cisplatin (IC50 = 40.1 ± 2.0 µM). Compounds 335 (IC50 = 64.3 ± 3.0 µM), 336 (IC50 = 40.7 ± 0.9 µM), 337 (IC50 = 40.7 ± 0.9 µM), 338 (IC50 = 49.5 ± 2.2 µM), 339 (IC50 = 39.8 ± 1.5 µM), and 342 (IC50 = 30.1 ± 1.0 µM) also displayed remarkable activity against HeLa cell line. Metabolites 335 (IC50 = 58.4 ± 1.6 µM), 336 (IC50 = 59.1 ± 2.6 µM), 337 (IC50 = 60.4 ± 0.9 µM), 338 (IC50 = 51.8 ± 3.4 µM), 339 (IC50 = 68.1 ± 1.2 µM), and 340 (IC50 = 39.1 ± 2.0 µM) showed a significant anti-cancer activity against PC-3 (prostate) cells, compared to compounds 342 (IC50 = 96.2 ± 3.0 µM), 335 (IC50 = 84.6 ± 6.4 µM), 339 (IC50 = 84.0 ± 3.1 µM), and standard cisplatin (IC50 = 76.5 ± 1.2 µM). Compounds 334 (IC50 = 5.0 ± 1.2 µM), 338 (IC50 = 12.4 ± 2.3 µM), 340 (IC50 = 16.7 ± 2.6 µM), and
In addition, nine more derivatives, 2α-methyl-7α, 11β, 17β-trihydroxy-5α-androstan-3-one (343), 2α-methyl-7β, 15α, 17β-trihydroxy-5α-androstan-3-one (344), 2α-hydroxymethyl-11β, 17β-dihydroxy-5α-androstan-3-one (345), 2α-methyl-7α, 11α, 17β-trihydroxy-5α-androstan-3-one (346), 2α-methyl-11α, 15β, 17β-trihydroxy-5α-androstan-3-one (347), 2α-methyl-3β, 5α, 17β-trihydroxy-5α-androstane (348), 2α-methyl-3β, 14α, 17β-trihydroxy-5α-androstane (349), 2α-methyl-17β-hydroxy-5α-androstan-3-one (350), and 2α-methyl-3β, 17β-dihydroxy-5α-androstane (351) were also synthesized through the biotransformation of anti-cancer drug 334 with Beauveria bassiana, and Macrophomina phaseolina[82] [Figure 62].
BIOTRANSFORMATION OF GLUCOCORTICOIDS
Biotransformation of melengestrol acetate (352)
Biotransformation of the progestin medication, melengestrol acetate (352) (Brand names, Heifermax and MGA) with Glomerella fusarioides and Rhizopus stolonifer afforded four new oxidative products, 17α-acetoxy-11α-hydroxy-6-methyl-16-methylenepregna-4, 6-diene-3, 20-dione (353), 17α-acetoxy-11α-hydroxy-6-methyl-16-methylenepregna-1, 4, 6-triene-3,20-dione (354), 17α-acetoxy-6, 7α-epoxy-6β-methyl-16-methylenepregna-4, 6-diene-3, 20-dione (355), and 17α-acetoxy-11β, 15β-dihydroxy-6-methyl-16-methylenepregna-4, 6-diene-3,20-dione (356)[83] [Figure 63]. Drug 352 (IC50 = 2.77 ± 0.08 µM), and its derivatives 353 (IC50 = 2.78 ± 0.07 µM), 355 (IC50 = 2.74± 0.1 µM), and 356 (IC50 ≤ 2 µM) showed potent T- cell proliferation inhibitory activities in vitro. While derivative 253 (IC50 = 29.9 ± 0.09 µM) showed moderate activity, as compared to the standard anti-inflammatory drug, prednisolone (IC50 = 9.73 ± 0.08 µM)[83].
Biotransformation of medrysone (357)
Medrysone is a synthetic glucocorticoid, which is marketed under the brand names HMS and Medrocort for the treatment of inflammatory diseases. Seven new metabolites, 14α-hydroxy-6α-methylpregn-4-ene-3, 11, 20-trione (358) (1.88%), 6β-hydroxy-6α-methylpregn-4-ene-3, 11, 20-trione (359) (1.55%), 15β-hydroxy-6α-methylpregn-4-ene-3, 11, 20-trione (360) (1.11%), 6β, 17α-dihydroxy-6α-methylpregn-4-ene-3, 11, 20-trione (361) (1.66%), 6β, 20(S)-dihydroxy-6α-methylpregn-4-ene-3, 11-dione (362) (0.64%), 11β, 16β-dihydroxy-6α-methylpregn-4-ene-3-one (363) (0.66%), and 15β, 20(R)-dihydroxy-6α-methylpregn-4-ene-3, 11-dione (364) (1.77%) of medrysone (357) were synthesized through the microbial transformation of drug 357 with the fungal cultures of Cunninghamella blakesleeana, Neurospora crassa, and Rhizopus stolonifer[84] [Figure 64]. Compounds 357 (IC50 = 2.0 ± 0.04 μg/mL), 358 (IC50 = 20.0 ± 0.9 μg/mL), 359 (IC50 = 14.6 ± 2.1 μg/mL), 360 (IC50 = 9.2 ± 0.7 μg/mL), 361 (IC50 = 1.2 ± 0.02 μg/mL), 362 (IC50 = 15.2 ± 2.4 μg/mL), 363 (IC50 ≤ 0.2 μg/mL), and 364 (IC50 = 10.4 ± 0.42 μg/mL) showed potent anti-inflammatory activities through T-cell proliferation inhibition, as compared to the standard drug, prednisolone (IC50 ≤ 3.1 μg/mL)[84].
BIOTRANSFORMATION OF PHYTOSTEROIDS
Biotransformation of (E)-guggulsterone (365)
Guggulsterone (365) is a naturally occurring bioactive plant sterol isolated from the gum resin of guggul (Commiphora wightii). This compound dramatically reverses multi-drug resistance in a number of human cancer cell lines, extending the efficacy of existing chemotherapy. The new metabolites, 12α-hydroxypregn-4-ene-3,16-dione (366) (1.5%), (17Z)-11α-hydroxypregna-4, 17-diene-3, 16-dione (367) (2.5%), (17E)-11α-hydroxypregna-4, 17-diene-3, 16-dione (368) (2.66%), (17Z)-6β, 11α-dihydroxypregna-4, 17-diene-3, 16-dione (369) (0.34%)[85] [Figure 65], (17E)-6β, 11α-dihydroxypregna-4, 17-diene-3, 16-dione (370) (1.52%), 6α, 11 α -dihydroxypregn-4-ene-3, 16-dione (371) (1.82%), and 11α, 16β-dihydroxypregn-4-en-3-one (372) (1.92%) were synthesized through the microbial transformation of 365 with Rhizopus stolonifer and Gibberella fujikuroi[85,86] [Figure 66].
Biotransformation of physalin H (373)
Rhizopus stolonifer-catalyzed the transformation of physalin H (373) and yielded the new derivative, 6, 7-dehydrophysalin H (374) (2.10%)[87] [Figure 67], while structural transformation with Cunninghamella elegans afforded a new compound, 6-deoxyphysalin H (375) (4.10%), along with the known metabolite, isophysalin B (376) (5.25%)[87] [Figure 68]. Compounds 373 (IC50 = 6.03 ± 0.005 μM), 374 (IC50 = 7.74 ± 0.015 μM), 375 (IC50 = 6.34 ± 0.03 μM), and 376 (IC50 = 13.8 ± 0.05 μM) showed potent anti-leishmanial activity in vitro, compared with the standard drug, amphotericin B (IC50 = 0.129 ± 0.105 μM) against promastigotes of Leishmania major (DESTO).
BIOTRANSFORMATION OF NEUROSTEROIDS
Biotransformation of pregnenolone (377)
Pregnenolone (377) is a naturally produced hormone by the adrenal glands in the human body. It is the starting material in the synthesis of steroidal hormones, including cortisol, testosterone, progesterone, estrogen, etc. Biotransformation of pregnenolone (377) with the fungus Cunninghamella elegans yielded two new metabolites, 3β, 6α, 11α, 12β-tetrahydroxypreg-5-en-20-one (378) (4.05%), and 3β, 6β, 11α-trihydroxypreg-5-en-20-one (379) (2.29%), along with the known metabolite, 3β, 7β, 11α-trihydroxypreg-5-en-20-one (380) (28.1%)[88] [Figure 69]. Two more derivatives, 3β, 7β-dihydroxypreg-5-en-20-one (381) (3.03%), and 3β, 6β, 7β-trihydroxypreg-5-en-20-one (382) (2.41%) were also synthesized through the biotransformation of drug 377 with Gibberella fujikuroi[88] [Figure 70]. Similarly, microbial transformation of pregnenolone acetate (383) with the fungus Cunninghamella elegans afforded four known metabolites, pregnenolone (384) (4.0%), androsta-1, 4-diene-3, 17-dione (385) (2.04%), 6β,15β-dihydroxyandrost-4-ene-3,17-dione (386) (2.04%), and 11α, 15β-dihydroxypreg-4-ene-3, 20-dione (387) (2.30%)[88] [Figure 71].
Biotransformation 6-dehydroprogesterone (388)
6-Dehydroprogesterone (388) is a synthetic derivative of progesterone hormone. Biotransformation of 6-dehydroprogesterone (388) with the fungal cell culture of Aspergillus niger afforded three new metabolites, 6β-chloro-7α, 11α-dihydroxypregna-4-ene-3, 20-dione (389) (1.0%), 7α-chloro-6β, 11α-dihydroxypregna-4-ene-3,20-dione (390) (1.33%), and 6α, 7α-epoxy-11α-hydroxypregna-4-ene-3,20-dione (391) (1.33%), along with the two known metabolites, 6α, 7α-epoxypregna-4-ene-3,20-dione (392) (2.0%), and 11α-hydroxypregna-4, 6-diene-3,20-dione (393) (2.33%)[89] [Figure 72]. Whereas, Gibberella fujikuroi-catalyzed transformation of substrate 388 yielded the known compound, 11α, 17β-dihydroxyandrosta-4, 6-dien-3-one (394) (15.4%)[89] [Figure 73].
Biotransformation 20-hydroxymethylpregna-1,4-dien-3-one (395)
(20S)-20-Hydroxymethylpregna-1, 4-dien-3-one (395) is another neurochemical, which is obtained by microbial-catalyzed degradation of sterols. Compound 395 is also used as an intermediate in the synthesis of many steroid-based drugs. Six new metabolites, 11α-hydroxy-20- acetoxymethylpregna-1,4-dien-3-one (396) (0.40%), 17α-hydroxy-20-hydroxymethylpregna-1, 4-dien-3-one (397) (0.46%), 6β, 11α-dihydroxy-20-hydroxymethyl pregna-1, 4-dien-3-one (398) (0.60%), 11α, 15β-dihydroxy-20-hydroxymethylpregna-1, 4-dien-3-one (399) (2.0%), 11α, 17α-dihydroxy-20-hydroxymethylpregna-1, 4-dien-3-one (400) (0.40%), 14α, 15β, 17α-trihydroxy-20-hydroxymethylpregna-1, 4-dien-3-one (401) (0.40%), along with the known metabolite, 11α-hydroxy-20-hydroxymethylpregna-1, 4-dien-3-one (402) (8.66%), were synthesized through the biotransformation of 20-hydroxymethylpregna-1, 4-dien-3-one (395) with Cunninghamella elegans[90] [Figure 74]. Three new derivatives, 15β-hydroxy-20-hydroxymethylpregna-1, 4-dien-3-one (403) (1.12%), 7β-hydroxy-20-hydroxymethylpregna-1, 4-dien-3-one (404) (0.62%), and 7β, 15β-dihydroxy-20-hydroxymethylpregna-1, 4-dien-3-one (405) (8.70%), were also synthesized by Macrophomina phaseolina-catalyzed transformation of compound 395[90] [Figure 75].
Figure 74. Biotransformation of 20-hydroxymethylpregna-1,4-dien-3-one (395) with Cunninghamella elegans.
Biotransformation ganaxolone (407)
Ganaxolone (407) is a synthetic steroidal-based anti-epileptic drug (CCD-1042) under development by Marinus Pharmaceuticals. Compound 407 is a 3β-methylated derivative of allopregnanolone (neurosteroid). The new metabolite, 14α-hydroxy-5α-pregnan-1-ene-3, 20-dione (408) (1.30%), along with the four known metabolites, 11α-hydroxy-pregn-1, 4-diene-3,20-dione (409) (2.87%), 6β, 11α-dihydroxy-pregn-4-ene-3, 20-dione (410) (4.66%), 5α-pregnan-3, 20-dione (411) (4.56%), and 5α-pregnan-4-ene-3, 20-dione (412) (5.46%)[60] [Figure 76]. Ganaxolone (407) showed good aromatase inhibitory activity (IC50 = 13.76 ± 2.00 µM), while its derivatives were found to be inactive.
BIOTRANSFORMATION OF STEROIDAL ALKALOID
Biotransformation of dictyophlebine (413)
The new compound, 3β-(propyloxycarbonylamino)-dictyophlebin-16-ene (414) (0.55%), along with the two known derivatives, 5, 6-dihydrosarconidine (415) (0.52%) and iso-N-formylchonemorphine (416) (0.54%), were synthesized through the biotransformation of the steroidal alkaloid, dictyophlebine (413)[91] [Figure 77]. The new derivative 414 was found to be the potent inhibitor of acetyl-, and butyrylcholinesterase enzymes with the IC50 values of 2.2 and 1.2 μM, respectively, as compared to the standard drug, galanthamine (IC50 0.5 and 8.2 μM for AChE and BChE, respectively).
BIOLOGICAL ACTIVITY EVALUATION OF TRANSFORMED PRODUCTS
Fully characterized derivatives were evaluated through various cell-based and biochemical assays. Bio-catalytic structural changes in natural/synthetic compounds of various classes have affected their biological activities.
Hydroxylation in derivatives, 13-hydroxyisolongi-folen-4-one (37) (IC50 = 9.64 ± 0.0008 μM) and 9-hydroxyisolongifolen-4-one (40) (IC50 = 6.68 ± 0.0096 μM), has increased their activities against tyrosinase, as compared to substrate, (+)-isolongifolen-4-one (35) (IC50 = 51.91 ± 0.0245 μM). Similarly, hydroxylation in derivatives, 17α-ethynyl-11α, 17β-dihydroxyandrost-4-en-3-one (278) (IC50 = 5.95 ± 0.00078 μM), 17α-ethyl-11α, 17β-dihydroxyandrost-4-en-3-one (284) (IC50 = 3.46 ± 0.01046 μM), and 17α-ethyl-6α, 17β-dihydroxy-5α-androstan-3-one (285) (IC50 = 1.72 ± 0.00089 μM) also increased their tyrosinase inhibitory activities, as compared to substrate, ethisterone (277) (IC50 = 2.61 ± 0.037328 μM).
Structural changes in metabolites, 4β-methoxycaryophyllene-5α, 14-diol (46) (IC50 = 3.09 ± 2.61 μg/mL), 4β-methoxycaryophyllene-5α, 15-diol (47) (IC50 = 0.72 ± 0.17 μg/mL), and caryophyllene-5α,15-diol (48) (IC50 = 1.35 ± 0.43 μg/mL), have significantly increased their anti-malarial activity in vitro, in comparison to the standard drug, chloroquine diphosphate (IC50 = 0.025 ± 0.01 μg/mL). Derivatives, 15-hydroxycaryophyllene oxide (52) (IC50 = 44.0 ± 0.2 μM), 4β, 5α-dihydroxycaryophyll-8(13)-ene (53) (IC50 = 455.8 ± 0.1 μM), clovane-5, 9-diol (54) (IC50 = 189.5 ± 0.2 μM), 4, 5-epoxycaryophyllan-8(13)-en-14-ol (55) (IC50 = 10.9 ± 0.2 μM), 4, 5-epoxy-13-norcaryophyllan-8-one (56) (IC50 = 458.7 ± 0.5 μM), caryolane-5, 8, 13-triol (57) (IC50 = 23.6 ± 0.1 μM), clovane-5, 9, 12-triol (58) (IC50 = 43.6 ± 0.3 μM), and 4, 5-epoxycaryophyllan-3, 13-diol (59) (IC50 = 154.6 ± 0.3 μM), showed a moderate to significant inhibitory potential against butyrylcholinesterase enzyme, as compared to their substrate, (–)-caryophyllene oxide (49) (IC50 = 208.4 ± 0.8 μM).
Structural transformations in derivatives, 3-ketosclareolide (86) (100%), 3β-hydroxysclareolide (88) (87.5%), 1β, 3β-dihydroxysclareolide (89) (72.3%), 2α-hydroxysclareolide (90) (82.7%), and 1α, 3β-dihydroxysclareolide (92) (75%), have increased their phytotoxicity against Lemna minor L., in comparison to sclareolide (84) at 100 μg/mL. Compounds, dehydroabietic acid (110) (IC50 = 11 ± 01 μM), 1β-hydroxydehydroabietic acid (111), (IC50 = 130 ± 15 μM), 15-hydroxy dehydroabietic acid (112) (IC50 = 99 ± 43 μM), and 16-hydroxy dehydroabietic acid (113) (IC50 = 81 ± 90 μM), showed potent α-glucosidase inhibitory activity. In addition, Compounds, oleanolic acid (117) (IC50 = 12.8 ± 0.00 μM), 2α, 3β, 11β-trihydroxyolean-12-en-28-oic acid (118) (IC50 = 444.0 ± 8.0 μM), and 2α, 3β-dihydroxyolean-12-en-28-oic acid (119) (IC50 = 666.0 ± 20.0 μM), showed significant α-glucosidase inhibitory activity, as compared to the standard drug, acarbose (IC50 = 780.0 ± 0.28 μM). Compounds, 18-glycyrrhetinic acid (120) (IC50 = 225.1 ± 0.5 μM), 3,7-dihydroxy-11-oxo-olean-12-en-30-oic acid (121) (IC50 ≥ 300 μM), and 3, 11-dioxo-olean-12-en-30-oic acid (122) (IC50 = 144.2 ± 0.2 μM), exhibited a good lipoxygenase (LOX) inhibitory activity, as compared to the standard drug, baicalein (IC50 = 22.4 ± 0.5 μM).
Compounds, DHEA (143) (IC50 = 77.9 ± 1.95 μM), 3β,7α-dihydroandrost-5-ene-17-one (150) (IC50 = 373.5 ± 9.57 μM), 11β-hydroxyandrost-4, 6-diene-3, 17-dione (152) (IC50 = 430 ± 7.13 μM), 5α-androstane-3, 17-dione (154)(IC50 = 221.6 ± 12.5 μM), and androst-4-ene-3, 17-dione (155) (IC50 = 191.4 ± 1.17 μM), showed significant activity against β-glucuronidase enzymes. Compounds 185 (IC50 = 190.3 ± 1.18 μM) and 187 (IC50 = 482.66 ± 6.86 μM) showed weak inhibition of β-glucuronidase. Derivative, 15α, 17β-dihydroxy-17α-methylandrost-1, 4-dien-3-one (247) showed a remarkable β-glucuronidase inhibitory activity (IC50 = 60.7 μM). Interestingly, derivative, 6β-hydroxy-etonogestrel (308), was found to be significantly active against β-glucuronidase enzyme with the IC50 value of 13.97 ± 0.12 μM, compared to the standard inhibitor, D-saccharic acid 1, 4 lactone (IC50 = 48.4 ± 1.25 μM).
Nandrolone (175) (IC50 = 32.0 ± 0.5 μM), and its derivatives, 10β, 12β, 17β-trihydroxy-19-nor-4-androsten-3-one (178) (IC50 ≥ 100 μM), 10β, 16α, 17β-trihydroxy-19-nor-4-androsten-3-one (179) (IC50 = 77.39 ± 5.52 μM), 6β, 10β, 17β-trihydroxy-19-nor-4-androsten-3-one (180) (IC50 = 70.90 ± 1.16 μM), 10β, 17β-dihydroxy-19-nor-4-androsten-3-one (181) (IC50 = 54.94 ± 1.01 μM), 6β, 17β-dihydroxy-19-nor-4-androsten-3-one (182) (IC50 = 80.23 ± 3.39 μM), 10β-hydroxy-19-nor-4-androsten-3, 17-dione (183) (IC50 = 61.12 ± 1.39 μM), and 16β, 17β-dihydroxy-19-nor-4-androsten-3-one (184) (IC50 = 29.55 ± 1.14 μM) exhibited significant anti-leishmanial activity against Leishmania major in vitro.
Compounds, metenolone acetate (220) (62.5% ± 4.4 %), 6α-hydroxy-1-methyl-3-oxo-5α-androst-1-en-17-yl acetate (221) (73.4% ± 0.6 %), 15β, 20-dihydroxy-1-methyl-3-oxo-5α-androst-1-en-17-yl acetate (224) (81.0% ± 2.5 %), 17β-hydroxy-1-methyl-3-oxo-5α-androst-1-en (227) (69.7% ± 1.4 %), 1-methyl-5β-androst-1-en-3, 17-dione (229) (73.2% ± 0.3%), 17β-hydroxy-1α-methyl-5α-androstan-3-one (232) (60.1% ± 3.3 %), and 17β, 15α-dihydroxy-1α-methyl-5α-androstan-3-one (233) (71.0% ± 7.2 %), showed good inhibition of cytokine (TNF-α) production. Melengestrol acetate (352) (IC50 = 2.77 ± 0.08 µM), and its derivatives, 17α-acetoxy-11α-hydroxy-6-methyl-16-methylenepregna-4,6-diene-3,20-dione (353) (IC50 = 2.78 ± 0.07 µM), 17α-acetoxy-6, 7α-epoxy-6β-methyl-16-methylenepregna-4, 6-diene-3,20-dione (355) (IC50 = 2.74± 0.1 µM), and 17α-acetoxy-11β, 15β-dihydroxy-6-methyl-16-methylenepregna-4, 6-diene-3, 20-dione (356) (IC50 ≤ 2 µM), showed potent T- cell proliferation inhibitory activities. Similarly, medrysone (357) (IC50 = 2.0 ± 0.04 μg/mL), and its derivatives, 14α-hydroxy-6α-methylpregn-4-ene-3, 11, 20-trione (358) (IC50 = 20.0 ± 0.9 μg/mL), 6β-hydroxy-6α-methylpregn-4-ene-3, 11, 20-trione (359) (IC50 = 14.6 ± 2.1 μg/mL), 15β-hydroxy-6α-methylpregn-4-ene-3, 11, 20-trione (360) (IC50 = 9.2 ± 0.7 μg/mL), 6β, 17α-dihydroxy-6α-methylpregn-4-ene-3, 11, 20-trione (361) (IC50 = 1.2 ± 0.02 μg/mL), 6β, 20(S)-dihydroxy-6α-methylpregn-4-ene-3, 11-dione (362) (IC50 = 15.2 ± 2.4 μg/mL), 11β, 16β-dihydroxy-6α-methylpregn-4-ene-3-one (363) (IC50 ≤ 0.2 μg/mL), and 15β, 20(R)-dihydroxy-6α-methylpregn-4-ene-3, 11-dione (364) (IC50 = 10.4 ± 0.42 μg/mL), also showed potent activities against T-cell proliferation in vitro, as compared to the standard drug, prednisolone (IC50 ≤ 3.1 μg/mL).
Biotransformed products, 17β-hydroxy-1-methyl-5α-androst-1-ene-3, 16-dione (236), and 15β, 17β -dihydroxy-1-methyl-5α-androstan-1-ene-3-one (237), also showed potent activity against isolated polymorphonuclear leukocytes (PMNs) with the IC50 values of 14.0 ± 1.7, and 4.70 ± 0.5 μg/mL, respectively.
Derivative, 14β, 17β-dihydroxy-2-(hydroxymethyl)-17α-pregn-4-en-20-yn-3-one (241) showed potent cytotoxicity against HeLa cancer cell line with the IC50 = 0.283 ± 0.013 μM, as compared to the standard drug, doxorubicin (IC50 = 0.506 ± 0.015 μM). Metabolite, 11α-hydroxy-6-methylene-androsta-1, 4-diene-3, 17-dione (319) showed moderate cytotoxicity against PC-3 (IC50= 16.83 ± 0.96 μM) and cancer HeLa (IC50= 24.87 ± 0.72 μM). Metabolite 2α-methyl-5α-androsta-17β-hydroxy-3-one (342) (IC50 = 19.6 ± 1.4 µM) exhibited potent activity against HeLa cells, in contrast to drostanolone enanthate (334) (IC50 = 54.7 ± 1.6 µM), and standard cisplatin (IC50 = 40.1 ± 2.0 µM). Derivatives, 2α-methyl-3α, 14α, 17β-trihydroxy-5α-androstane (335) (IC50 = 64.3 ± 3.0 µM), 2-methylandrosta-11α-hydroxy-1, 4-diene-3, 17-dione (336) (IC50 = 40.7 ± 0.9 µM), 2-methylandrosta-14α-hydroxy-1, 4-diene-3, 17-dione (337) (IC50 = 40.7 ± 0.9 µM), 2α-methyl-3α,17β-dihydroxy-5α-androstane (338) (IC50 = 49.5 ± 2.2 µM), 2-methylandrosta-1, 4-diene-3, 17-dione (339) (IC50 = 39.8 ± 1.5 µM), 2-methyl-5α-androsta-7α-hydroxy-1-ene-3, 17-dione (341) (IC50 = 58.0 ± 1.0 µM), and 2α-methyl-5α-androsta-17β-hydroxy-3-one (342) (IC50 = 30.1 ± 1.0 µM) also displayed a remarkable activity against HeLa cell line. Metabolites 335 (IC50 = 58.4 ± 1.6 µM), 336 (IC50 = 59.1 ± 2.6 µM), 337 (IC50 = 60.4 ± 0.9 µM), 338 (IC50 = 51.8 ± 3.4 µM), 339 (IC50 = 68.1 ± 1.2 µM), and 340 (IC50 = 39.1 ± 2.0 µM) showed significant anti-cancer activity against PC-3 cells, compared to compounds 342 (IC50 = 96.2 ± 3.0 µM), 335 (IC50 = 84.6 ± 6.4 µM), 339 (IC50 = 84.0 ± 3.1 µM), and standard cisplatin (IC50 = 76.5 ± 1.2 µM). Compounds 334 (IC50 = 5.0 ± 1.2 µM), 338 (IC50 = 12.4 ± 2.3 µM), 340 (IC50 = 16.7 ± 2.6 µM), and 90 (IC50 = 14.7 ± 2.6 µM) showed potent activity against H460 cells, as compared to cisplatin (IC50 = 22.2 ± 2.1 µM). Compounds 335 (IC50 = 44.4 ± 2.0 µM), 336 (IC50 = 33.2 ± 1.0 µM), 337 (IC50 = 38.5 ± 2.8 µM), 339 (IC50 = 31.9 ± 1.8 µM), and 340 (IC50 = 26.4 ± 0.9 µM) also presented good anti-cancer activity against H460 cells. Compound 334 (IC50 = 3.1 ± 3.2 µM) showed potent anti-cancer activity against HCT116 cells, in contrast to standard cisplatin (IC50 = 11.2 ± 3.0 µM).
Physalin H (373) (IC50 = 6.03 ± 0.005 μM), and its structural analogues, 6, 7-dehydrophysalin H (374) (IC50 = 7.74 ± 0.015 μM), 6-deoxyphysalin H (375) (IC50 = 6.34 ± 0.03 μM), and isophysalin B (376) (IC50 = 13.8 ± 0.05 μM), showed potent anti-leishmanial activity, compared to the standard drug, amphotericin B (IC50 = 0.129 ± 0.105 μM), against promastigotes of Leishmania major (DESTO).
CONCLUSION AND FUTURE PERSPECTIVES
The scope of present biotransformation studies conducted in our laboratories was to synthesize new analogues of monoterpenes, sesquiterpenes, diterpenes, sesterterpenes, triterpenes, and steroidal-based anabolic, contraceptive, anti-cancer, and anti-epileptic drugs by using the cost-effective, and eco-friendly bio-catalytic approach. In the present review, over 350 new and known metabolites are presented through biotransformation of natural/synthetic/semisynthetic compounds. Aromatization, hydroxylation, epoxidation, hydrogenation, and dehydrogenation were the main reactions that occurred during the whole-cell bio-catalyzed transformation reactions. The technique of biotransformation was found to be a robust method to produce compounds having structural similarities with their parent molecules. Newly synthesized derivatives were evaluated for various biological activities. Variations in the structures of the transformed products often led to changes in their biological activities in comparison to their parent drugs.
Several bio-transformed products exhibit biological activities and have been explored for different applications, mainly in the food and pharmaceutical industries. These structurally altered compounds are extensively being studied for their effects on human health. In the search for biologically active compounds, derivatization of natural products and existing drugs has emerged as an important research area. In the future, further biotransformation studies will include the production of biologically important transformed products in bulk quantities for their in vitro, ex vivo, and in vivo studies. After successful evaluation, these compounds may serve as new therapeutic agents against various ailments. The mode of actions of potent derivatives through NMR spectroscopy and molecular docking studies will also be performed. Moreover, the biologically important metabolites need to be studied at enzymatic levels using modern molecular and structural biology techniques in order to understand the Supplementary Figures involvedin the biotransformation reactions.
DECLARATIONS
Acknowledgments
We would like to acknowledge present and past students and research officers of the biotransformation laboratories whose names are cited in the references. Financial support from national and international funding agencies is also appreciated here.
Authors’ contributions
Designed the topic and provided ideas: Choudhary M, Atia-tul-Wahab
Collected the data and drafted the review: Siddiqui M, Atia-tul-Wahab
Checked and finalized this review article: Choudhary M, Atia-tul-Wahab
Availability of data and materials
Not applicable.
Financial support and sponsorship
Not applicable.
Conflicts of interest
All authors declared that there are no conflicts of interest.
Ethical approval and consent to participate
Not applicable.
Consent for publication
Not applicable.
Copyright
© The Author(s) 2023.
Supplementary Materials
REFERENCES
1. Feng J, Wu Q, Zhu D, Ma Y. Biotransformation enables innovations toward green synthesis of steroidal pharmaceuticals. ChemSusChem 2022;15:e202102399.
2. Choudhary M, Gupta S, Dhar MK, Kaul S. Endophytic fungi-mediated biocatalysis and biotransformations paving the way toward green chemistry. Front Bioeng Biotechnol 2021;9:664705.
3. Salter R, Beshore DC, Colletti SL, et al. Microbial biotransformation - an important tool for the study of drug metabolism. Xenobiotica 2019;49:877-86.
4. Bianchini LF, Arruda MF, Vieira SR, et al. Microbial biotransformation to obtain new antifungals. Front Microbiol 2015;6:1433.
5. Birolli WG, Ferreira IM, Alvarenga N, et al. Biocatalysis and biotransformation in Brazil: an overview. Biotechnol Adv 2015;33:481-510.
6. Banerjee S, Singh S, Ur Rahman L. Biotransformation studies using hairy root cultures - a review. Biotechnol Adv 2012;30:461-8.
7. Gkantzou E, Chatzikonstantinou AV, Fotiadou R, et al. Trends in the development of innovative nanobiocatalysts and their application in biocatalytic transformations. Biotechnol Adv 2021;51:107738.
8. Pervaiz I, Ahmad S, Madni MA, Ahmad H, Khaliq FH. Microbial biotransformation: a tool for drug designing. Appl Biochem Microbiol 2013;49:437-50.
9. Borges WDS, Borges KB, Bonato PS, Said S, Pupo MT. Endophytic fungi: natural products, enzymes and biotransformation reactions. Curr Org Chem 2009;13:1137-1163.
10. Buttgereit F, Bijlsma JWJ, Strehl C. Will we ever have better glucocorticoids? Clin Immunol 2018;186:64-6.
11. Hegazy ME, Mohamed TA, ElShamy AI, et al. Microbial biotransformation as a tool for drug development based on natural products from mevalonic acid pathway: a review. J Adv Res 2015;6:17-33.
12. Basso A, Serban S. Industrial applications of immobilized enzymes-a review. Mol Catal 2019;479:110607.
14. Choi JM, Han SS, Kim HS. Industrial applications of enzyme biocatalysis: current status and future aspects. Biotechnol Adv 2015;33:1443-54.
15. Carvalho CC. Enzymatic and whole cell catalysis: finding new strategies for old processes. Biotechnol Adv 2011;29:75-83.
16. Otles S, Özyurt VH. Biotransformation in the production of secondary metabolites. Bioactive Natural Products. Elsevier; 2021. pp. 435-57.
17. Kebamo S, Tesema S, Geleta B. The role of biotransformation in drug discovery and development. J Drug Metab Toxicol 2015;6:1000196.
18. Liu JH, Yu BY. Biotransformation of bioactive natural products for pharmaceutical lead compounds. COC 2010;14:1400-06.
19. Pratush A, Ye X, Yang Q, et al. Biotransformation strategies for steroid estrogen and androgen pollution. Appl Microbiol Biotechnol 2020;104:2385-409.
20. Parales RE, Bruce NC, Schmid A, Wackett LP. Biodegradation, biotransformation, and biocatalysis (b3). Appl Environ Microbiol 2002;68:4699-709.
21. Farooq A, Tahara S, Choudhary MI, et al. Biotransformation of (-)-a-pinene by botrytis cinerea. Z Naturforsch C J Biosci 2002;57:303-6.
22. Farooq A, Choudhary MI, Tahara S, et al. The microbial oxidation of (-)-beta-pinene by botrytis cinerea. Z Naturforsch C J Biosci 2002;57:686-90.
23. Farooq A, Choudhary MI, Atta-ur-Rahman, et al. Detoxification of terpinolene by plant pathogenic fungus botrytis cinerea. Z Naturforsch C J Biosci 2002;57:863-6.
24. Atta-ur-Rahman Au, Yaqoob M, Farooq A, et al. Fungal transformation of (1R,2S,5R)-(-)-menthol by cephalosporiumaphidicola. J Nat Prod 1998;61:1340-2.
25. Musharraf SG, Ahmed MA, Ali RA, Choudhary MI. Hydroxylation of (+)-menthol by Macrophomina phaseolina. Biocatal Biotransfor 2011;29:77-82.
26. Mohammad MY, Shakya A, Al-Bakain R, Haroon MH, Choudhary MI. New monoterpenoid by biotransformation of thymoquinone using Aspergillus niger. Bioorg Chem 2018;80:212-5.
27. Choudhary M, Musharraf S, Khan M, et al. Microbial Transformation of Isolongifolen-4-one. HCA 2003;86:3450-60.
28. Choudhary MI, Musharraf SG, Nawaz SA, et al. Microbial transformation of (-)-isolongifolol and butyrylcholinesterase inhibitory activity of transformed products. Bioorg Med Chem 2005;13:1939-44.
29. Musharraf SG, Najeeb A, Khan S, et al. Microbial transformation of 5α-hydroxycaryophylla-4(12), 8(13)-diene with Macrophomina phaseolina. J Mol Catal B-Enzym 2010;66:156-60.
30. Choudhary MI, Siddiqui ZA, Khan S, et al. Biotransformation of (–)-caryophyllene oxide by cell suspension culture of catharanthus roseus. Zeitschrift für Naturforschung B 2006;61:197-200.
31. Choudhary MI, Siddiqui ZA, Nawaz SA, Atta-ur-Rahman. Microbial transformation and butyrylcholinesterase inhibitory activity of (-)-caryophyllene oxide and its derivatives. J Nat Prod 2006;69:1429-34.
32. Choudhary MI, Kausar W, Siddiqui ZA, Rahman A. Microbial Metabolism Of (+)-Cycloisolongifol-5β-Ol. Zeitschrift für Naturforschung B 2006;61:1035-8.
33. Choudhary MI, Musharraf SG, Sami A, Atta-ur-Rahman. Microbial transformation of sesquiterpenes, (-)-ambrox, and (+)-sclareolide. HCA 2004;87:2685-94.
34. Nasib A, Musharraf SG, Hussain S, et al. Biotransformation of (-)-ambrox by cell suspension cultures of Actinidia deliciosa. J Nat Prod 2006;69:957-9.
35. Musharraf SG, Naz S, Najeeb A, Khan S, Choudhary MI. Biotransformation of perfumery terpenoids, (-)-ambrox® by a fungal culture Macrophomina phaseolina and a plant cell suspension culture of Peganum harmala. Chem Cent J 2012;6:82.
36. Musharraf SG, Uddin J, Akhter M, et al. Biotransformation of an antimalarial drug, artemether by plant and fungal cell cultures. J Mol Catal B-Enzym 2012;82:80-5.
38. Choudhary MI, Batool I, Atif M, Hussain S, Atta-Ur-Rahman. Microbial transformation of (-)-guaiol and antibacterial activity of its transformed products. J Nat Prod 2007;70:849-52.
39. Choudhary MI, Siddiqui ZA, Hussain S. Atta-ur-Rahman. Structure elucidation and antibacterial activity of new fungal metabolites of sclareol. Chem Biodivers 2006;3:54-61.
40. Sultan S, Atif M, Ali SAS, et al. metabolism of an anti-HIV and anti-malarial natural product andrographolide. Available from: https://innovareacademics.in/journals/index.php/ijpps/article/view/2799/9253 [Last accessed on 26 Apr 2023].
41. Choudhary MI, Atif M, Shah SAA, et al. Biotransformation of dehydroabietic acid with microbial cell cultures and α-glucosidase inhibitory activity of resulting metabolites. Available from: https://innovareacademics.in/journals/index.php/ijpps/article/view/2016 [Last accessed on 26 Apr 2023].
42. Choudhary MI, Ranjit R, Atta-Ur-Rahman, et al. Hydroxylation of the sesterterpene leucosceptrine by the fungus Rhizopus stolonifer. Phytochemistry 2006;67:439-43.
43. Choudhary MI, Batool I, Khan SN, et al. Microbial transformation of oleanolic acid by Fusarium lini and alpha-glucosidase inhibitory activity of its transformed products. Nat Prod Res 2008;22:489-94.
44. Choudhary M, Ali Siddiqui Z, Ahmed Nawaz S. Microbial transformation of 18beta-glycyrrhetinic acid by Cunninghamella elegans and Fusarium lini, and lipoxygenase inhibitory activity of transformed products. Nat Prod Res 2009;23:507-13.
45. Rahman AU, Choudhary MI, Asif F, Farooq A, Yaqoob M. Microbial transformations of testosterone. Nat Prod Lett 1998;12:255-61.
46. Al-Aboudi A, Mohammad MY, Musharraf SG, Choudhary MI, Atta-ur-Rahman. Microbial transformation of testosterone by Rhizopus stolonifer and Fusarium lini. Nat Prod Res 2008;22:1498-509.
47. Atif M, Shah SAA, Sultan S, Choudhary MI. Solid phase microbial fermentation of anabolic steroid, dihydrotestosterone with ascomycete fungus Fusarium oxysporum. Int J Pharm Pharm Sci 2015;7:104-107.
48. Choudhary MI, Asif F, Farooq A, Yaqoob M. Fungal transformations of steroids by cephalosporium aphidicola and trichothecium roseum. Nat Prod Lett 2000;14:217-24.
49. Atif M, Sultan S, Shah SAA, Choudhary MI. Solid phase microbial reactions of sex hormone, trans-androsterone with filamentous fungi. Int J Pharm Pharm Sci 2015;7:385-8.
50. Choudhary MI, Shah SA, Musharraf SG, Shaheen F, Atta-Ur-Rahman. Microbial transformation of dehydroepiandrosterone. Nat Prod Res 2003;17:215-20.
51. Azizuddin, Khan S, Ali K, Choudhary MI. Biotransformation of dehydroepiandrosterone by cell suspension culture of codiaeum variegatum. Chem Nat Compd 2014;50:669-72.
52. Choudhary MI, Zafar S, Khan NT, et al. Biotransformation of dehydroepiandrosterone with macrophomina phaseolina and β-glucuronidase inhibitory activity of transformed products. J Enzyme Inhib Med Chem 2012;27:348-55.
53. Choudhary MI, Musharraf SG, Shaheen F, Atta-Ur-Rahman. Microbial transformation of (+)-androsta-1 ,4-diene-3,17-dione by cephalosporium aphidicola. Nat Prod Lett 2002;16:377-82.
54. Musharraf SG, Atta-Ur-Rahman, Choudhary MI, Sultan S. Microbial transformation of (+)-adrenosterone. Nat Prod Lett 2002;16:345-9.
55. Choudhary MI, Khan NT, Musharraf SG, Anjum S, Atta-Ur-Rahman. Biotransformation of adrenosterone by filamentous fungus, cunninghamella elegans. Steroids 2007;72:923-9.
56. Choudhary M, Adnan S, Shah A, Anjum S, Atta-Ur-Rahman. Microbial oxidation of anabolic steroids. Nat Prod Res 2008;22:1289-96.
57. Baydoun E, Karam M, Atia-tul-Wahab, et al. Microbial transformation of nandrolone with cunninghamella echinulata and cunninghamella blakesleeana and evaluation of leishmaniacidal activity of transformed products. Steroids 2014;88:95-100.
58. Choudhary MI, Mohammad MY, Musharraf SG, Parvez M, Al-Aboudi A, Atta-ur-Rahman. New oxandrolone derivatives by biotransformation using Rhizopus stolonifer. Steroids 2009;74:1040-4.
59. Smith C, Wahab AT, Khan MS, et al. Microbial transformation of oxandrolone with macrophomina phaseolina and cunninghamella blakesleeana. Steroids 2015;102:39-45.
60. Siddiqui M, Atia-tul-wahab, Naveed Shaikh N, Baydoun E, Atta-ur-rahman, Choudhary MI. Biocatalytic transformation of steroidal drugs oxandrolone and ganaxolone, and aromatase inhibitory activity of transformed products. Phytochem Lett 2021;44:137-41.
61. Choudhary MI, Sultan S, Jalil S, et al. Atta-ur-Rahman. Microbial transformation of mesterolone. Chem Biodivers 2005;2:392-400.
62. Ahmad MS, Zafar S, Bibi M, et al. Biotransformation of androgenic steroid mesterolone with cunninghamella blakesleeana and macrophomina phaseolina. Steroids 2014;82:53-9.
63. Ahmad MS, Farooq R, Hussain N, et al. Three new analogues of androgenic drug mesterolone through biotransformation with Cunninghamella blakseleeana. J Mol Catal B-Enzym 2016;133:S395-9.
64. Siddiqui M, Ahmad MS, Wahab AT, et al. Biotransformation of a potent anabolic steroid, mibolerone, with cunninghamella blakesleeana, C. echinulata, and Macrophomina phaseolina, and biological activity evaluation of its metabolites. PLoS One 2017;12:e0171476.
65. Siddiqui M, Atia-Tul-Wahab, Jabeen A, et al. Whole-cell fungal-mediated structural transformation of anabolic drug metenolone acetate into potent anti-inflammatory metabolites. J Adv Res 2020;24:69-78.
66. Hussain Z, Dastagir N, Hussain S, et al. Aspergillus niger-mediated biotransformation of methenolone enanthate, and immunomodulatory activity of its transformed products. Steroids 2016;112:68-73.
67. Baydoun E, Atia-tul-Wahab, Mehmood H, et al. Microbial transformation of danazol with Cunninghamella blakesleeana and anti-cancer activity of danazol and its transformed products. Steroids 2016;105:121-7.
68. Khan NT, Zafar S, Noreen S, et al. Biotransformation of dianabol with the filamentous fungi and β-glucuronidase inhibitory activity of resulting metabolites. Steroids 2014;85:65-72.
69. Ahmad MS, Yousuf S, Atia-Tul-Wahab, et al. Biotransformation of anabolic compound methasterone with Macrophomina phaseolina, Cunninghamella blakesleeana, and Fusarium lini, and TNF-α inhibitory effect of transformed products. Steroids 2017;128:75-84.
70. Aamer M, Siddiqui M, Jabeen A, et al. Structural transformation of methasterone with Cunninghamella blakesleeana and Macrophomina phaseolina. RSC Adv 2022;12:9494-500.
71. Atia-Tul-Wahab, Siddiqui M, Ibrahim I, et al. Cunninghamella blakesleeana-mediated biotransformation of a contraceptive drug, desogestrel, and anti-MDR-Staphylococcus aureus activity of its metabolites. Bioorg Chem 2018;77:152-8.
72. Choudhary MI, Sultan S, Khan MT, Rahman AU. Microbial transformation of 17alpha-ethynyl- and 17alpha-ethylsteroids, and tyrosinase inhibitory activity of transformed products. Steroids 2005;70:798-802.
73. Aziz A, Bano S, Atia-Tul-Wahab, Choudhary MI. Microbial transformation of oral contraceptive ethisterone by aspergillus niger and cunninghamella blakesleeana. Steroids 2020;154:108467.
74. Choudhary MI, Musharraf SG, Siddiqui ZA, et al. Microbial transformation of mestranol by cunninghamella elegans. Chem Pharm Bull 2005;53:1011-3.
75. Zafar S, Bibi M, Yousuf S, Choudhary MI. New metabolites from fungal biotransformation of an oral contraceptive agent: methyloestrenolone. Steroids 2013;78:418-25.
76. Baydoun E, Atia-Tul-Wahab, Iqbal S, Smith C, Choudhary MI. Biotransformation of drospirenone, a contraceptive drug, with Cunninghamella elegans. Steroids 2017;126:30-4.
77. Baydoun E, Wahab AT, Shoaib N, et al. Microbial transformation of contraceptive drug etonogestrel into new metabolites with cunninghamella blakesleeana and cunninghamella echinulata. Steroids 2016;115:56-61.
78. Zafar S, Yousuf S, Kayani HA, et al. Biotransformation of oral contraceptive ethynodiol diacetate with microbial and plant cell cultures. Chem Cent J 2012;6:109.
79. Baydoun E, Bibi M, Iqbal MA, et al. Microbial transformation of anti-cancer steroid exemestane and cytotoxicity of its metabolites against cancer cell lines. Chem Cent J 2013;7:57.
80. Baydoun S, Atia-tul-Wahab, Bano S, Imad R, Choudhary MI. Microbial-catalysed derivatization of anti-cancer drug exemestane and cytotoxicity of resulting metabolites against human breast adenocarcinoma cell line (MCF-7)
81. Baydoun S, Wahab AT, Bano S, Imad R, Choudhary MI. Microbial-catalysed derivatization of anti-cancer drug exemestane and cytotoxicity of resulting metabolites against human breast adenocarcinoma cell line (MCF-7) in vitro. Steroids 2016;115:67-74.
82. Hussain Z, Atia-Tul-Wahab, Hussain N, et al. Seven new metabolites of drostanolone heptanoate by using Beauveria bassiana, and Macrophomina phaseolina cell suspension cultures. RSC Adv 2019;10:451-60.
83. Javed S, Atia-Tul-Wahab, Jabeen A, et al. Fungal mediated biotransformation of melengestrol acetate, and T-cell proliferation inhibitory activity of biotransformed compounds. Bioorg Chem 2020;104:104313.
84. Bano S, Wahab AT, Yousuf S, et al. New anti-inflammatory metabolites by microbial transformation of medrysone. PLoS One 2016;11:e0153951.
85. Choudhary MI, Shaheen F, Ashraf M, Jahan S. Microbial transformations of hypolipemic E-guggulsterone. J Nat Prod 1998;61:428-31.
86. Choudhary MI, Shah SA, Sami A, Ajaz A, Shaheen F. Atta-ur-Rahman. Fungal metabolites of (E)-guggulsterone and their antibacterial and radical-scavenging activities. Chem Biodivers 2005;2:516-24.
87. Choudhary MI, Yousuf S, Samreen, et al. Biotransformation of physalin H and leishmanicidal activity of its transformed products. Chem Pharm Bull (Tokyo) 2006;54:927-30.
88. Choudhary MI, Batool I, Shah SA, Nawaz SA, Atta-ur-Rahman. Microbial hydroxylation of pregnenolone derivatives. Chem Pharm Bull 2005;53:1455-9.
89. Ahmad MS, Zafar S, Yousuf S, et al. Biotransformation of 6-dehydroprogesterone with Aspergillus niger and Gibberella fujikuroi. Steroids 2016;112:62-7.
90. Choudhary MI, Erum S, Atif M, et al. Biotransformation of (20S)-20-hydroxymethylpregna-1,4-dien-3-one by four filamentous fungi. Steroids 2011;76:1288-96.
Cite This Article

How to Cite
Download Citation
Export Citation File:
Type of Import
Tips on Downloading Citation
Citation Manager File Format
Type of Import
Direct Import: When the Direct Import option is selected (the default state), a dialogue box will give you the option to Save or Open the downloaded citation data. Choosing Open will either launch your citation manager or give you a choice of applications with which to use the metadata. The Save option saves the file locally for later use.
Indirect Import: When the Indirect Import option is selected, the metadata is displayed and may be copied and pasted as needed.
About This Article
Special Issue
Copyright
Author Biographies
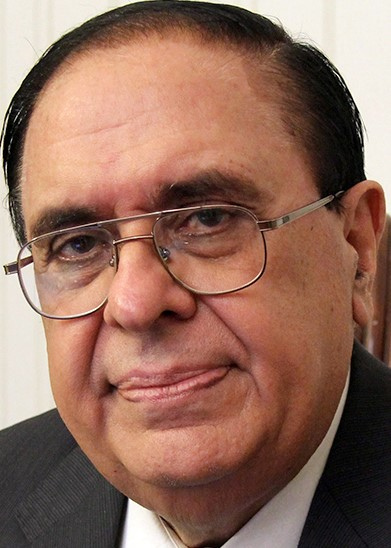
Prof. Atta-ur-Rahman, (Citations: 33226, h index 73, Google Scholar), Ph.D. in organic chemistry from Kings College, University of Cambridge (1968), has 1315 international publications in several fields of organic chemistry. He has won four civil awards including Tamgha-i-Imtiaz (1983), Sitara-i-Imtiaz (1991), Hilal-i-Imtiaz (1998), and the highest national civil award Nishan-i-Imtiaz (2002).
He was elected as a Fellow of the prestigious Royal Society (London) (2006) and an Honorary Life Fellow of Kings College, Cambridge University, UK (2007). Prof. Rahman won the UNESCO Science Prize (1999). The Austrian government has conferred its high civil award (“Grosse Goldene Ehrenzeischen am Bande") (2007). He has been conferred honorary doctorate degrees by several top universities including Cambridge University (UK) (1987).
Prof. Atta-ur-Rahman was conferred the International Science & Technology Cooperation Award (2020). He is Academician Chinese Academy of Sciences and Fellow Korean Academy of Science&Technology. Research Centers have been established in China and Malaysia-(“Academician Professor Atta-ur-Rahman One Belt and One Road TCM Research Center” in Hunan, China and “Atta-ur-Rahman Institute on Natural Product Discovery (AuRins)” in UiTM, Malaysia.
He was Federal Minister of Science (2000-2002), Education (2002), Chairman Higher Education Commission (2002-2008) and Coordinator General COMSTECH (1996-2012).
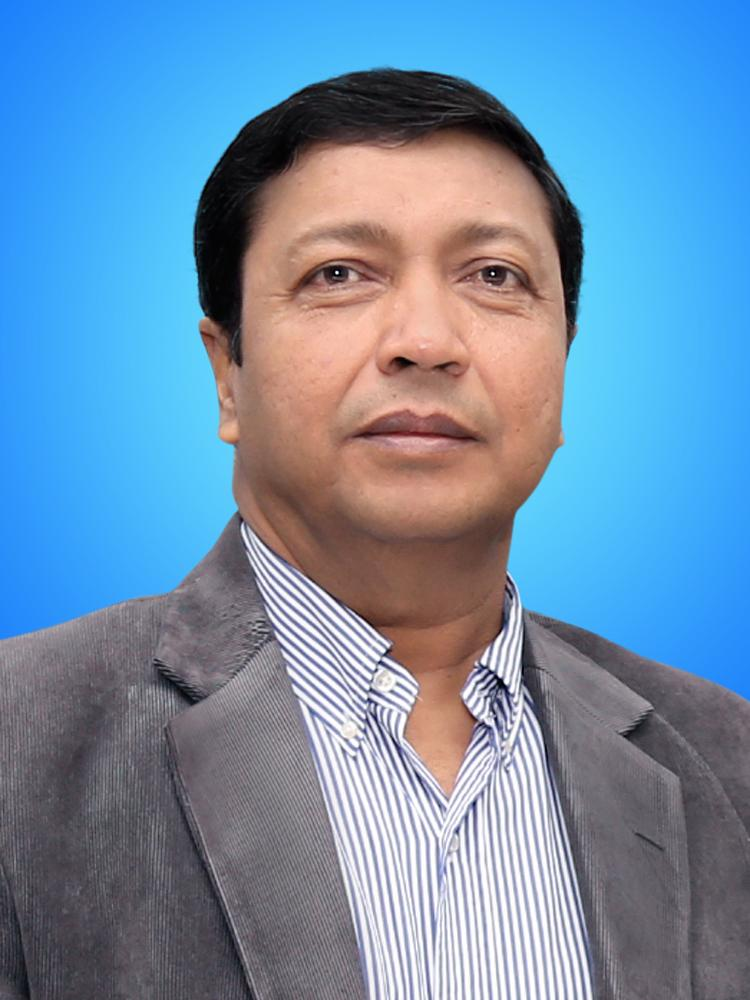
M. Iqbal Choudhary (citations over 38,000, h index 80, i10 index 874) is internationally renowned as an inspiring researcher, in organic, and bio-organic chemistry. Prof. Choudhary has 1,272 publications, 48 US patents, 94 books and 40 chapters in books. On the basis of his researches, over 100 students have been awarded Ph.D. degrees in various areas of natural product and bioorganic chemistry. He is currently serving as the Coordinator General COMSTECH (the Ministerial Standing Committee on Scientific and Technological Cooperation of the OIC (Organization of Islamic Cooperation)), and the Director International Center for Chemical and Biological Sciences, University of Karachi, Pakistan. His careers ranges from fundamental researcher to fervent leader who has built large teams of scientists, build large centers of excellence, trained human resources in developing countries, and work tirelessly for international collaboration in S&T.
He is recipient of Mustafa (pbuh) Prize, International Friendship of Peoples Republic of China, Golden Silkball Friendship Award (2020), UNESCO Chair holder (2020), Gold Medal by International Turkic Academy (2022), Distinguished Scientist Award Chinese Academy of Sciences (2020), highest National Civil Awards (2008, 2002, 1999), First Khwarizmi International Award and Prize (2006), and Economic Cooperation Organization Excellence Awards in Education (2006).
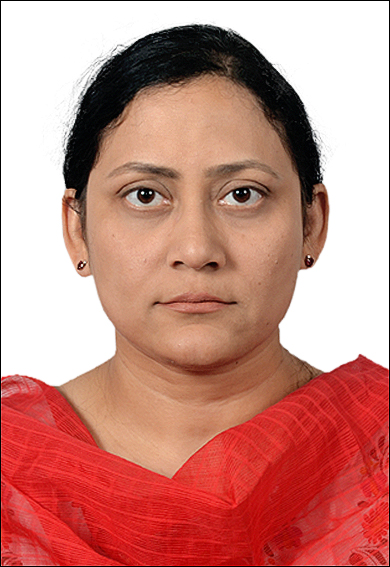
Prof. Atia-tul-Wahab Ph.D. in Organic Chemistry (H. E. J. Research Institute of Chemistry, Karachi University (2007). Has 275 impact factor, 17 US patents (granted / applied) and 03 books (co-author and edited). Post-Doctoral (Structural Biology) Germany (2014 and 2016). Post-Doctoral (Structural Biology) USA (2009 and 2010). HEC Merit Scholarship (2003-2007). Graduate Student Research Studies at University of California Irvine, USA (2006), Graduate Research Studies at Facultés Universitaires Notre-Dame de la Paix (FUNDP), Belgium (2004-2005). Pak-US Ph.D. Partial Support Program by USDOS (2005-2006). Protein deposited in Protein Data Bank (2L25, 2L1S, 2KYZ, 5GQS, 5X1X). Professor of Molecular and Structural Biology (2021-Present), Founder of first Structural Biology Laboratory of Pakistan, In charge NMR facility (ICCBS), Microbial Bank (ICCBS), Cell Culture Facility (ICCBS), National Institute of Virology (ICCBS) and Instrument access program (HEC). She is a recipient of number of international and national research grants.
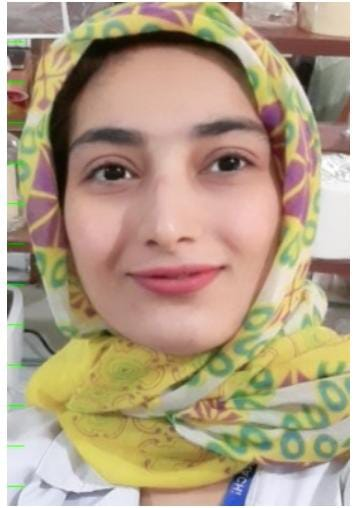
Mahwish Siddiqui received her Ph.D. degree from the International Center of Chemical and Biological Sciences, University of Karachi, Pakistan in 2021. She is currently working as a research associate at the same university. She is result-oriented research scholar for over 9 years' experience in the field of organic chemistry.
Comments
Comments must be written in English. Spam, offensive content, impersonation, and private information will not be permitted. If any comment is reported and identified as inappropriate content by OAE staff, the comment will be removed without notice. If you have any queries or need any help, please contact us at [email protected].