Two-dimensional materials: synthesis and applications in the electro-reduction of carbon dioxide
Abstract
The emission of CO2 has become an increasingly prominent issue. Electrochemical reduction of CO2 to value-added chemicals provides a promising strategy to mitigate energy shortage and achieve carbon neutrality. Two-dimensional (2D) materials are highly attractive for the fabrication of catalysts owing to their special electronic and geometric properties as well as a multitude of edge active sites. Various 2D materials have been proposed for synthesis and use in the conversion of CO2 to versatile carbonous products. This review presents the latest progress on various 2D materials with a focus on their synthesis and applications in the electrochemical reduction of CO2. Initially, the advantages of 2D materials for CO2 electro-reduction are briefly discussed. Subsequently, common methods for the synthesis of 2D materials and the role of these materials in the electrochemical reduction of CO2 are elaborated. Finally, some perspectives for future investigations of 2D materials for CO2 electro-reduction are proposed.
Keywords
INTRODUCTION
The concentration of greenhouse gas CO2 has risen by 100 ppm since the industrial revolution. The average temperature has been rising at an average rate of 0.1 °C per decade for nearly half a century, which results in serious global warming[1-3]. From another perspective, CO2 is an abundant, clean, and cheap feedstock for the production of value-added chemicals. Hence, it is of great ecological and social significance to utilize and convert CO2 into high-value-added chemical products[4-6]. Nonetheless, CO2 has thermodynamically stable and chemically inert properties, and thus its conversion is highly energy intensive. Considering the prominent role of CO2 in global warming and the chemical characteristics of CO2, numerous chemical technologies have been developed for CO2 conversion, including thermal catalysis[7-9], photocatalysis[10-12], and electrocatalysis[13-15]. The development of highly effective catalysts and related equipment for CO2 transformation has boomed in recent years[16]. Among them, the electro-reduction of CO2 to value-added chemicals benefits from low investment in equipment, high efficiency, and environmental acceptance[17-20].
The electrochemical reduction of CO2 is a multi-electron transfer process, which mainly occurs at the electrode and the electrolyte interface. This process consists of four steps: (i) CO2 is chemically adsorbed on the electrode surface; (ii) CO2 is converted into CO2•− by one-electron attacks; (iii) Multiple electrons and protons are transferred to CO2•− for the construction of C-H and C-C bonds; and (iv) Products are released from the electrode[21].The half-reactions and corresponding standard reduction potentials for the electro-reduction of CO2 to various products have been elucidated through thermodynamic studies, as illustrated in Figure 1.
Figure 1. Half reactions and corresponding standard reduction potentials vs. reversible hydrogen electrode (RHE) (E0/V vs. RHE) for the electrochemical reduction of CO2 to various products.
The electro-reduction of CO2 to related products is a multi-step reduction. In spite of the low chemical reactivity of CO2, the theoretical potential required for products is not a large negative value[22]. In fact, the first hurdle of CO2 electro-reduction is the potential barrier for CO2 activation (CO2 + e- = CO2•−), which is
Current density and selectivity are two evaluation indicators in CO2 electro-reduction. A large current density indicates a fast reaction rate to provide a high yield of products from CO2, and high selectivity can ease separation to reduce cost. Electrode materials play a decisive role in electrocatalytic performance. Hence, the design and synthesis of electrode materials with high activity, selectivity, and stability are significant for highly efficient CO2 electro-reduction. 2D materials are defined as a type of nanomaterials with a layer-like morphology and small thickness (normally 1-100 nm) in which electrons move on the nanoscale in two dimensions[25]. 2D materials have been developed for various applications, such as energy conversion[26-28], catalysis[29-31], sensing[32-34], photodetector[35-37], and memristor[38-40], owing to their unique 2D geometry, nanoscale thickness, and high surface-to-volume ratio. 2D structures, such as nanoplates, nanosheets, and nanoflakes, have been using for preparation of various materials, including metals, metal oxides, metallic sulfides, and carbon materials[41-44]. Until now, numerous 2D materials have been designed and prepared for CO2 electro-reduction[45-47]. For commercial applications of electrocatalysts, high yield and controllable fabrication techniques are essential. Versatile methods for the preparation of 2D materials, such as exfoliation, extraction, chemical or physical vapor deposition, and wet-chemical synthesis, have been developed. Different synthesis routes have different advantages. These strategies determine the morphology of materials and further affect their properties, providing opportunities for their utilization in electrocatalysis.
This review covers the current progress on CO2 electro-reduction using 2D material electrodes with a focus on the (i) advantages of 2D materials for CO2 electro-reduction, (ii) strategies for 2D nanomaterial synthesis, and (iii) catalytic performance of 2D materials in the electrochemical reduction of CO2.
ADVANTAGES OF 2D NANOSTRUCTURES FOR CO2 ELECTRO-REDUCTION
Electrochemical reduction of CO2 is a process involving gas-solid-liquid interactions[48,49], and its current density and Faradaic efficiency (FE) are largely affected by the specific surface area of electrode materials. Generally, a higher specific surface area guarantees more adsorption and active sites, leading to better catalytic performance. In addition, the concentration gradient of CO2 and reaction intermediates are related to CO2 diffusion on the electrode surface. The adsorption/desorption thermodynamics and kinetics of intermediates determine the selectivity and production rate of target products. Thus, materials with special morphologies and structures play a crucial role in catalyzing this process.
The electrochemical reduction of CO2 is surface reaction, and thus 2D materials are suitable as catalysts because of the following advantages. First, 2D materials have a unique planar structure, which promotes interactions with the substrate or active components as well as the formation of heterojunctions to realize comprehensive regulation of electrocatalysis[50]. Second, 2D materials have enhanced electronic properties, such as high electron mobility and carrier concentration[51,52], which increase electron transfer from the electrode to CO2. Third, 2D materials have a high proportion of uncoordinated sites and large specific surface areas, which increases interactions between CO2 and the catalyst[53]. Exposed surface atoms in 2D materials can escape from their respective lattices to form defect sites, and these thermodynamically unstable sites can trap electrons and shorten electron transport and ion diffusion distances[54,55]. Owing to the low atomic coordination number at the edge, the activity at the edge is higher than that at the basal plane in 2D materials[56]. Fourth, a multi-layer or single-layer structure increases the confinement effect, which improves the catalytic performance[57,58]. The surface of 2D materials can be modified to produce special gas trapping cavities, where interactions between the electrode, electrolyte, and CO2 gas are enhanced[59,60]. Fifth, pores in 2D materials are fully exploited owing to the ultrathin structure. Porous structures in 2D materials can effectively amplify the differences in mass transfer efficiency as well as intermediate diffusion during CO2 reduction.
In summary, 2D materials have geometries, electronic structures, and defects that are suitable for CO2 electro-reduction. Owing to their large specific surface area, 2D materials can serve as supports for hybrid materials. In addition, the unique atomic-layer-thick structure provides an ideal platform for revealing atomic-scale mechanisms. The rational development and exploitation of distinct structural and electronic properties of 2D materials endow 2D materials with more opportunities for broad applications in the field of CO2 electro-reduction.
SYNTHESIS OF 2D NANOSTRUCTURES
The electrocatalytic performances of 2D materials are mainly determined by the morphology, structure and chemical properties of the materials, which in turn are closely related to the synthesis methods. Since the discovery of graphene, many efficient routes have been developed for the synthesis of 2D materials, allowing researchers to design more promising 2D materials. Top-down and bottom-up routes are commonly used for the synthesis of 2D materials[61-63]. Top-down routes, such as exfoliation and extraction procedures, are easily performed without special equipment, and thus they are effective for the synthesis of inorganic materials, such as graphene and metal dichalcogenides. Bottom-up routes, including chemical/physical vapor deposition and wet-chemical syntheses, provide 2D materials with accurately controlled morphologies and structures, and thus they are frequently used to prepare materials with unique structures. In this section, several typical routes for the fabrication of 2D materials are briefly described.
Exfoliation
Exfoliation requires external mechanical forces to peel off 2D materials with a single or few layers from bulk objects. This method relies on driving forces, such as mechanical and ultrasonic forces, to overcome intermolecular interactions, such as the van der Waals force, between atomic layers. This process is simple to operate and produces few defects in the resulting material, although it suffers from low effectiveness and poor repeatability, which limits its practical applications.
Mechanical exfoliation has been a common method for the fabrication of emergent 2D materials
Figure 2. Synthesis of 2D materials by exfoliation. (A) Graphene films synthesized by mechanical exfoliation[64]. (B) Schematic diagram of Au-assisted mechanical exfoliation. (C) 2D materials synthesized by Au-assisted mechanical exfoliation[66]. (D) Schematic diagram of CTAB-assisted material swelling[69]. 2D: 2-dimensional; CTAB: hexadecyl trimethyl ammonium bromide.
Ultrasonic exfoliation in the liquid phase relies on the cavitation effect. When bulk materials are subjected to an ultrasonic wave in a suitable solvent, micro-gas core cavitation bubbles produced in the solvent expand the space between the layers of the material, thus producing the 2D material. Coleman et al. reported in detail that ultrasonic exfoliation of transition metal dichalcogenides, such as BN, MoS2, WS2, MoSe2, MoTe2, TaSe2, NbSe2, NiTe2, and Bi2Te2, in organic solvents can be used to produce 2D materials with few layers[67]. Metal oxide and hydroxide with limited layers can also be produced by ultrasonic exfoliation. Compared with transition metal dichalcogenides, which are stabilized by weak intermolecular interactions, such as the van der Waals force, metal oxides and hydroxides are stabilized by strong ionic interactions, which makes exfoliation difficult[68]. Although ultrasonic exfoliation is the most promising strategy for the industrial production of 2D materials, this strategy has fatal defects. For example, (i) the number of layers and thickness are difficult to control and (ii) the recovery rate is low, which confines the method to the laboratory.
Intercalation-based exfoliation has been widely explored owing to the unique characteristics of high-yield monolayers. Suitable intercalation species are vital for effective intercalation and determine whether intercalation proceeds. For example, surfactants are often used for the production of 2D nanosheets. Dakhchoune et al. reported that intercalation of surfactant hexadecyl trimethyl ammonium bromide (CTAB) into sodalite precursors leads to material swelling[69]. Consequently, the lattice spacing increases from 1.4 nm to 3.1 nm [Figure 2D], indicating that interlayer interactions are weakened by the intercalation of CTAB.
Chemical vapor deposition
Chemical vapor deposition (CVD) is one of the most commonly used methods for the synthesis of 2D materials[70]. In this technique, a thin solid layer is deposited on the surface of a substrate through vapor-phase chemical reactions in a high-temperature gas. Various solid-state layers (e.g., single crystals, continuous thin films, and heterojunctions) can be produced on substrates by adjusting the operating temperature, flow gas species, phase composition, and substrate. Numerous functional van der Waals heterostructures can also be created by this method[71] [Figure 3A-D]. In contrast to exfoliation and extraction, CVD allows control of the number of layers as well as the density and purity of products through changes in the synthesis conditions.
Figure 3. Synthesis of 2D materials by CVD. (A) Schematic diagram of the CVD method. (B-D) Schematic diagram and TEM images of graphene/hBN heterostructures. Here, carbon atoms are represented by blue spheres, boron is shown in yellow, and nitrogen is in purple[71]. (E-G) Synthesis of 2D Fe single-crystal nanoflakes by space-confined CVD[77]. 2D: 2-dimensional; CVD: chemical vapor deposition; TEM: transmission electron microscopy.
CVD has become an important method for the preparation of 2D materials since it was used to prepare graphene in 2009[72,73]. Browne et al. synthesized MoS2 and WS2 materials to serve as electrodes[74]. The surface of as-prepared MoS2/WS2 is rougher than that of commercial materials. Li et al. used methane to prepare graphene films with a large surface area on a copper substrate by CVD[73]. The as-prepared graphene film can be easily transferred to alternative substrates for the fabrication of functional devices. Yin et al. synthesized high-quality nonlayered Fe2O3 nanosheets with a thickness of 1.95 nm, which exhibit lattice relaxation owing to the ultrathin structure[75]. Although the growth of 2D materials on substrates is rapid, the direct growth of 2D metal/metal oxides onto substrates is restricted because of the high melting point of metals/metal oxides. Introducing molten salts as additives can effectively reduce the reaction temperature and promote the growth of high-quality 2D materials. For instance, Zhou et al. reported a new method to synthesize 47 compounds by adding NaCl and KI as adjuvants[76]. Despite various efforts focused on synthesizing 2D materials by CVD, poor repeatability and unscalable production restrict the industrial application of CVD.
As mentioned above, the thickness and size of 2D materials can be precisely controlled by adjusting the working temperature, carrier gas rate, and chamber pressure. Space-confined CVD can be used to achieve the growth of high-quality 2D materials. Recently, Li et al. used Te-assisted CVD to precisely synthesize 2D Fe single-crystal nanoflakes with different thicknesses[77] [Figure 3E-G]. Xu et al. used space-confined CVD to grow homogeneous 1T’-MoTe2 monolayers with a lateral size of 100 μm in batches, demonstrating that the confined space can increase the vapor pressure of sulfur to extend the lifetime of monolayer tellurides from several minutes to at least 24 h[78].
Hydrothermal and solvothermal methods
Hydrothermal and solvothermal methods are widely used to prepare various 2D materials with high yields. Both methods are based on heterogeneous chemical reactions occurring at high temperature and pressure during which substances are dissolved and recrystallized. The difference between the two methods is the solvent. Water is used as the solvent in hydrothermal methods, while non-aqueous organic solvents are used in solvothermal methods. Materials prepared by hydro/solvothermal methods have advantageous characteristics, such as high crystallinity, small particle size, and uniform distribution.
Among various 2D materials, metal oxides have attracted considerable attention in the fields of catalysis, energy conversion, and electronics owing to their low cost and large surface area. Sun et al. developed a general solvothermal method to prepare various transition metal oxide nanosheets, such as ZnO, WO3,
Figure 4. Synthesis of 2D metal oxide nanosheets by the hydrothermal method. (A) Schematic diagram of the synthesis route. TEM images of (B) Co3O4, (C) TiO2, (D) ZnO, and (E) WO3[79]. 2D: 2-dimensional; TEM: transmission electron microscopy.
Electrodeposition
Electrochemical deposition is a liquid-phase process based on redox reactions by which 2D materials are easily formed. This method has numerous advantages, including mild conditions, low cost, high safety, easy operation, and high controllability. The morphology, structure, and physicochemical property of products can be controlled by adjusting various conditions, including the pH of the solution, potential, time, and temperature, during electrodeposition.
Electrodes prepared by electrodeposition have very low contact resistance, which promotes electron transfer in functional devices. Rabiee et al. grew uniform Bi-based nanosheets on a substrate by pulse electrodeposition[87]. In contrast, a Bi-based bulk material was obtained by continuous electrodeposition. Abdelazim et al. reported the lateral growth of MoS2 on an insulating surface[88]. The highly anisotropic growth rate of MoS2 can be controlled by simple electrodeposition to obtain a lateral to vertical growth ratio exceeding 20. This paves a new pathway to precisely control the growth direction to obtain 2D materials. Polymetallic alloys have shown great potential in various applications, and co-deposition of versatile metals can be realized with electrodeposition by adjusting the pH of the solution or applied voltage. For example, Feng et al. used electrodeposition in a solution of pH 10 to obtain an active borate-intercalated NiCoFe-LDH, which is susceptible to redeposition to recover catalytic activity, further confirming the advantage of electrodeposition[89]. Shen et al. synthesized amorphous 2D FeMn by electrodeposition using sodium citrate as a structure-directing agent[90] [Figure 5A-C]. OH- is produced near the surface of the cathode, and metal ions are deposited with OH- at the cathode to form hydroxides.
Figure 5. Synthesis of 2D materials by electrodeposition. (A) Schematic diagram of the preparation of FeMn-based nanosheets.
Despite recent achievements in the electrodeposition of 2D materials, the mechanism remains difficult to demonstrate. Tan et al. used transmission electron microscopy (TEM) to visualize material synthesis, including nucleation sites, growth mechanisms, and structures formed during electrochemical reactions[91]. This promising technology can be used to observe an electron beam-stable and low-contrast extended electrode area with high resolution [Figure 5D].
Extraction
To date, many new types of 2D materials have been developed for functional devices. 2D transition metal carbides and nitrides (MXenes) have good electrical properties owing to the inner conductive carbide layer and hydrophilic transition metal oxide surface, and thus they are promising materials for the fabrication of batteries and catalysts.
The most effective synthesis route of MXenes is the selective etching of “A” layers from an Al-containing MAX phase, where A represents a III A or IV A element (e.g., Al, Ga, Si, or Ge) and X represents either C or N. Lukatskaya et al. and Ghidiu et al. synthesized various MXenes by selective extraction, electrochemical etching, and ion intercalation[92-94]. The as-prepared 2D materials are applied in Li-ion batteries, which acquire excellent electricity performance.
In addition to the methods above, researchers have explored many other routes for the synthesis of 2D materials. Among the multitude of strategies, templated synthesis can easily and directly realize 2D materials with controlled structures. For example, Liu et al. synthesized a series of mesoporous materials with a single-layer structure[95]. Phenolic formaldehyde resin, a flexible template, is beneficial for the adsorption and subsequent confinement of inorganic precursors on the salt surface. Surface-limited cooperative assembly allows for large-scale production, and 14.1 g of mesoporous TiO2 with single-layer structure can be synthesized per batch. Although templated synthesis has been extensively explored for the preparation of 2D materials, the long preparation time and high cost restrict its wide application in industry. Developing template-free preparation methods is of significance for the mass production of 2D materials. Zhang et al. developed a general method for control-oriented growth of MOF nanosheets with ultrathin thicknesses and abundant unsaturated coordination metal sites using CO2 as a capping agent[96]. The process is template-free and CO2 can be easily removed by depressurization after synthesis. Atomic layer deposition has emerged as a powerful technique to prepare nanofilms with a thickness approaching the Debye length. Ko et al. reported the preparation of a large-area WS2 nanosheet with a precisely controlled number of layers by atomic layer deposition[97]. These methods can be used for reference and guidance in the development and application of 2D materials for CO2 electrocatalytic reduction.
ELECTROCHEMICAL REDUCTION OF CO2 OVER 2D NANOSTRUCTURES
Various 2D nanostructures have been extensively explored for the electro-reduction of CO2 owing to their unique structure and properties. Versatile electrode materials, including metals, metal oxides, metal dichalcogenides, and carbon-based nanomaterials, have shown potential in CO2 electrocatalytic reduction [Table 1]. In this section, advances in 2D materials for CO2 electrocatalytic reduction a reviewed and the related mechanism is discussed.
Summary of 2D-based materials developed for CO2 electrocatalytic reduction
Catalysts | Electrolyte | Products | FE | Potential | j (mA cm-2) | Refs. |
Ag nanosheet | 0.5 M NaHCO3 | CO | 95 | -0.8 V vs. RHE | 10 | [98] |
Ag foil | 75 | ~0.3 | ||||
Zn nanosheet | 0.5 M NaHCO3 | CO | 86 | -1.13 V vs. RHE | 6 | [99] |
Zn foil | 9.5 | ~1 | ||||
Sn sheet confined in graphene | 0.1 M NaHCO3 | formate | 89 | -1.8 V vs. SCE | 21.1 | [100] |
Bulk Sn | 44.5 | 1.6 | ||||
Cu nanosheet | 0.1 M K2SO4 | ethylene | 83.2 | -1.18 V vs. RHE | 58.8 | [101] |
Cu nanoparticles | 37.2 | 36.4 | ||||
Sn nanosheet | 0.5 M NaHCO3 | formate | 83 | -0.9 vs. RHE | 14 | [102] |
Sn foil | 25 | 6 | ||||
Cu plates | 0.5 M KCl | ethylene | 74.9 | -0.9 vs. RHE | 50 | [103] |
Cu nanoparticles | 52 | 10 | ||||
Zn nanosheet | 0.1 M KHCO3 | CO | 90 | -1.0 V vs. RHE | 100 | [104] |
Zn nanoparticles | 70 | 50 | ||||
Co nanosheet | 0.1 M Na2SO4 | formate | 90.1 | -0.85 V vs. SCE | 10.59 | [105] |
Bulk Co | 25 | 68.0 | ||||
Co3O4 nanosheets | 0.1 M KHCO3 | formate | 64.3 | -0.88 V vs. SCE | 0.68 | [106] |
Bulk Co3O4 | 18.5 | 0.034 | ||||
Bi nanosheet | 0.5 M NaHCO3 | formate | ~90 | -1.74 V vs. SHE | 24 | [107] |
Bi nanoparticles | ~60 | 6 | ||||
ZnO nanosheet | 0.1 M KHCO3 | CO | 83 | -1.1 V vs. RHE | 16.1 | [108] |
Bi2WO6 nanosheet | 0.5 M BmimPF6/MeCN | CO | 91 | -2.4 V vs. Ag/Ag+ | 43 | [109] |
N-codoped graphenes nanosheet | 0.5 M KHCO3 | formate | 64 | -0.80 V vs. RHE | 4 | [110] |
SnS2 | 0.1 M KHCO3 | formate | 93.3 | -0.75 V vs. RHE | 55 | [111] |
InN nanosheet | 1 M KOH | formate | 91 | -0.9 V vs. RHE | 46 | [112] |
Mo-Bi bimetallic chalcogenide nanosheet | 0.5 M BmimBF4/MeCN | methanol | 71.2 | -0.7 V vs. SHE | 12.1 | [85] |
FeTPP[Cl]/Cu | 1 M KHCO3 | ethanol | 41 | -0.82 V vs. SHE | 124 | [113] |
Metal materials
Zero-valence metals are the most popular electrode materials for CO2 electro-reduction owing to their high conductivity, high stability, controllable crystal faces, and ease of CO2 activation.
Noble metals
Noble metals are considered the most effective catalysts[114,115], and electrochemical reduction of CO2 over noble metal-based electrodes has been extensively studied in recent years[116,117]. The particle size determines the catalytic performance because larger particles have fewer active sites, whereas smaller particles favor H2 evolution over CO2 reduction. Noble metal nanosheets exhibit excellent catalytic performance in CO2 electro-reduction compared with their nanoparticles. For instance, Lee et al. prepared Ag nanosheets
Figure 6. Electrochemical reduction of CO2 over 2D noble metals. (A) Plot of current density vs. potential over Ag electrodes. (B) FECO and FEH2 vs. potential over Ag electrodes. (C) Tafel plots[98]. (D) Plot of current density vs. potential over Pd electrodes. (E) FECO vs. potential over Pd electrodes. (F) Electrochemical active surface area of different catalysts. (G) Schematic illustration of different Pd materials[118]. 2D: 2-dimensional.
Different crystal planes exhibit different catalytic activities for the conversion of CO2 to products, and thus regulation of the crystal planes of nanosheet is an effective strategy to obtain high current density and FE for certain products. For example, Zhao et al. demonstrated that Pd nanosheets prepared by co-precipitation exhibit excellent catalytic activity in the electro-reduction of CO2 to CO at the moderate overpotential of 590 mV[118] [Figure 6D-F]. Pd nanosheets with dominant (111) facet sites are transformed into more active (100) sites after 1 h of electrolysis. The reconstruction of crystal planes not only increases the density of active sites but also reduces the binding energy with CO intermediates, leading to high CO selectivity [Figure 6G].
It is worth mentioning that noble metal nanosheets favor CO over other carbon products of CO2 electro-reduction because noble metals can strongly adsorb the *COOH intermediate, which is further reduced to CO* on the electrode surface. In addition, CO* is weakly adsorbed on the surface of noble metals, and thus CO easily desorbs from the surface of noble metal nanosheets.
Transition metals
Noble metals are expensive, and thus cost-effective transition metals are increasingly used for CO2 electro-reduction[119]. For example, Zn nanosheets prepared by the hydrothermal method perform better than Zn-foil in the electro-reduction of CO2 and show high selectivity toward CO[99]. 2D nanostructures exhibit high catalytic activity in CO2 electro-reduction owing to the high density of edge sites. Xiao et al. reported that hexagonal Zn nanoplates prepared by cathodic electrochemical deposition [Figure 7A and B] are suitable for the electrochemical reduction of CO2 to CO at a wide potential range[120]. FECO over Zn nanoplate can reach 94.2%, which is 2.2 times higher than that over Zn foil [Figure 7C]. The high catalytic performance of Zn nanoplates is attributed to the exposed Zn (100) facets and edges, where COOH* can form more easily than at any other facet, indicating that an increased edge-to-corner ratio can enhance the reactivity.
Figure 7. Electrochemical reduction of CO2 over 2D transition metals. (A) SEM image of hexagonal Zn nanoplates. (B) TEM image of Zn nanoplates. (C) Plot of FECO vs. potential over different Zn electrodes[120]. (D) Schematic diagram of the partially oxidized and pure Co 4-atomic-layer. (E) Plot of FEformate vs. potential over different Co electrodes[105]. (F) Plot of current density vs. potential over different Cu electrodes. (G) Plot of FEC2H4 vs. potential over different Cu electrodes. (H) Plot of jC2H4 vs. potential over different Cu electrodes[101]. 2D: 2-dimensional; TEM: transmission electron microscopy.
Co is the first transition metal used in CO2 electro-reduction. Gao et al. prepared a 4-atom-thick Co sheet with and without surface Co oxide for CO2 electro-reduction [Figure 7D], achieving a high FEformate of 90.1% at a low overpotential of 0.85 V vs. SCE[105] [Figure 7E]. Moreover, the catalyst exhibits long-term stability, and FEHCOOH remains at a high level of approximately 90% within 30 h. Volumetric CO2 adsorption measurements were used to demonstrate that changes in the oxidation state of Co and increases in the surface area synergistically favor CO2 adsorption, thereby enhancing catalytic performance. Porphyrin Co is one of the most efficient species for CO2 electro-reduction because of the strong interaction between the metal and ligand. Han et al. prepared Co single-site catalysts by assembling Co-porphyrin molecules[121]. The catalyst can promote the conversion of CO2 to CO with an FE of 96 % at an overpotential of 500 mV. This outstanding catalytic performance is attributed to the increase in the energy of d orbitals originating in the strong repulsive force between the ligand and electrons in the z-direction. Yin et al. recently prepared Co nanosheets for the conversion of CO2 to C2+ products[122]. Co nanoparticles were first prepared by a hydrothermal reaction and then converted to Co nanosheets by exfoliation in formamide. Remarkably, ethanal is the main product of the Co nanosheet electrode with an FEethanal of 60%. Ultraviolet photoelectron spectroscopy was used to show that Co nanosheets have a wide electronic distribution near the Fermi level, resulting in rapid electron transfer. The electron-rich environment around Co 3d of Co nanosheets is beneficial for CO-CO coupling.
Bi is cheap and environmentally acceptable because of its low toxicity. Bi precursors are used to prepare Bi nanosheets in situ by a hydrothermal method[123], during which O is introduced into the Bi nanosheets. The as-prepared electrode can achieve an FEformic acid of > 90% with a current density of approximately
Sn is another transition metal that can be used to convert CO2 into valuable formate by electrochemical methods. A series of Sn nanosheets have been designed and synthesized for the electro-reduction of CO2. Wu et al. prepared a very sensitive Sn nanosheet with a layer thickness of approximately 9.2 μm by directly spraying Sn catalyst ink onto a gas diffusion layer[125]. The Sn nanosheet electrode exhibits a high FEformate, owing to the desirable proton concentration, electronic conduction, and gas diffusion of the 2D structure. The thickness of Sn nanosheets also influences the current density, FE, and operating potentials. An ultrathin Sn layer can significantly enhance these reduction-related properties, but an excessively thin layer may increase the oxidation rate of Sn, leading to poor stability. This problem can be resolved by confining metal layers into carbon materials[126,127]. Lei et al. reported a method to prepare Sn quantum sheets confined in graphene[100]. The confined nanostructure enhances CO2 adsorption and rate-limiting electron transfer and stabilizes the CO2•− intermediate. As a result, the graphene confined Sn quantum sheet displays a current density of 21.1 mA cm-2 and FEformate of 89% at -1.8 V versus SCE, which is higher than that of the mixture of Sn nanoparticles and graphene. Electrochemical impedance spectroscopy was used to confirm the very low interfacial charge-transfer resistance, suggesting that the existence of 2D graphene can improve the conductivity of the electrocatalyst. Moreover, the electronic state of the complex interface can stabilize reaction intermediates, reducing the free energy barrier for the formation of products.
Among the elements, Cu is the most effective in harvesting various products, such as CO, HCOOH, and C2+ products, because Cu has a suitable binding energy between products and the surface of catalysts according to the Sabatier principle[128]. Thus, exploring Cu-based electrodes for CO2 electro-reduction has become a vigorous research topic. Recently, numerous efforts have been dedicated to developing 2D Cu materials for the electro-reduction of CO2 to hydrocarbons and alcohols [129].
In a pioneering work, Hori et al. reported the efficient electro-conversion of CO2 to hydrocarbons and alcohols over an electrodeposited Cu sheet electrode in aqueous inorganic salt solutions[130]. KCl, K2SO4, KClO4, and dilute HCO3- electrolytes favor the formation of ethylene and alcohols, while concentrated HCO3- and phosphate solutions prefer the generation of methane. Zhang et al. demonstrated that nanodefective Cu nanosheets (n-CuNS) effectively reduce CO2 into C2 products[101]. FEC2H4 over n-CuNS electrode is 83.2%, which is higher than that over Cu nanosheets with a smooth surface (CuNS, 45.7%) and Cu nanoparticles (CuNP, 37.2%). The current density and FEC2H4 over different electrodes at different applied potentials are shown in Figure 7F-H. Interestingly, n-CuNS does not produce CO, while CuNS and CuNP convert CO2 into CO in high quantities. Therefore, nano defects in Cu nanosheet significantly improve the current density because CO2 adsorption and C-C coupling are boosted by the enrichment and confinement of reaction intermediates and OH-. Chen et al. investigated the effects of grain boundary (GB) density and Cu+/Cu0 ratio during CO2 electro-reduction[131]. As the GB density increases, FEC2H4 first increases and then decreases, which is related to the content of Cu+ in the catalyst during the reaction. Therefore, the GB density can activate CO2 molecules, and Cu+ can promote selectivity toward C2+ products.
2D metal nanomaterials have highly anisotropic characteristics, abundant coordination sites, and a special electronic structure. Moreover, 2D metals have a unique structure in the absence of external fields, which leads to special properties that can be exploited for electrocatalysis.
Metal oxide materials
Since the pioneering report on the catalytic behaviors of metal oxide semiconductor powders in the 1970s[132], the past decades have witnessed great progress in the fundamental study of metal oxide catalysts. Semiconductor metal oxides for CO2 electro-reduction offer several advantages, including low cost, easy maintenance, and the potential to generate C2+ products. At present, many kinds of metal oxides have been used for CO2 electro-reduction. It is worth pointing out that metal oxides are usually unstable and partially or entirely convert to low-valence metal oxide or zero-valence metallic species under negative potentials. Moreover, the final state and reconstruction of electrodes during electrolysis play important roles in CO2 conversion[133,134].
Different ZnO nanosheets have been synthesized and employed as excellent electrodes for CO2 electro-reduction[135,136]. A series of strategies, such as hydrothermal method, spray-coating, and electrodeposition, was used by Luo et al. to prepare ZnO precursors with different morphologies[137]. ZnO is reconstructed to a porous sheet-like structure, specifically hexagonal Zn crystals, regardless of the initial morphology and reduced to the metallic state during the electrochemistry process. ZnO nanosheets exhibit an FECO of 91.6% with a current density of 200 mA cm-2 at -0.62 V vs. RHE in a flow reactor. Overall, the reconstruction provides a high surface area, which enhances the catalytic activity and potentially promotes gas diffusion.
In addition to ZnO nanosheets, a number of other 2D metal oxide nanostructures, such as SnO2, Co3O4, and CuO, have been tested for CO2 electro-reduction. In particular, porous nanosheets are more advantageous. Han et al. synthesized porous SnO2 nanosheets by a two-step method[102]. A precursor of SnS2 nanosheets is first obtained by a hydrothermal method and subsequently annealed to obtain SnO2 nanosheets with a highly porous architecture. The calcination step increases the surface area and active sites of SnO2, resulting in a small onset potential, large current density, high FEHCOOH, and high stability. Gao et al. prepared Co3O4 nanolayers for the selective electro-reduction of CO2 to formate[138]. The catalyst exhibits good stability, and the current density negligibly changes within 40 h. The materials have abundant oxygen vacancies, which serve as active sites to stabilize reduction intermediates and reduce the activation energy barrier. Moreover, the valence of Co3O4 is retained, although the crystal structure is destroyed after the reaction owing to the existence of oxygen vacancies.
CuO is widely used in electro-reduction of CO2 to C2+ products. CuO is reduced to Cu+ and metallic Cu during CO2 electro-reduction[139,140]. Liu et al. prepared an oxygen-rich ultrathin CuO nanoplate for the electro-reduction of CO2 to C2H4 with an FEC2H4 of 84.5%, which can be maintained for at least 55 h[103] [Figure 8A]. At the optimal current density of 75 mA cm-2 and a full-cell voltage of -3.1 V, the FEC2H4 is 77.3% and the energy efficiency of C2H4 is 28.9% [Figure 8B and C]. Cu/Cu2O heterogeneous interfaces are formed through the self-evolution of CuO nanoplate arrays during electrocatalysis. The impressive performance is derived from Cu/Cu2O interfaces. The enhanced adsorption of the *OCCOH intermediate on the surface of Cu(110)/Cu2O(110) facilitates the production of C2H4. A network comprising Ag and
Figure 8. Electrochemical reduction of CO2 over 2D metal oxides. (A) Schematic illustration of the electrochemical reconstruction of CuO nanosheets. (B) Plot of FE of various products vs. potential over the CuO electrode. (C) Plot of full cell potential and EEC2H4 vs. current density over the CuO electrode[103]. (D) Schematic illustration of the BiCuSeO superlattice. (E) EDS mapping images of BiCuSeO. (F) Chronoamperometric curves at different potentials over the BiCuSeO electrode. (G) FEformate, FECO, and FEH2 vs. potential over the BiCuSeO electrode[142]. 2D: 2-dimensional; FE: Faradaic efficiency; EDS: energy dispersive spectroscopy.
Recently, polymetallic oxides have been developed for CO2 electro-reduction owing to their structural flexibility. A tangible superlattice model of metal oxide and selenide for CO2 electro-reduction was demonstrated by Duan et al.[142]. The as-prepared BiCuSeO (layer stacked superlattices) [Figure 8D and E] shows an FEformate of > 90% at a low overpotential [Figure 8F]. The outstanding catalytic activity is attributed to the active 2D Bi2O22+ sublayer. It is worth pointing out that the layer-stacked superlattices can inhibit the reduction of metal oxide and HER, resulting in long-term stability and a high FEformate over electrocatalysts [Figure 8G]. Zhao et al. reported that 2D ZnGa2O4 nanoplates prepared by an ion-exchange method can reduce CO2 into CO at the relatively low applied potential of -1.4 V vs. Ag/AgCl, with a high FECO of 96%, owing to the high specific area of the nanoplates[143]. During CO2 electro-reduction, partial Zn2+ reduces to Zn+, and the Zn2+/Zn+ redox couple favors the activation of CO2.
2D transition metal oxides have a large specific surface area, atomic-scale thickness, and abundant dangling bonds, leading to high catalytic performance in CO2 electro-reduction. In principle, metal oxides are first reduced to the corresponding metals, and the as-reduced metal oxides become the active species for CO2 electro-reduction. Defects and other active components are introduced into the final electrocatalysts during the reduction of metal oxides.
2D metal dichalcogenides
Transition metal dichalcogenides, such as MoS2, WS2, MoSe2 and WSe2, are common 2D nanostructures and can be easily prepared by simple exfoliation and CVD methods. These materials have a high surface-to-volume ratio and rich active sites, and thus they have the potential to convert CO2 into valuable products.
During the past decades, MoS2 has attracted considerable attention owing to its special 2D structure and property. Asadi et al. reported that excess d electrons on Mo-edge atoms and a low work function result in high catalytic performance[144] [Figure 9A and B]. The catalyst shows a current density of 65 mA cm-2 at
Figure 9. Electrochemical reduction of CO2 over 2D metal dichalcogenides. (A) PDOS for the spin-up channel of Mo atoms at the edge and within the lattice. (B) PDOS for the spin-up channel of the s, p, and d orbitals of Mo-edge atoms[144]. (C) Schematic illustration of the synthesis of H-SnS2 nanosheets. (D) TEM image of H-SnS2 nanosheets. (E) HRTEM image of H-SnS2 nanosheets. (F) XRD patterns of H-SnS2 nanosheets. (G) Plot of current density vs. potential over SnS2 and H-SnS2 electrodes. (H) Structural model of H-SnS2 nanosheets. (I) Plot of FE of products vs. potential over SnS2 and H-SnS2 electrodes[148]. 2D: 2-dimensional; PDOS: projected density of states; TEM: transmission electron microscopy; FE: Faradaic efficiency.
Asadi et al. also synthesized a series of 2D transition metal dichalcogenides, including MoS2, WS2, MoSe2 and WSe2, by a chemical vapor transport method for CO2 electro-reduction[147]. Among the as-prepared materials, WSe2 shows the best catalytic performance, leading to an FECO of 24% with a current density of 18.95 mA cm-2 at -0.164 V vs. RHE (overpotential of 54 mV) in EmimBF4/H2O (1:1) solution. The calculated work functions of the four catalysts decrease in the following sequence: MoS2 > WS2 > MoSe2 > WSe2, in agreement with experimental results.
In addition to 2D transition metal dichalcogenides, other 2D metal sulfides, such as SnS2, also promote efficient electrochemical reduction of CO2. Zheng et al. prepared SnS2 nanosheets by an atomic layer deposition method[111]. Using over 2D SnS2, the current density and FEformate at -0.75 V vs. RHE are
Transition metal dichalcogenides present a typical sandwich structure in which the metal atomic layer is sandwiched between two layers of sulfur atoms. Although the van der Waals force between each layer is very weak, strong covalent bonds in the plane ensure the stability of the 2D nanostructure. Therefore, 2D transition metal dichalcogenides exhibit excellent stability for long-term CO2 electro-reduction.
2D carbon-based materials
Carbon-based materials represent a class of submetallic materials owing to the sp2 hybrid state and the existence of metallized electrons on the surface. In the past decade, carbon-based materials have received increasing attention for electrocatalysis owing to their active edges, large surface area, and high charge carrier mobility[149-151]. The ultrahigh surface area and high electron transport along the carbon base plane make 2D carbon-based materials suitable for the electrochemical reduction of CO2.
Graphene is a representative of the carbon family and has been extensively explored in different research fields. Neither pure graphene nor graphene oxide (GO) is active in the electrochemical reduction of CO2 because the π-π framework cannot effectively activate CO2[152]. The electronic structure, physical structure, and morphology of graphene materials can be adjusted by heteroatom doping[153-155]. N-doped carbon has been widely studied because N is more electronegative than C. Four types of N exist in N-doped graphene: basal plane quaternary N, edge pyrrolic N, pyridinic N, and nitrilic N. Wang et al. prepared a nitrogen-doped graphene by high-temperature pyrolysis[110]. The nitrogen-doped graphene shows high catalytic activity in the electrochemical conversion of CO2 to formate, leading to an FEformate of 73%. Recently, many groups have reported that N-doped carbon materials can promote the conversion of CO2 to C2 products. For example, Song et al. developed a novel method to synthesize nitrogen-doped mesoporous carbon using a copolymer as the template[156] [Figure 10A]. Pyridinic N sites in this material facilitate the formation of
Figure 10. Electrochemical reduction of CO2 over 2D carbon-based materials. (A) TEM images of nitrogen-doped mesoporous carbons. (B) FEs of various products vs. potential over nitrogen-doped mesoporous carbon electrodes. (C) Schematic illustration of CO2 electro-reduction over nitrogen-doped mesoporous carbon electrodes. (D) DFT calculations of the electro-reduction of CO2 to ethanol over different pyrrolic N sites[156]. (E) Free-energy diagrams of CO2 electro-reduction over different N species[157]. (F) Magnified high-angle annular dark-field scanning transmission electron microscopy image of In/NC. (G) Fourier transform of In K-edge extended X-ray absorption fine structure spectra of different In-based catalysts. (H) Long-term stability of CO2 electro-reduction over In/NC[160]. 2D: 2-dimensional; TEM: transmission electron microscopy; FE: Faradaic efficiency; DFT: density functional theory.
N-doped carbon hybrid nanosheets, normally prepared by wet chemical synthesis, have the advantages of feasible operation, low cost, and large-scale synthesis, and thus they are widely employed as electrodes for the electro-reduction of CO2. Genovese et al. demonstrated that iron (III) oxyhydroxide on nitrogen-doped graphene exhibits an FECH3COOH of 61% at -0.5 V vs. Ag/AgCl[158]. The N-doped graphene not only boosts the activation of CO2 but also stabilizes Fe(II) species to suppress HER. Lu et al. reported that pyridinic N-rich carbon layers encapsulating Ni nanoparticles prepared by a hydrothermal method and pyrolysis exhibit a high FECO of 95% and long-term stability of 92 h at -1.05 V vs. RHE[159]. The excellent catalytic performance is attributed to the core-shell structure, which promotes mass transfer and the synergistic effect of N-C and metal oxides, while pyridinic-N increases the CO2 adsorption capacity and decreases the reaction energy barrier for *COOH formation, the rate-determining step.
Single-atom loaded carbon-based materials are also attractive for CO2 electro-reduction. Guo et al. reported that atomic indium on carbon (In/NC) exhibits a current density of 39.4 mA cm-2 and FECO of 97.2% in
2D carbon materials, such as graphene, have unique electronic properties, including the ambipolar electric field effect and quantum Hall effect. These unique electronic properties enable carbon materials to exhibit metal-like behaviors, which is beneficial for CO2 electro-reduction. Moreover, 2D carbon materials are often used as substrates for doping or decoration, broadening their utilizations in CO2 electro-reduction. Nevertheless, developing highly efficient 2D carbon based materials for CO2 electro-reduction still has a long way to go.
Other 2D materials
In addition to traditional 2D materials, many other 2D nanostructures are potential candidates to realize highly efficient electro-conversion of CO2 to valuable products. Recently, a series of novel 2D materials was applied to the electrochemical reduction of CO2 to various products, and their special structure determines the catalytic performance.
MOFs have attracted considerable attention in the field of catalysis and energy conversion owing to their rich active sites, diverse structure, and controllable morphology. Kang et al. designed and synthesized a highly efficient MOF catalyst [Cu2(L)-e/Cu] by in situ growth on Cu-foam substrates in IL-based solutions[162]. MOF particles with assemblies of approximately 50 nm on the surface of the Cu-foam form a thin film [Figure 11A-D]. The MOF thin film contains 15.3% of uncoupled Cu(II) sites, which are considered active sites for the electro-reduction of CO2 to formic acid. The as-prepared catalyst achieves a high current density of 65.8 mA cm2 and FEformate of 90.5% at -1.8 V vs. Ag/Ag+ in an IL-based electrolyte
Figure 11. Electrochemical reduction of CO2 over other 2D materials. (A) Photograph of Cu2(L)-e/Cu. (B) SEM image of Cu2(L)-e/Cu. (C and D) Views of the crystal structure of Cu2(L). (E) Plot of current density vs. potential over Cu2(L)-e/Cu. (F) Plot of FEHCOOH vs. potential over Cu2(L)-e/Cu[162]. (G) Schematic illustration of the heterogenization of molecular complexes on the Cu surface.
With their specific layered structure, 2D transition metal carbides and nitrides (MXenes) show high conductivity, high chemical stability, and multiple catalytic sites. Li et al. calculated the catalytic activity of single-component MXenes by DFT, and the results showed that Cr3C2Tx and Mo3C2Tx are the best candidates for the electrochemical reduction of CO2 to CH4[163]. These two materials favor the activation of CO2 rather than H2O, resulting in highly efficient CO2 electro-reduction and HER suppression. Qu et al. prepared N-doped Ti3C2 MXene nanosheets with abundant titanium vacancies (VTi) by a facile NH3-etching pyrolysis approach to achieve an FECO of 92% and current density of 10 mA cm-2 at -0.7 V vs. RHE in seawater as the electrolyte[164]. The coexistence of N and VTi modulates the electronic structure, leading to a decrease in the reaction energy barriers for *COOH formation.
Molecule-metal catalysts are molecular adsorbates that can accumulate intermediates during CO2 electro-reduction. Li et al. immobilized FeTPP[Cl] onto a Cu substrate to produce a molecular metal catalyst
Substrates functionalized by molecular monolayers are considered a type of 2D electrodes, which have been extensively studied for CO2 electro-reduction[166-169]. For instance, Fang et al. reported that a Au electrode modified with 4-pyridinylethanemercaptan(4-PEM) exhibits high CO2 selectivity toward formate compared with pristine Au electrode[170]. The high surface concentration of H+ impedes the first electron transfer to CO2•−. Moreover, the organometallic complex was also modified as a gas diffusion layer to fabricate a 2D electrode, which was employed as a cathode in a flow cell microreactor for CO2 electro-reduction. The electrode exhibits an FEHCOOH of 76% and FECO of 10%[171].
Covalent organic frameworks (COFs), composed of organic monomers and hybrid atoms, are new porous organic materials with high crystallinity. Covalent bonds are formed through reversible chemical reactions. COFs have gradually been applied to CO2 electrocatalysis owing to their designable structure, low density, adjustable pore structure, and easily modified structure. Bandomo et al. synthesized MnI tricarbonyl-based 2D COFs for CO2 electro-reduction[172]. The as-prepared 2D COFs exhibit a low onset potential of 190 mV, at which a current density of 12 mA cm-2 and FECO of 72% are achieved, and the catalyst remains active even after 16 h. Zhu et al. developed metalloporphyrin-tetrathiafulvalene-based COFs (M-TTCOFs), which have impressive catalytic performance, achieving an FECO of > 90% and high cycling stability (> 40 h)[173]. Tetrathiafulvalene in the 2D nanostructure serves as an electron donor to construct an efficient pathway to accelerate electron transfer and obtain a low activation energy for CO2 reduction.
To understand the catalytic nature of 2D materials, the electrochemical properties of the same material with various nanostructures are summarized in Table 1. In general, 2D catalysts exhibit excellent catalytic performance compared with the corresponding bulk catalysts.
SUMMARY AND OUTLOOK
This review summarizes the progress on various 2D materials with a focus on preparation methods and applications to CO2 electro-reduction. Without any doubt, advances in the synthesis of 2D materials over the past decade have produced 2D materials that markedly enhance the efficiency of CO2 electro-reduction.
Methods based on different principles for the preparation of 2D nanostructures have been reviewed. Wet-chemical synthesis and CVD are still the dominant methods for the mass production of 2D materials. It remains urgent to understand how to control the number of layers, flake dimensions, defect levels, and yield of 2D materials. Surface decoration is very important to broaden the application of 2D materials, and it is also an effective way to realize high catalytic performance for CO2 electro-reduction. Selecting the electrode material is the first step of catalysis. The distribution and production rate of products are determined by the thermodynamic and kinetic energy barriers of reaction pathways. In principle, the electron distribution and the charge density of the catalyst surface affect the kinetic energy barriers. Therefore, selecting electrode materials with appropriate electronic properties can enhance the efficiency of CO2 electroreduction.
Selectivity is an essential evaluation indicator of the catalytic performance of 2D materials. Although great progress has been made, the reaction pathway determined by the 2D structure is still unclear. In other words, catalytic performance as a function of structural parameters remains unexplored. Strategies such as morphology modification, doping, and surface decoration have been developed to achieve high catalytic performance of 2D materials. Nevertheless, designing in situ characterization techniques and developing theoretical calculations to unveil the effect of the structure of 2D materials on CO2 electro-reduction are highly significant.
Nowadays, high current density can be easily realized by potential increase, structure design, surface functionalization, and cell modification. However, high potentials require high energy consumption, which is inconsistent with the concept of sustainable development. High potentials also influence the long-term stability and promote HER. The surface structure and activity of 2D materials also change under extremely negative potentials. Additionally, most cases of CO2 electro-reduction over 2D materials are currently being investigated in laboratories owing to the high cost, difficulties in scale-up, and uncontrollable operation. Large-scale synthesis of 2D materials and the preparation of stable and large 2D material-based electrodes are the first important steps into industrialization. We believe that 2D nanostructures will gradually prevail in CO2 electro-reduction and contribute to carbon neutrality worldwide.
DECLARATIONS
Authors’ contributionsPrepared the manuscript: Yin Y
Performed manuscript correcting: Kang X, Han B
Availability of data and materialsNot applicable.
Financial support and sponsorshipThis work is supported by National Natural Science Foundation of China (22273108, 22073104) and Beijing Natural Science Foundation (2222043).
Conflict of interestAll authors declared that there are no conflicts of interest.
Ethical approval and consent to participateNot applicable.
Consent for publicationNot applicable.
Copyright© The Author(s) 2022.
REFERENCES
1. He M, Sun Y, Han B. Green carbon science: efficient carbon resource processing, utilization, and recycling towards carbon neutrality. Angew Chem Int Ed Engl 2022;61:e202112835.
2. Mehla S, Kandjani AE, Babarao R, et al. Porous crystalline frameworks for thermocatalytic CO2 reduction: an emerging paradigm. Energy Environ Sci 2021;14:320-52.
3. Wu Y, Jiang Z, Lu X, Liang Y, Wang H. Domino electroreduction of CO2 to methanol on a molecular catalyst. Nature 2019;575:639-42.
4. Yang HB, Hung S, Liu S, et al. Atomically dispersed Ni(I) as the active site for electrochemical CO2 reduction. Nat Energy 2018;3:140-7.
5. Li CW, Ciston J, Kanan MW. Electroreduction of carbon monoxide to liquid fuel on oxide-derived nanocrystalline copper. Nature 2014;508:504-7.
6. Li L, Li X, Sun Y, Xie Y. Rational design of electrocatalytic carbon dioxide reduction for a zero-carbon network. Chem Soc Rev 2022;51:1234-52.
7. Zhang J, An B, Li Z, et al. Neighboring Zn-Zr sites in a metal-organic framework for CO2 hydrogenation. J Am Chem Soc 2021;143:8829-37.
8. Koshy DM, Nathan SS, Asundi AS, et al. Bridging thermal catalysis and electrocatalysis: catalyzing CO2 conversion with carbon-based materials. Angew Chem Int Ed Engl 2021;60:17472-80.
9. Sun Q, Wang N, Yu J. Advances in catalytic applications of zeolite-supported metal catalysts. Adv Mater 2021;33:e2104442.
10. Rao H, Schmidt LC, Bonin J, Robert M. Visible-light-driven methane formation from CO2 with a molecular iron catalyst. Nature 2017;548:74-7.
11. Barman S, Singh A, Rahimi FA, Maji TK. Metal-free catalysis: a redox-active donor-acceptor conjugated microporous polymer for selective visible-light-driven CO2 reduction to CH4. J Am Chem Soc 2021;143:16284-92.
12. Zhao W, Zhai D, Liu C, et al. Unblocked intramolecular charge transfer for enhanced CO2 photoreduction enabled by an imidazolium-based ionic conjugated microporous polymer. Appl Catal B-environ 2022;300:120719.
13. Ozden A, Wang Y, Li F, et al. Cascade CO2 electroreduction enables efficient carbonate-free production of ethylene. Joule 2021;5:706-19.
14. Qiu XF, Zhu HL, Huang JR, Liao PQ, Chen XM. Highly selective CO2 electroreduction to C2H4 using a metal-organic framework with dual active sites. J Am Chem Soc 2021;143:7242-6.
15. Ma W, Xie S, Liu T, et al. Electrocatalytic reduction of CO2 to ethylene and ethanol through hydrogen-assisted C-C coupling over fluorine-modified copper. Nat Catal 2020;3:478-87.
16. Cheng Y, Hou P, Wang X, Kang P. CO2 electrolysis system under industrially relevant conditions. Acc Chem Res 2022;55:231-40.
17. Gao D, Wei P, Li H, Lin L, Wang G, Bao X. Designing electrolyzers for electrocatalytic CO2 reduction. Acta Phys-Chim Sin 2021;37:2009021.
18. Li N, Si D, Wu Q, Wu Q, Huang Y, Cao R. Boosting electrocatalytic CO2 reduction with conjugated bimetallic CO/Zn polyphthalocyanine frameworks. CCS Chem 2022.
19. Jia S, Ma X, Sun X, Han B. Electrochemical transformation of CO2 to value-added chemicals and fuels. CCS Chem 2022;4:3213-29.
20. Cheng Y, Hou P, Pan H, Shi H, Kang P. Selective electrocatalytic reduction of carbon dioxide to oxalate by lead tin oxides with low overpotential. Appl Catal B-environ 2020;272:118954.
21. Yang D, Zhu Q, Han B. Electroreduction of CO2 in ionic liquid-based electrolytes. Innovation 2020;1:100016.
22. Schwarz HA, Dodson RW. Reduction potentials of CO2- and the alcohol radicals. J Phys Chem 1989;93:409-14.
23. Zhao K, Quan X. Carbon-based materials for electrochemical reduction of CO2 to C2+ oxygenates: recent progress and remaining challenges. ACS Catal 2021;11:2076-97.
24. Cheng Y, Hou J, Kang P. Integrated capture and electroreduction of flue gas CO2 to formate using amine functionalized SnOx nanoparticles. ACS Energy Lett 2021;6:3352-8.
25. Chang C, Chen W, Chen Y, et al. Recent progress on two-dimensional materials. Acta Phys-Chim Sin 2021;37:2108017.
26. Fu Q, Liu H, Tang X, Wang R, Chen M, Liu Y. Multifunctional two-dimensional polymers for perovskite solar cells with efficiency exceeding 24%. ACS Energy Lett 2022;7:1128-36.
27. Song D, Chen X, Lin Z, et al. Usability identification framework and high-throughput screening of two-dimensional materials in lithium ion batteries. ACS Nano 2021;15:16469-77.
28. Wang J, Malgras V, Sugahara Y, Yamauchi Y. Electrochemical energy storage performance of 2D nanoarchitectured hybrid materials. Nat Commun 2021;12:3563.
29. Liang Q, Zhang Q, Zhao X, Liu M, Wee ATS. Defect engineering of two-dimensional transition-metal dichalcogenides: applications, challenges, and opportunities. ACS Nano 2021;15:2165-81.
30. Shamzhy M, Gil B, Opanasenko M, Roth WJ, Čejka J. MWW and MFI frameworks as model layered zeolites: structures, transformations, properties, and activity. ACS Catal 2021;11:2366-96.
31. Xu L, Ma T, Shen Y, et al. Rational manipulation of stacking arrangements in three-dimensional zeolites built from two-dimensional zeolitic nanosheets. Angew Chem Int Ed Engl 2020;59:19934-9.
32. Li G, Shen Y, Zhao S, et al. Construction of rGO-SnO2 heterojunction for enhanced hydrogen detection. Appl Surf Sci 2022;585:152623.
33. Li S, Thiering G, Udvarhelyi P, Ivády V, Gali A. Carbon defect qubit in two-dimensional WS2. Nat Commun 2022;13:1210.
34. Kovalska E, Antonatos N, Luxa J, Sofer Z. Edge-hydrogenated germanene by electrochemical decalcification-exfoliation of CaGe2: Germanene-enabled vapor sensor. ACS Nano 2021;15:16709-18.
35. Maiti R, Patil C, Saadi MASR, et al. Strain-engineered high-responsivity MoTe2 photodetector for silicon photonic integrated circuits. Nat Photonics 2020;14:578-84.
36. Liu R, Wang F, Liu L, et al. Band alignment engineering in two-dimensional transition metal dichalcogenide-based heterostructures for photodetectors. Small Structures 2021;2:2000136.
37. An C, Nie F, Zhang R, et al. Two-dimensional material-enhanced flexible and self-healable photodetector for large-area photodetection. Adv Funct Mater 2021;31:2100136.
38. Wang K, Chen J, Yan X. MXene Ti3C2 memristor for neuromorphic behavior and decimal arithmetic operation applications. Nano Energy 2021;79:105453.
39. Cao G, Gao C, Wang J, Lan J, Yan X. Memristor based on two-dimensional titania nanosheets for multi-level storage and information processing. Nano Res 2022;15:8419-27.
40. Tang B, Veluri H, Li Y, et al. Wafer-scale solution-processed 2D material analog resistive memory array for memory-based computing. Nat Commun 2022;13:3037.
41. Nguyen TN, Salehi M, Le QV, Seifitokaldani A, Dinh CT. Fundamentals of electrochemical CO2 reduction on single-metal-atom catalysts. ACS Catal 2020;10:10068-95.
42. Wang Y, Liu J, Wang Y, Al-Enizi AM, Zheng G. Tuning of CO2 reduction selectivity on metal electrocatalysts. Small 2017;13:1701809.
43. Shimoni R, Shi Z, Binyamin S, et al. Electrostatic secondary-sphere interactions that facilitate rapid and selective electrocatalytic CO2 reduction in a fe-porphyrin-based metal-organic framework. Angew Chem Int Ed Engl 2022;61:e202206085.
44. Zhou P, Shen Y, Zhao S, et al. Hydrothermal synthesis of novel ternary hierarchical MoS2/TiO2/clinoptilolite nanocomposites with remarkably enhanced visible light response towards xanthates. Appl Surf Sci 2021;542:148578.
45. Sun Z, Ma T, Tao H, Fan Q, Han B. Fundamentals and challenges of electrochemical CO2 reduction using two-dimensional materials. Chem 2017;3:560-87.
46. Fang W, Huang L, Zaman S, Wang Z, Han Y, Xia BY. Recent progress on two-dimensional electrocatalysis. Chem Res Chin Univ 2020;36:611-21.
47. Li Z, Zhai L, Ge Y, et al. Wet-chemical synthesis of two-dimensional metal nanomaterials for electrocatalysis. Natl Sci Rev 2022;9:nwab142.
48. Ma Y, Shi R, Zhang T. Research progress on triphase interface electrocatalytic carbon dioxide reduction. Acta Chimica Sinica 2021;79:369.
49. Pan F, Yang Y. Designing CO2 reduction electrode materials by morphology and interface engineering. Energy Environ Sci 2020;13:2275-309.
50. Gu H, Zhong L, Shi G, et al. Graphdiyne/Graphene heterostructure: a universal 2D scaffold anchoring monodispersed transition-metal phthalocyanines for selective and durable CO2 electroreduction. J Am Chem Soc 2021;143:8679-88.
51. Chen S, Kang Z, Hu X, et al. Delocalized spin states in 2D atomic layers realizing enhanced electrocatalytic oxygen evolution. Adv Mater 2017;29:1701687.
52. Wang P, Zhao D, Yin L. Two-dimensional matrices confining metal single atoms with enhanced electrochemical reaction kinetics for energy storage applications. Energy Environ Sci 2021;14:1794-834.
53. Li X, Wang S, Li L, Zu X, Sun Y, Xie Y. Opportunity of atomically thin two-dimensional catalysts for promoting CO2 electroreduction. Acc Chem Res 2020;53:2964-74.
54. Rong X, Wang HJ, Lu XL, Si R, Lu TB. Controlled synthesis of a vacancy-defect single-atom catalyst for boosting CO2 electroreduction. Angew Chem Int Ed Engl 2020;59:1961-5.
55. Pan J, Sun Y, Deng P, et al. Hierarchical and ultrathin copper nanosheets synthesized via galvanic replacement for selective electrocatalytic carbon dioxide conversion to carbon monoxide. Appl Catal B-environ 2019;255:117736.
56. Chia X, Pumera M. Characteristics and performance of two-dimensional materials for electrocatalysis. Nat Catal 2018;1:909-21.
57. Li Y, Chen J, Chen S, et al. In situ confined growth of bismuth nanoribbons with active and robust edge sites for boosted CO2 electroreduction. ACS Energy Lett 2022;7:1454-61.
58. Liu W, Qi J, Bai P, Zhang W, Xu L. Utilizing spatial confinement effect of N atoms in micropores of coal-based metal-free material for efficiently electrochemical reduction of carbon dioxide. Appl Catal B-environ 2020;272:118974.
59. Niu ZZ, Gao FY, Zhang XL, et al. Hierarchical copper with inherent hydrophobicity mitigates electrode flooding for high-rate CO2 Electroreduction to multicarbon products. J Am Chem Soc 2021;143:8011-21.
60. Lv K, Teng C, Shi M, et al. Hydrophobic and electronic properties of the E-MoS2 nanosheets induced by FAS for the CO2 electroreduction to syngas with a wide range of CO/H2 ratios. Adv Funct Mater 2018;28:1802339.
61. Zeng L, You C, Hong N, Zhang X, Liang T. Large-scale preparation of 2D metal films by a top-down approach. Adv Eng Mater 2020;22:1901359.
62. Xu Y, Sprick RS, Brownbill NJ, et al. Bottom-up wet-chemical synthesis of a two-dimensional porous carbon material with high supercapacitance using a cascade coupling/cyclization route. J Mater Chem A 2021;9:3303-8.
63. Watts MC, Picco L, Russell-Pavier FS, et al. Production of phosphorene nanoribbons. Nature 2019;568:216-20.
64. Novoselov KS, Geim AK, Morozov SV, et al. Electric field effect in atomically thin carbon films. Science 2004;306:666-9.
65. Li H, Lu G, Wang Y, et al. Mechanical exfoliation and characterization of single- and few-layer nanosheets of WSe2, TaS2, and TaSe2. Small 2013;9:1974-81.
66. Huang Y, Pan YH, Yang R, et al. Universal mechanical exfoliation of large-area 2D crystals. Nat Commun 2020;11:2453.
67. Coleman JN, Lotya M, O’Neill A, et al. Two-dimensional nanosheets produced by liquid exfoliation of layered materials. Science 2011;331:568-71.
68. Ma R, Sasaki T. Two-dimensional oxide and hydroxide nanosheets: controllable high-quality exfoliation, molecular assembly, and exploration of functionality. Acc Chem Res 2015;48:136-43.
69. Dakhchoune M, Villalobos LF, Semino R, et al. Gas-sieving zeolitic membranes fabricated by condensation of precursor nanosheets. Nat Mater 2021;20:362-9.
70. Obst M, Arnauts G, Cruz AJ, et al. Chemical vapor deposition of ionic liquids for the fabrication of ionogel films and patterns. Angew Chem Int Ed Engl 2021;60:25668-73.
71. Novoselov KS, Mishchenko A, Carvalho A, Castro Neto AH. 2D materials and van der Waals heterostructures. Science 2016;353:aac9439.
72. Kim KS, Zhao Y, Jang H, et al. Large-scale pattern growth of graphene films for stretchable transparent electrodes. Nature 2009;457:706-10.
73. Li X, Cai W, An J, et al. Large-area synthesis of high-quality and uniform graphene films on copper foils. Science 2009;324:1312-4.
74. Browne MP, Novotný F, Manzanares Palenzuela CL, Šturala J, Sofer Z, Pumera M. 2H and 2H/1T-transition metal dichalcogenide films prepared via powderless gas deposition for the hydrogen evolution reaction. ACS Sustainable Chem Eng 2019;7:16440-9.
75. Yin C, Gong C, Chu J, et al. Ultrabroadband photodetectors up to 10.6 µm based on 2D Fe3O4 nanosheets. Adv Mater 2020;32:e2002237.
76. Zhou J, Lin J, Huang X, et al. A library of atomically thin metal chalcogenides. Nature 2018;556:355-9.
77. Li W, Qiu X, Lv B, et al. Free-standing 2D ironene with magnetic vortex structure at room temperature. Matter 2022;5:291-301.
78. Xu T, Li S, Li A, et al. Structural evolution of atomically thin 1T’-MoTe2 alloyed in chalcogen atmosphere. Small Struct 2022;3:2200025.
79. Sun Z, Liao T, Dou Y, et al. Generalized self-assembly of scalable two-dimensional transition metal oxide nanosheets. Nat Commun 2014;5:3813.
80. Peng Y, Tan Q, Huang H, et al. Customization of functional MOFs by a modular design strategy for target applications. Chem Synth 2022;2:15.
81. Wang L, Saji SE, Wu L, et al. Emerging synthesis strategies of 2D MOFs for electrical devices and integrated circuits. Small 2022;18:e2201642.
82. Pham HTB, Choi JY, Huang S, et al. Imparting functionality and enhanced surface area to a 2D electrically conductive MOF via macrocyclic linker. J Am Chem Soc 2022;144:10615-21.
83. Zheng Y, Zheng S, Xu Y, Xue H, Liu C, Pang H. Ultrathin two-dimensional cobalt-organic frameworks nanosheets for electrochemical energy storage. Chem Eng J 2019;373:1319-28.
84. Sun X, Lu L, Zhu Q, et al. MoP nanoparticles supported on indium-doped porous carbon: outstanding catalysts for highly efficient CO2 electroreduction. Angew Chem Int Ed Engl 2018;57:2427-31.
85. Sun X, Zhu Q, Kang X, et al. Molybdenum-bismuth bimetallic chalcogenide nanosheets for highly efficient electrocatalytic reduction of carbon dioxide to methanol. Angew Chem Int Ed Engl 2016;55:6771-5.
86. Lu L, Guo W, Chen C, et al. Synthesis of Sn4P3/reduced graphene oxide nanocomposites as highly efficient electrocatalysts for CO2 reduction. Green Chem 2020;22:6804-8.
87. Rabiee H, Ge L, Zhang X, et al. Shape-tuned electrodeposition of bismuth-based nanosheets on flow-through hollow fiber gas diffusion electrode for high-efficiency CO2 reduction to formate. Appl Catal B-environ 2021;286:119945.
88. Abdelazim NM, Noori YJ, Thomas S, et al. Lateral growth of MoS2 2D material semiconductors over an insulator via electrodeposition. Adv Electron Mater 2021;7:2100419.
89. Feng C, Wang F, Liu Z, et al. A self-healing catalyst for electrocatalytic and photoelectrochemical oxygen evolution in highly alkaline conditions. Nat Commun 2021;12:5980.
90. Shen L, Zhang Q, Luo J, et al. Heteroatoms adjusting amorphous FeMn-based nanosheets via a facile electrodeposition method for full water splitting. ACS Sustainable Chem Eng 2021;9:5963-71.
91. Tan SF, Reidy K, Lee S, et al. Multilayer graphene - a promising electrode material in liquid cell electrochemistry. Adv Funct Materials 2021;31:2104628.
92. Lukatskaya MR, Mashtalir O, Ren CE, et al. Cation intercalation and high volumetric capacitance of two-dimensional titanium carbide. Science 2013;341:1502-5.
93. Lukatskaya MR, Halim J, Dyatkin B, et al. Room-temperature carbide-derived carbon synthesis by electrochemical etching of MAX phases. Angew Chem Int Ed Engl 2014;53:4877-80.
94. Ghidiu M, Lukatskaya MR, Zhao MQ, Gogotsi Y, Barsoum MW. Conductive two-dimensional titanium carbide “clay” with high volumetric capacitance. Nature 2014;516:78-81.
95. Liu L, Yang X, Xie Y, et al. A universal lab-on-salt-particle approach to 2D single-layer ordered mesoporous materials. Adv Mater 2020;32:e1906653.
96. Zhang F, Zhang J, Zhang B, et al. CO2 controls the oriented growth of metal-organic framework with highly accessible active sites. Nat Commun 2020;11:1431.
97. Ko KY, Song JG, Kim Y, et al. Improvement of gas-sensing performance of large-area tungsten disulfide nanosheets by surface functionalization. ACS Nano 2016;10:9287-96.
98. Lee C, Zhao Y, Wang C, Mitchell DRG, Wallace GG. Rapid formation of self-organised Ag nanosheets with high efficiency and selectivity in CO2 electroreduction to CO. Sustain Energy Fuels 2017;1:1023-7.
99. Zhang T, Li X, Qiu Y, et al. Multilayered Zn nanosheets as an electrocatalyst for efficient electrochemical reduction of CO2. J Catal 2018;357:154-62.
100. Lei F, Liu W, Sun Y, et al. Metallic tin quantum sheets confined in graphene toward high-efficiency carbon dioxide electroreduction. Nat Commun 2016;7:12697.
101. Zhang B, Zhang J, Hua M, et al. Highly electrocatalytic ethylene production from CO2 on nanodefective Cu nanosheets. J Am Chem Soc 2020;142:13606-13.
102. Han N, Wang Y, Deng J, et al. Self-templated synthesis of hierarchical mesoporous SnO2 nanosheets for selective CO2 reduction. J Mater Chem A 2019;7:1267-72.
103. Liu W, Zhai P, Li A, et al. Electrochemical CO2 reduction to ethylene by ultrathin CuO nanoplate arrays. Nat Commun 2022;13:1877.
104. Liu K, Wang J, Shi M, Yan J, Jiang Q. Simultaneous achieving of high faradaic efficiency and CO Partial current density for CO2 reduction via robust, noble-metal-free Zn nanosheets with favorable adsorption energy. Adv Energy Mater 2019;9:1900276.
105. Gao S, Lin Y, Jiao X, et al. Partially oxidized atomic cobalt layers for carbon dioxide electroreduction to liquid fuel. Nature 2016;529:68-71.
106. Gao S, Jiao X, Sun Z, et al. Ultrathin CO3O4 layers realizing optimized CO2 electroreduction to formate. Angew Chem Int Ed Engl 2016;55:698-702.
107. Han N, Wang Y, Yang H, et al. Ultrathin bismuth nanosheets from in situ topotactic transformation for selective electrocatalytic CO2 reduction to formate. Nat Commun 2018;9:1320.
108. Geng Z, Kong X, Chen W, et al. Oxygen vacancies in ZnO nanosheets enhance CO2 electrochemical reduction to CO. Angew Chem Int Ed Engl 2018;57:6054-9.
109. Chu M, Chen C, Guo W, et al. Enhancing electroreduction of CO2 over Bi2 WO6 nanosheets by oxygen vacancies. Green Chem 2019;21:2589-93.
110. Wang H, Chen Y, Hou X, Ma C, Tan T. Nitrogen-doped graphenes as efficient electrocatalysts for the selective reduction of carbon dioxide to formate in aqueous solution. Green Chem 2016;18:3250-6.
111. Zheng X, De Luna P, García de Arquer FP, et al. Sulfur-modulated tin sites enable highly selective electrochemical reduction of CO2 to formate. Joule 2017;1:794-805.
112. Zhang A, Liang Y, Li H, et al. In-situ surface reconstruction of InN nanosheets for efficient CO2 electroreduction into formate. Nano Lett 2020;20:8229-35.
113. Li F, Li YC, Wang Z, et al. Cooperative CO2-to-ethanol conversion via enriched intermediates at molecule-metal catalyst interfaces. Nat Catal 2020;3:75-82.
114. Yu Y, Lee SJ, Theerthagiri J, et al. Reconciling of experimental and theoretical insights on the electroactive behavior of C/Ni nanoparticles with AuPt alloys for hydrogen evolution efficiency and non-enzymatic sensor. Chem Eng J 2022;435:134790.
115. Yu Y, Lee SJ, Theerthagiri J, Lee Y, Choi MY. Architecting the AuPt alloys for hydrazine oxidation as an anolyte in fuel cell: comparative analysis of hydrazine splitting and water splitting for energy-saving H2 generation. Appl Catal B-environ 2022;316:121603.
116. Lu Q, Rosen J, Zhou Y, et al. A selective and efficient electrocatalyst for carbon dioxide reduction. Nat Commun 2014;5:3242.
117. Mistry H, Reske R, Zeng Z, et al. Exceptional size-dependent activity enhancement in the electroreduction of CO2 over Au nanoparticles. J Am Chem Soc 2014;136:16473-6.
118. Zhao Y, Tan X, Yang W, et al. Surface reconstruction of ultrathin palladium nanosheets during electrocatalytic CO2 reduction. Angew Chem Int Ed Engl 2020;59:21493-8.
119. Wang Z, Li C, Yamauchi Y. Nanostructured nonprecious metal catalysts for electrochemical reduction of carbon dioxide. Nano Today 2016;11:373-91.
120. Xiao J, Gao MR, Liu S, Luo JL. Hexagonal Zn nanoplates enclosed by Zn(100) and Zn(002) facets for highly selective CO2 electroreduction to CO. ACS Appl Mater Interfaces 2020;12:31431-8.
121. Han J, An P, Liu S, et al. Reordering d orbital energies of single-site catalysts for CO2 electroreduction. Angew Chem Int Ed Engl 2019;58:12711-6.
122. Yin J, Yin Z, Jin J, et al. A new hexagonal cobalt nanosheet catalyst for selective CO2 conversion to ethanal. J Am Chem Soc 2021;143:15335-43.
123. Yang J, Wang X, Qu Y, et al. Bi-based metal-organic framework derived leafy bismuth nanosheets for carbon dioxide electroreduction. Adv Energy Mater 2020;10:2001709.
124. Yang H, Han N, Deng J, et al. Selective CO2 reduction on 2D mesoporous Bi nanosheets. Adv Energy Mater 2018;8:1801536.
125. Wu J, Sharma PP, Harris BH, Zhou X. Electrochemical reduction of carbon dioxide: IV dependence of the Faradaic efficiency and current density on the microstructure and thickness of tin electrode. J Power Sources 2014;258:189-94.
126. Wu D, Wang X, Fu X, Luo J. Ultrasmall Bi nanoparticles confined in carbon nanosheets as highly active and durable catalysts for CO2 electroreduction. Appl Catal B-environ 2021;284:119723.
127. Shifa TA, Vomiero A. Confined catalysis: progress and prospects in energy conversion. Adv Energy Mater 2019;9:1902307.
128. Xiao C, Zhang J. Architectural design for enhanced C2 product selectivity in electrochemical CO2 reduction using Cu-based catalysts: a review. ACS Nano 2021;15:7975-8000.
129. Zhang Z, Bian L, Tian H, et al. Tailoring the surface and interface structures of copper-based catalysts for electrochemical reduction of CO2 to ethylene and ethanol. Small 2022;18:e2107450.
130. Hori Y, Murata A, Takahashi R. Formation of hydrocarbons in the electrochemical reduction of carbon dioxide at a copper electrode in aqueous solution. J Chem Soc, Faraday Trans 1 1989;85:2309.
131. Chen C, Sun X, Yan X, et al. A strategy to control the grain boundary density and Cu+/Cu0 ratio of Cu-based catalysts for efficient electroreduction of CO2 to C2 products. Green Chem 2020;22:1572-6.
132. Inoue T, Fujishima A, Konishi S, Honda K. Photoelectrocatalytic reduction of carbon dioxide in aqueous suspensions of semiconductor powders. Nature 1979;277:637-8.
133. Han Z, Han D, Chen Z, et al. Steering surface reconstruction of copper with electrolyte additives for CO2 electroreduction. Nat Commun 2022;13:3158.
134. Sang J, Wei P, Liu T, et al. A reconstructed Cu2P2O7 catalyst for selective CO2 electroreduction to multicarbon products. Angew Chem Int Ed Engl 2022;61:e202114238.
135. Xiang Q, Li F, Wang J, et al. Heterostructure of ZnO nanosheets/Zn with a highly enhanced edge surface for efficient CO2 electrochemical reduction to CO. ACS Appl Mater Interfaces 2021;13:10837-44.
136. Sikam P, Takahashi K, Roongcharoen T, et al. Effect of 3d-transition metals doped in ZnO monolayers on the CO2 electrochemical reduction to valuable products: first principles study. Appl Surf Sci 2021;550:149380.
137. Luo W, Zhang Q, Zhang J, Moioli E, Zhao K, Züttel A. Electrochemical reconstruction of ZnO for selective reduction of CO2 to CO. Appl Catal B-environ 2020;273:119060.
138. Gao S, Sun Z, Liu W, et al. Atomic layer confined vacancies for atomic-level insights into carbon dioxide electroreduction. Nat Commun 2017;8:14503.
139. Cheng D, Zhao ZJ, Zhang G, et al. The nature of active sites for carbon dioxide electroreduction over oxide-derived copper catalysts. Nat Commun 2021;12:395.
140. Yuan X, Chen S, Cheng D, et al. Controllable Cu0-Cu+ sites for electrocatalytic reduction of carbon dioxide. Angew Chem Int Ed Engl 2021;60:15344-7.
141. Li P, Bi J, Liu J, et al. In situ dual doping for constructing efficient CO2-to-methanol electrocatalysts. Nat Commun 2022;13:1965.
142. Duan J, Liu T, Zhao Y, et al. Active and conductive layer stacked superlattices for highly selective CO2 electroreduction. Nat Commun 2022;13:2039.
143. Zhao M, Gu Y, Chen P, et al. Highly selective electrochemical CO2 reduction to CO using a redox-active couple on low-crystallinity mesoporous ZnGa2O4 catalyst. J Mater Chem A 2019;7:9316-23.
144. Asadi M, Kumar B, Behranginia A, et al. Robust carbon dioxide reduction on molybdenum disulphide edges. Nat Commun 2014;5:4470.
145. Abbasi P, Asadi M, Liu C, et al. Tailoring the edge structure of molybdenum disulfide toward electrocatalytic reduction of carbon dioxide. ACS Nano 2017;11:453-60.
146. Mao X, Wang L, Xu Y, Li Y. Modulating the MoS2 edge structures by doping transition metals for electrocatalytic CO2 reduction. J Phys Chem C 2020;124:10523-9.
147. Asadi M, Kim K, Liu C, et al. Nanostructured transition metal dichalcogenide electrocatalysts for CO2 reduction in ionic liquid. Science 2016;353:467-70.
148. Zhang A, Liang Y, Li H, et al. Electronic tuning of SnS2 nanosheets by hydrogen incorporation for efficient CO2 electroreduction. Nano Lett 2021;21:7789-95.
149. Ma X, Du J, Sun H, et al. Boron, nitrogen co-doped carbon with abundant mesopores for efficient CO2 electroreduction. Appl Catal B-environ 2021;298:120543.
150. Tuci G, Rossin A, Zhang X, Pham-huu C, Giambastiani G. Exohedrally functionalized carbon-based networks as catalysts for electrochemical syntheses. Curr Opin Green Sustain Chem 2022;33:100579.
151. Zhang X, Xue D, Jiang S, et al. Rational confinement engineering of MOF-derived carbon-based electrocatalysts toward CO2 reduction and O2 reduction reactions. InfoMat 2022:4.
152. Hasani A, Teklagne MA, Do HH, et al. Graphene-based catalysts for electrochemical carbon dioxide reduction. Carbon Energy 2020;2:158-75.
153. Tao H, Gao Y, Talreja N, et al. Two-dimensional nanosheets for electrocatalysis in energy generation and conversion. J Mater Chem A 2017;5:7257-84.
154. Wang ZL, Choi J, Xu M, et al. Optimizing electron densities of Ni-N-C complexes by hybrid coordination for efficient electrocatalytic CO2 reduction. ChemSusChem 2020;13:929-37.
155. Lee SJ, Theerthagiri J, Nithyadharseni P, et al. Heteroatom-doped graphene-based materials for sustainable energy applications: a review. Renew Sust Energ Rev 2021;143:110849.
156. Song Y, Chen W, Zhao C, Li S, Wei W, Sun Y. Metal-free nitrogen-doped mesoporous carbon for electroreduction of CO2 to ethanol. Angew Chem Int Ed Engl 2017;56:10840-4.
157. Hao X, An X, Patil AM, et al. Biomass-derived N-doped carbon for efficient electrocatalytic CO2 reduction to CO and Zn-CO2 batteries. ACS Appl Mater Interfaces 2021;13:3738-47.
158. Genovese C, Schuster ME, Gibson EK, et al. Operando spectroscopy study of the carbon dioxide electro-reduction by iron species on nitrogen-doped carbon. Nat Commun 2018;9:935.
159. Lu Q, Chen C, Di Q, et al. Dual role of pyridinic-N doping in carbon-coated Ni nanoparticles for highly efficient electrochemical CO2 reduction to CO over a wide potential range. ACS Catal 2022;12:1364-74.
160. Guo W, Tan X, Bi J, et al. Atomic indium catalysts for switching CO2 electroreduction products from formate to CO. J Am Chem Soc 2021;143:6877-85.
161. Shi G, Xie Y, Du L, et al. Constructing Cu-C bonds in a graphdiyne-regulated Cu single-atom electrocatalyst for CO2 reduction to CH4. Angew Chem Int Ed Engl 2022;61:e202203569.
162. Kang X, Li L, Sheveleva A, et al. Electro-reduction of carbon dioxide at low over-potential at a metal-organic framework decorated cathode. Nat Commun 2020;11:5464.
163. Li N, Chen X, Ong WJ, et al. Understanding of electrochemical mechanisms for CO2 capture and conversion into hydrocarbon fuels in transition-metal carbides (MXenes). ACS Nano 2017;11:10825-33.
164. Qu D, Peng X, Mi Y, et al. Nitrogen doping and titanium vacancies synergistically promote CO2 fixation in seawater. Nanoscale 2020;12:17191-5.
165. Han Z, Kortlever R, Chen HY, Peters JC, Agapie T. CO2 reduction selective for C(≥ 2) products on polycrystalline copper with N-substituted pyridinium additives. ACS Cent Sci 2017;3:853-9.
166. Costentin C, Savéant JM. Molecular approach to catalysis of electrochemical reaction in porous films. Curr Opin Electrochem 2019;15:58-65.
167. Stuardi FM, Tiozzo A, Rotundo L, Leclaire J, Gobetto R, Nervi C. Efficient electrochemical reduction of CO2 to formate in methanol solutions by Mn-functionalized electrodes in the presence of amines. Chem Eur J 2022;28:e202104377.
168. McCarthy BD, Beiler AM, Johnson BA, Liseev T, Castner AT, Ott S. Analysis of electrocatalytic metal-organic frameworks. Coord Chem Rev 2020;406:213137.
169. Sun C, Gobetto R, Nervi C. Recent advances in catalytic CO2 reduction by organometal complexes anchored on modified electrodes. New J Chem 2016;40:5656-61.
170. Fang Y, Flake JC. Electrochemical reduction of CO2 at functionalized Au electrodes. J Am Chem Soc 2017;139:3399-405.
171. Filippi J, Rotundo L, Gobetto R, et al. Turning manganese into gold: efficient electrochemical CO2 reduction by a fac-Mn(apbpy)
172. Dubed Bandomo GC, Mondal SS, Franco F, et al. Mechanically constrained catalytic Mn(CO)3 Br single sites in a two-dimensional covalent organic framework for CO2 electroreduction in H2O. ACS Catal 2021;11:7210-22.
Cite This Article

How to Cite
Download Citation
Export Citation File:
Type of Import
Tips on Downloading Citation
Citation Manager File Format
Type of Import
Direct Import: When the Direct Import option is selected (the default state), a dialogue box will give you the option to Save or Open the downloaded citation data. Choosing Open will either launch your citation manager or give you a choice of applications with which to use the metadata. The Save option saves the file locally for later use.
Indirect Import: When the Indirect Import option is selected, the metadata is displayed and may be copied and pasted as needed.
About This Article
Copyright
Author Biographies
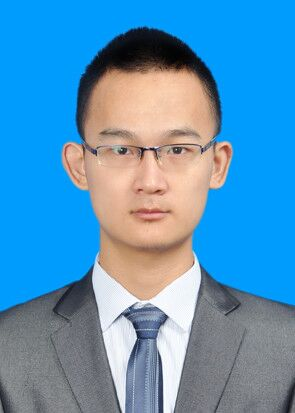
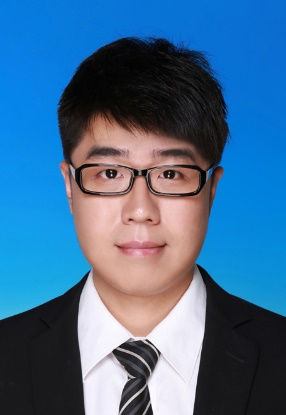
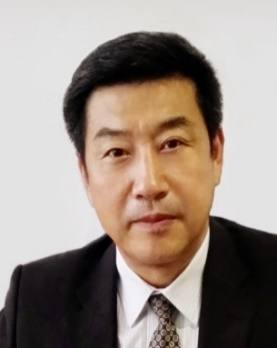
Comments
Comments must be written in English. Spam, offensive content, impersonation, and private information will not be permitted. If any comment is reported and identified as inappropriate content by OAE staff, the comment will be removed without notice. If you have any queries or need any help, please contact us at [email protected].