Trapezohedral platinum nanocrystals with high-index facets for high-performance hydrazine electrooxidation
Abstract
Direct hydrazine fuel cell is a promising portable energy conversion device due to its high energy density and free of carbon emissions. To realize the practical applications, the design of highly efficient electrocatalysts for hydrazine oxidation reaction (HzOR) is crucial. Metal nanocrystals with high-index facets have abundant step sites with reactivity. In this study, we prepared trapezohedral Pt nanocrystals (TPH Pt NCs) enclosed by {311} high-index facets and investigated the catalytic performance for hydrazine oxidation. TPH Pt NCs possess a specific activity of 39.1 mA·cm-2 at 0.20 V, much higher than {111}-faceted octahedral (13.9 mA·cm-2) and {100}-faceted cubic Pt NCs (9.11 mA·cm-2). Meanwhile, TPH Pt NCs also show superior stability. Density functional theory (DFT) calculation indicates that Pt(311) facilitates the deprotonation of N2H4* to N2H3* (the rate-determining step) and improves the HzOR activity. This study is helpful for the design of advanced electrocatalysts for HzOR, especially high-index faceted Pt nanocatalysts.
Keywords
INTRODUCTION
Hydrazine (N2H4) is one of the most promising substitutes for hydrogen in fuel cells[1-3]. In comparison with other candidates (e.g., methanol, ethanol, and formic acid) applied in direct fuel cells, hydrazine is a carbon-free fuel with high energy density (5.5 kW·h·L-1). Therefore, direct hydrazine fuel cells (DHFCs) have been recognized as one of the promising energy conversion devices due to their high theoretical potential of
Despite the great progress in the recent developments on DHFCs, the actual open circuit voltage is still significantly lower than the theoretical one, and a large amount of energy is consumed by the overpotential of the HzOR[8]. Therefore, the design of highly active electrocatalysts is crucial for the practical applications of DHFCs. Current studies of HzOR mainly focus on the design and fabrication of efficient electrocatalysts such as noble metal and intermetallic compounds[9-13], non-noble metals and their single atoms[14-17], and non-metallic materials[18]. The catalytic activity is closely related to the surface and electronic structure of the catalysts[19-25]. However, the effect of surface structure of metal nanocrystals on HzOR is rarely studied, especially that of high-index facets. Rosca and Koper[26,27] reported that the HzOR on bulk Pt single-crystal electrode is structure-sensitive, and the electrocatalytic activity increases in the order of
Herein, we carried out the HzOR on high-index faceted Pt NCs for the first time. We prepared trapezohedral Pt nanocrystals (TPH Pt NCs) enclosed with {311} high-index facets, octahedral Pt NCs with {111} facets and cubic Pt NCs with {100} facets by electrochemical method. It was found that TPH Pt NCs show much higher catalytic activity and stability of HzOR than octahedral and cubic Pt NCs. The activity of TPH Pt NCs just decreased by only 28% after 5000 s test, while 57% and 48% decline are observed on octahedral and cubic Pt NCs, respectively. Density functional theory (DFT) calculation demonstrates that the rate-determining step of HzOR on the three Pt surfaces of Pt(311), Pt(111) and Pt(100) is the first-step dehydrogenation of N2H4* to N2H3*, and Pt(311) shows the lowest energy barrier, which is beneficial for the HzOR activity.
EXPERIMENTAL
Electrochemical preparation of Pt NCs was performed in a standard three-electrode cell with a saturated calomel electrode (SCE) as reference electrode and a platinum plate as counter electrode. The working electrode was a glassy carbon rod (D = 6 mm, Takai Carbon Co., Ltd., Tokyo, Japan), which was controlled by a 263A potentiostat (EG & G) with an electrochemical square-wave potential (SWP) program
Prior to each electrochemical measurement, the electrolyte solution was deoxygenated by bubbling high-purity N2 for 20 min. The electrochemical measurements of HzOR were conducted in
RESULTS AND DISCUSSION
The Pt NCs are electrodeposited on a glassy carbon electrode using a programmed square-wave potential method [Supplementary Table 1]. The SEM images of TPH Pt NCs, OTH Pt NCs, and cubic Pt NCs are shown in Figure 1. The corresponding average sizes were about 139, 110 and 91 nm, respectively. The inset provided the atomic model of the corresponding facets: TPH Pt NCs are enclosed by 24 {hkk} (h > k > 0) high-index facets, OTH and cubic Pt NCs are enclosed by {111} and {100} low-index facets, respectively. The Miller indices of TPH Pt NCs were determined by measuring the plane angle in the TEM image along the [001] orientation [Supplementary Figure 1]. The average values of angles α and β were 142.0° and 128.1°, respectively, which are near to the theoretical values of 143.1° and 126.9° for {311} facets, and thus the TPH Pt NCs are denoted as TPH Pt-{311} NCs. The {311} facet is composed of a two-atomic-width (100) terrace separated by a monatomic (111) step. The 4-fold-symmetrical SAED pattern confirms that the nanoparticle is of single crystalline. From HRTEM image of TPH Pt NC [Supplementary Figure 2], the {311} step sites could be observed directly. The formation of high-index faceted Pt NCs can be ascribed to the repetitive adsorption/desorption of oxygen species on the Pt NCs induced by the SWP[35]. However, over intensive etching at high EU of SWP can remove Pt step atoms and result in the formation of cubic or octahedral
Figure 1. SEM images of (A) trapezohedral (TPH) Pt NCs; (B) octahedral (OTH) Pt NCs; and (C) cubic Pt NCs. The inset presents high magnification SEM image, size histogram and atomic model of the facet. (D) Cyclic voltammograms of the TPH, OTH, and cubic Pt NCs recorded in 0.1 M H2SO4 at 50 mV s-1.
The surface structure of the as-prepared Pt NCs was further characterized by cyclic voltammetry (CV) in deaerated 0.1 M H2SO4 solution. As shown in Figure 1D, the obtained CV curves could be divided into three regions, including the HUPD (underpotentially deposited hydrogen) region at the potential of 0.05 ~ 0.40 V
The performance of the as-prepared catalysts for HzOR was evaluated. Figure 2A shows the CV curve of HzOR on TPH Pt-{311} NCs in an Ar-saturated 0.5 M N2H4 + 1.0 M KOH solution at room temperature. High oxidation current can be seen on TPH Pt-{311} NCs and the current increases with the increase of potential, while the bare glassy carbon electrode is almost inert to the HzOR. The onset potential of HzOR on TPH Pt-{311} NCs is about 0.1 V, close to the value on bulk Pt(110) with step atoms, much lower than that of Pt(100) and Pt(111) reported previously[27]. The hysteresis loop in the CV curve of HzOR on TPH Pt NCs might be caused by the decrease of N2H4 concentration near electrode surface in the positive scan due to the intensive HzOR, and the formation of some poisoning species. Figure 2B compares the linear sweep voltammetric (LSV) curves of the as-prepared catalysts, where the current density is normalized by the geometric area. The current density on TPH Pt-{311} NCs is 41.7 mA·cm-2geo at 0.20 V, which is much higher than 13.4 mA·cm-2geo on OTH Pt-{111} NCs and 9.7 mA·cm-2geo on cubic Pt-{100} NCs, demonstrating that the TPH Pt-{311} NCs have a high activity for HzOR. The catalysts were further tested for oxygen evolution reaction (OER) in 1.0 M KOH solution [Figure 2B]. Obviously, the TPH Pt-{311} NCs only need 0.12 V to acquire a current density of 6.0 mA·cm-2geo in HzOR, which is much lower than the potential of 1.94 V in OER. This result indicates that the substitution of OER with HzOR in the water electrolysis system can significantly improve energy conversion efficiency.
Figure 2. (A) CV curves of HzOR on TPH Pt-{311} NCs and bare glassy carbon electrode in an Ar-saturated 0.5 M N2H4 + 1.0 M KOH solution; (B) LSV curves of the catalysts for HzOR and OER; (C) Specific activity and mass activity of the catalysts at 0.20 V; (D) Tafel plots of the catalysts.
The currents of HzOR on different catalysts were also normalized to the ECSA and the estimated Pt loading of the catalysts [Supplementary Tables 2 and 3], as shown in Figure 2C. The specific activity and estimated mass activity of TPH Pt-{311} NCs are 39.1 mA·cm-2ECSA and 2.21 A·mgPt-1 at 0.20 V, respectively. The specific activity of TPH Pt-{311} NCs is 2.8-fold that of OTH Pt-{111} NCs (13.90 mA·cm-2ECSA) and 4.3-fold that of cubic Pt-{100} NCs (9.11 mA·cm-2ECSA). The high activity of TPH Pt-{311} NCs could be attributed to the high density of step sites on the surface. The Tafel slope was calculated to compare the catalytic kinetics during the HzOR [Figure 2D]. From the linear fitting of the plot of η versus log (j), the Tafel slope of TPH Pt-{311} NCs is measured to be 46 mV·dec-1, which is lower than that of OTH Pt-{111} NCs (59 mV·dec-1) and cubic Pt-{100} NCs (65 mV·dec-1), revealing that the TPH Pt-{311} NCs have a fast charge transfer kinetic.
The stability of the catalysts for HzOR was evaluated, as shown in Figure 3A. A sudden decrease in the current density at the initial stage can be observed for all the three catalysts. The current density of the TPH Pt-{311} NCs only decreased by 28% of the initial activity after 5000 s at 0.20 V; however, the OTH Pt-{111} NCs and cubic Pt-{100} NCs decreased by 57% and 48%, respectively. After the stability test, the remained specific activity of the TPH Pt-{311} NCs is 28.2 mA·cm-2ECSA, which was 4.7-fold (5.98 mA·cm-2ECSA) that of OTH Pt-{111} NCs and 5.9-fold (4.74 mA·cm-2ECSA) that of cubic Pt-{100} NCs [Figure 3B]. The estimated mass activity of the TPH Pt-{311} NCs is 1.59 A·mgPt-1, which is 2.4-fold (0.65 A·mgPt-1) that of
Figure 3. (A) Chronoamperometric test of the as-prepared catalysts at 0.20 V vs. RHE in 0.5 M N2H4 + 1 M KOH solution; (B) Specific activity and mass activity at 0.20 V of the as-prepared catalysts before and after accelerated durability tests (ADT) of 5000 s at 0.20 V; (C) EIS of TPH Pt-{311} NCs, OTH Pt-{111} NCs and cubic Pt-{100} NCs at 0.20 V (inset: equivalent circuit used for data analyses, Rs and Rct are the ohmic and charge-transfer resistance, respectively); (D) HzOR activities of TPH Pt-{311} NCs compared with the reference results shown in Supplementary Table 4.
Figure 3C shows the electrochemical impedance spectroscopy (EIS) of the as-prepared catalysts at 0.20 V. The charge-transfer resistance (Rct) reflects the kinetics of electrocatalysis on the catalysts, and a lower Rct value corresponds to a faster reaction rate. TPH Pt-{311} NCs exhibit a smaller Rct of 6.4 Ω than that of OTH Pt-{111} NCs (7.0 Ω) and cubic Pt-{100} NCs (7.2 Ω), indicating that a fast charge transport rate at the catalyst/electrolyte interface of TPH Pt-{311} NCs, which is consistent with the activity tendency observed in the LSV test for HzOR. Compared with the catalysts reported in recent studies[40-42], TPH Pt-{311} NCs exhibit an outstanding activity for HzOR, as shown in Figure 3D and Supplementary Table 4.
To understand the reaction mechanism and different catalytic activity of TPH Pt-{311} NCs, OTH Pt-{111} NCs and cubic Pt-{100} NCs, density functional theory (DFT) calculation of HzOR on Pt(311), Pt(111) and Pt(100) planes are conducted. The slab models of N2H4 adsorption on Pt(311), Pt(111) and Pt(100) are first given to reveal the charge density difference of N2H4* [Figure 4A]. Charge density difference analysis (CDDA) indicates prominent charge transfer from the N atoms in N2H4 to the nearby Pt atoms. The Bader charge analysis indicates that the electron transferred is -0.98, -1.57, and -1.82 e on Pt(311), Pt(111), and Pt(100), respectively. Less electron transfer suggests weaker interaction between N2H4* and Pt(311). The
Figure 4. (A) The structural model of N2H4 adsorption on Pt(311), Pt(111), and Pt(100) planes, and the corresponding CDDA, where the yellow and cyan regions indicate the accumulation and depletion of the charge, respectively; (B) Free energy profiles of stepwise dehydrogenation of N2H4 on different Pt planes; (C) Proposed pathway of the HzOR on Pt(311). The pink, blue and grey balls represent Pt, N and H atoms, respectively.
The elementary reactions for stepwise N2H4 dehydrogenation (N2H4 → N2H4* → N2H3* → N2H2* → N2H* → N2*) are investigated on the three Pt planes. The adsorption of N2H4 on all the three Pt planes is thermodynamically spontaneous, which makes the catalysts easily covered by the N2H4* at the initial stage. The initial dehydrogenation of N2H4* to N2H3* is endothermic on all the studied surfaces, and is the rate-determining step (RDS) of HzOR since this step holds the highest energy barrier. For Pt(311), the energy barrier of the initial dehydrogenation of N2H4* to N2H3* is 0.15 eV, which is much lower than that on Pt(111) and Pt(100) (0.29 and 0.32 eV, respectively). The lower energy barrier of RDS on Pt(311) compared with that of Pt(111) and Pt(100) verify that Pt(311) is a highly active surface for HzOR. Note that unlike
From the free energy profiles of N2H4 stepwise dehydrogenation [Figure 4B], the low RDS energy barrier of N2H4* to N2H3* mainly comes from the low adsorption energy of N2H4* on Pt(311). This is an unexpected phenomenon, because the low-coordinated Pt step atoms [coordination number (CN) = 7] on Pt(311)
Calculated Gibbs free energies of elementary steps for electrocatalytic hydrazine oxidation reaction on Pt(311), Pt(111) and Pt(100), respectively
Elementary steps | Gibbs free energies (ΔG)/eV | ||
Pt(311) | Pt(111) | Pt(100) | |
N2H4 + * → N2H4* | -0.43 | -0.58 | -0.63 |
N2H4* → N2H3* + H+ + e- | 0.15 | 0.29 | 0.32 |
N2H3* → N2H2* + H+ + e- | -1.06 | -1.08 | -1.08 |
N2H2* → N2H* + H+ + e- | -0.04 | -0.04 | -0.04 |
N2H* → N2* + H+ + e- | -0.04 | -0.04 | -0.04 |
N2* → N2 + * | 0.03 | 0.04 | 0.05 |
N2H4* → 2NH2* | 0.26 | 0.47 | 0.74 |
N2H3* → NH* + NH2* | 0.67 | 0.73 | 0.85 |
N2H2* → 2NH* | 0.34 | 0.52 | 0.59 |
The dehydrogenation process of HzOR on Pt is given [Figure 4C and Supplementary Figures 4 and 5]. The mechanism of HzOR on Pt(311) can be described as follows. First, as N2H4 is close to the surface of Pt(311), it is easily adsorbed on the step sites forming N2H4*. Then, N2H4* stepwise-dehydrogenated and finally converted to N2, and the N-H bond cleavage and the retention of N-N bonds are facilitated by the chemisorption of N2H4 through both nitrogen atoms[47].
CONCLUSIONS
In summary, we synthesized TPH Pt NCs with {311} high-index facets, OTH Pt-{111} NCs, and cubic
DECLARATIONS
Authors’ contributions
Conceived the idea of the project: Tian N, Hu SN, Lou YY
Made substantial contributions to conception and design of the study, performed data analysis and interpretation and wrote the draft of manuscript: Tian N, Zhou ZY, Hu SN, Lou YY, Li MY, Xiao C
Performed data acquisition and provided administrative, technical, and material support: Sun SG, Tian N, Zhou ZY
Discussed and revised the manuscript: Tian N, Zhou ZY, Hu SN, Lou YY
Finalized the manuscript: Sun SG, Tian N, Zhou ZY
Availability of data and materials
The data supporting this article have been included as part of the Supplementary Materials.
Financial support and sponsorship
This research was financially supported by grants from National Natural Science Foundation of China (22172135, 22002131) and China Postdoctoral Science Foundation (2020M671963).
Conflicts of interest
All authors declared that there are no conflicts of interest.
Ethical approval and consent to participate
Not applicable.
Consent for publication
Not applicable.
Copyright
© The Author(s) 2023.
Supplementary Materials
REFERENCES
1. An L, Zhao T, Li Y. Carbon-neutral sustainable energy technology: direct ethanol fuel cells. Renew Sust Energ Rev 2015;50:1462-8.
2. Rostamikia G, Janik MJ. Direct borohydride oxidation: mechanism determination and design of alloy catalysts guided by density functional theory. Energy Environ Sci 2010;3:1262.
3. Ji X, Lee KT, Holden R, et al. Nanocrystalline intermetallics on mesoporous carbon for direct formic acid fuel cell anodes. Nat Chem 2010;2:286-93.
4. Serov A, Padilla M, Roy AJ, et al. Anode catalysts for direct hydrazine fuel cells: from laboratory test to an electric vehicle. Angew Chem 2014;126:10504-7.
5. Varcoe JR, Atanassov P, Dekel DR, et al. Anion-exchange membranes in electrochemical energy systems. Energy Environ Sci 2014;7:3135-91.
6. Wang T, Wang Q, Wang Y, et al. Atomically dispersed semimetallic selenium on porous carbon membrane as an electrode for hydrazine fuel cells. Angew Chem 2019;131:13600-5.
7. Xue Q, Huang H, Zhu J, et al. Au@Rh core-shell nanowires for hydrazine electrooxidation. Appl Catal B Environ 2020;278:119269.
8. Liu F, Jiang X, Wang H, et al. Boosting electrocatalytic hydrazine oxidation reaction on high-index faceted Au concave trioctahedral nanocrystals. ACS Sustain Chem Eng 2022;10:696-702.
9. Rees NV, Compton RG. Carbon-free energy: a review of ammonia- and hydrazine-based electrochemical fuel cells. Energy Environ Sci 2011;4:1255.
10. Li F, Ji Y, Wang S, Li S, Chen Y. Ethylenediaminetetraacetic acid mediated synthesis of palladium nanowire networks and their enhanced electrocatalytic performance for the hydrazine oxidation reaction. Electrochim Acta 2015;176:125-9.
11. Ensafi AA, Abarghoui MM, Rezaei B. Facile synthesis of Pt-Cu@silicon nanostructure as a new electrocatalyst supported matrix, electrochemical detection of hydrazine and hydrogen peroxide. Electrochim Acta 2016;190:199-207.
12. Liu Y, Chen S, Wang A, Feng J, Wu X, Weng X. An ultra-sensitive electrochemical sensor for hydrazine based on AuPd nanorod alloy nanochains. Electrochim Acta 2016;195:68-76.
13. Chen L, Jiang L, Wang A, Chen Q, Feng J. Simple synthesis of bimetallic AuPd dendritic alloyed nanocrystals with enhanced electrocatalytic performance for hydrazine oxidation reaction. Electrochim Acta 2016;190:872-8.
14. Sakamoto T, Matsumura D, Asazawa K, et al. Operando XAFS study of carbon supported Ni, NiZn, and Co catalysts for hydrazine electrooxidation for use in anion exchange membrane fuel cells. Electrochim Acta 2015;163:116-22.
15. Zhang C, Yuan W, Wang Q, Peng X, Liu X, Luo J. Single Cu atoms as catalysts for efficient hydrazine oxidation reaction. ChemNanoMat 2020;6:1474-8.
16. Feng Z, Li D, Wang L, et al. In situ grown nanosheet Ni Zn alloy on Ni foam for high performance hydrazine electrooxidation. Electrochim Acta 2019;304:275-81.
17. Tang P, Lin X, Yin H, et al. Hierarchically nanostructured nickel-cobalt alloy supported on nickel foam as a highly efficient electrocatalyst for hydrazine oxidation. ACS Sustain Chem Eng 2020;8:16583-90.
18. Zhang T, Asefa T. Heteroatom-doped carbon materials for hydrazine oxidation. Adv Mater 2019;31:e1804394.
19. Sápi A, Rajkumar T, Kiss J, Kukovecz Á, Kónya Z, Somorjai GA. Metallic nanoparticles in heterogeneous catalysis. Catal Lett 2021;151:2153-75.
20. Theerthagiri J, Karuppasamy K, Lee SJ, et al. Fundamentals and comprehensive insights on pulsed laser synthesis of advanced materials for diverse photo- and electrocatalytic applications. Light Sci Appl 2022;11:250.
21. Yu Y, Theerthagiri J, Lee SJ, Muthusamy G, Ashokkumar M, Choi MY. Integrated technique of pulsed laser irradiation and sonochemical processes for the production of highly surface-active NiPd spheres. Chem Eng J 2021;411:128486.
22. Theerthagiri J, Lee SJ, Murthy AP, Madhavan J, Choi MY. Fundamental aspects and recent advances in transition metal nitrides as electrocatalysts for hydrogen evolution reaction: a review. Curr Opin Solid State Mater Sci 2020;24:100805.
23. Xiao C, Lu B, Xue P, et al. High-index-facet- and high-surface-energy nanocrystals of metals and metal oxides as highly efficient catalysts. Joule 2020;4:2562-98.
24. Koper MT. Structure sensitivity and nanoscale effects in electrocatalysis. Nanoscale 2011;3:2054-73.
25. Bentley CL, Kang M, Unwin PR. Nanoscale surface structure-activity in electrochemistry and electrocatalysis. J Am Chem Soc 2019;141:2179-93.
26. Yu N, Tian N, Zhou Z, et al. Pd nanocrystals with continuously tunable high-index facets as a model nanocatalyst. ACS Catal 2019;9:3144-52.
27. Rosca V, Koper MT. Electrocatalytic oxidation of hydrazine on platinum electrodes in alkaline solutions. Electrochim Acta 2008;53:5199-205.
28. Kim C, Dionigi F, Beermann V, Wang X, Möller T, Strasser P. Alloy nanocatalysts for the electrochemical oxygen reduction (ORR) and the direct electrochemical carbon dioxide reduction reaction (CO2 RR). Adv Mater 2019;31:e1805617.
29. Zhong H, Liu K, Zhang Q, Meng F, Bao D, Zhang X. Copper tetrazolate based metal-organic frameworks as highly efficient catalysts for artificially chemical and electrochemical CO2 conversion. Nano Select 2020;1:311-9.
30. Wang F, Mao L, Xie H, Mao J. Graphene derivatives and graphene composite electrocatalysts for N2 reduction reaction. Small Struct 2021;2:2000075.
31. Zhou Z, Shang S, Tian N, et al. Shape transformation from Pt nanocubes to tetrahexahedra with size near 10 nm. Electrochem Commun 2012;22:61-4.
32. Tian N, Zhou ZY, Sun SG, Ding Y, Wang ZL. Synthesis of tetrahexahedral platinum nanocrystals with high-index facets and high electro-oxidation activity. Science 2007;316:732-5.
33. Zhou ZY, Tian N, Li JT, Broadwell I, Sun SG. Nanomaterials of high surface energy with exceptional properties in catalysis and energy storage. Chem Soc Rev 2011;40:4167-85.
34. Álvarez-ruiz B, Gómez R, Orts JM, Feliu JM. Role of the metal and surface structure in the electro-oxidation of hydrazine in acidic media. J Electrochem Soc 2002;149:D35.
35. Xiao J, Liu S, Tian N, et al. Synthesis of convex hexoctahedral Pt micro/nanocrystals with high-index facets and electrochemistry-mediated shape evolution. J Am Chem Soc 2013;135:18754-7.
36. Xiao C, Tian N, Li W, et al. Shape transformations of Pt nanocrystals enclosed with high-index facets and low-index facets. CrystEngComm 2021;23:6655-60.
37. Chen Q, Vidal-iglesias FJ, Solla-gullón J, Sun S, Feliu JM. Role of surface defect sites: from Pt model surfaces to shape-controlled nanoparticles. Chem Sci 2012;3:136-47.
38. Zhong C, Hu WB, Cheng YF. Recent advances in electrocatalysts for electro-oxidation of ammonia. J Mater Chem A 2013;1:3216-38.
39. Chang F, Gao W, Guo J, Chen P. Emerging materials and methods toward ammonia-based energy storage and conversion. Adv Mater 2021;33:e2005721.
40. Li J, Zhang C, Zhang C, et al. Electronic configuration of single ruthenium atom immobilized in urchin-like tungsten trioxide towards hydrazine oxidation-assisted hydrogen evolution under wide pH media. Chem Eng J 2022;430:132953.
41. Chen S, Wang C, Liu S, et al. Boosting hydrazine oxidation reaction on CoP/Co mott-schottky electrocatalyst through engineering active sites. J Phys Chem Lett 2021;12:4849-56.
42. Dhiman N, Pradhan D, Mohanty P. Heteroatom (N and P) enriched nanoporous carbon as an efficient electrocatalyst for hydrazine oxidation reaction. Fuel 2022;314:122722.
43. Yan Y, Zhang JY, Shi XR, et al. A zeolitic-imidazole framework-derived trifunctional electrocatalyst for hydrazine fuel cells. ACS Nano 2021;15:10286-95.
44. Zhu Y, Zhang J, Qian Q, et al. Dual nanoislands on Ni/C hybrid nanosheet activate superior hydrazine oxidation-assisted high-efficiency H2 production. Angew Chem Int Ed Engl 2022;61:e202113082.
45. Li J, Li Y, Wang J, et al. Elucidating the critical role of ruthenium single atom sites in water dissociation and dehydrogenation behaviors for robust hydrazine oxidation-boosted alkaline hydrogen evolution. Adv Funct Mater 2022;32:2109439.
46. Li Y, Zhang J, Liu Y, et al. Partially exposed RuP2 surface in hybrid structure endows its bifunctionality for hydrazine oxidation and hydrogen evolution catalysis. Sci Adv 2020:6.
Cite This Article

How to Cite
Download Citation
Export Citation File:
Type of Import
Tips on Downloading Citation
Citation Manager File Format
Type of Import
Direct Import: When the Direct Import option is selected (the default state), a dialogue box will give you the option to Save or Open the downloaded citation data. Choosing Open will either launch your citation manager or give you a choice of applications with which to use the metadata. The Save option saves the file locally for later use.
Indirect Import: When the Indirect Import option is selected, the metadata is displayed and may be copied and pasted as needed.
About This Article
Copyright
Author Biographies
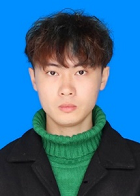
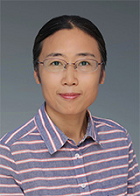
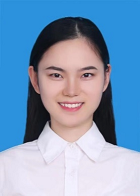
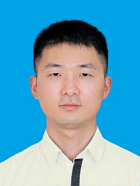
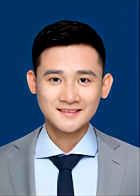
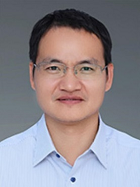
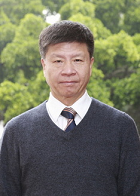
Comments
Comments must be written in English. Spam, offensive content, impersonation, and private information will not be permitted. If any comment is reported and identified as inappropriate content by OAE staff, the comment will be removed without notice. If you have any queries or need any help, please contact us at [email protected].