Recent advances in circularly polarized luminescence generated by inorganic materials
Abstract
Circularly polarized luminescence (CPL) is an interesting phenomenon that represents the unequal emission of left- and right-handed polarized light from an emitter. CPL is promising in chirality characterization and various optical applications. Traditionally, research on CPL has been centered on organic substances. Nevertheless, in recent years, CPL based on inorganic substrates has also become a nascent topic, which is significant in exploring novel chirality- and luminescence-related properties and applications in inorganic materials. This short review summarizes the recent progress made regarding the following two aspects: 1) how to endow common inorganic luminophores with CPL activity; 2) how to use emerging chiral inorganic nanomaterials to design CPL-active systems. The general synthesis strategies, optical properties, applications and outlook of CPL-active inorganic materials are also demonstrated.
Keywords
INTRODUCTION
Chirality is termed as the phenomenon where a certain object is nonsuperposable on its mirror image. When two objects with such a relationship exist, they are known as enantiomers. Chirality is pervasive and manifests itself at different scales, ranging from macroscopic left and right hands to molecular-scale asymmetric carbon atoms and to various helical structures [Figure 1A-C]. The significance of chirality is well acknowledged, for example, only one given enantiomeric form of many biomolecules is preferred to maintain life. The above homochirality of life also raises an exacting enantiomer selectivity toward numerous daily chemicals, drugs and pesticides. Therefore, understanding chiral structure-property relationships is one of the most important topics across a broad range of disciplines[1,2].
Figure 1. Typical enantiomers of (A) left and right hands, (B) chiral carbon centers, (C) helices and (D) left- and right-handed CP light. (E) Schematic of CPL from two CPL-active enantiomers. CPL: Circularly polarized luminescence.
Light can also be chiral in the form of circularly polarized (CP) light. As shown in Figure 1D, around the direction of propagation, the electric field of CP light rotates in either a counterclockwise or clockwise helical pattern, resulting in left-handed CP light (LCP) or its counter-enantiomer of right-handed CP light (RCP), respectively. CP light has many promising applications, including three-dimensional (3D) and holographic display, spintronics, encryption, diagnosis and asymmetric synthesis[3-10]. Traditionally, a combination of a linear polarizer and a quarter-wave plate is applied to obtain CP light. However, this method suffers from a severe loss of light, especially in LED displays (up to 50%), invalidity beyond a certain frequency range and incompatibility with highly integrated photonic circuits[11,12]. Therefore, it is of interest to explore high-efficiency methods for the generation of CP light.
Usually, enantiomers are indistinguishable to some extent, since they share many identical properties. Fortunately, they respond differently toward LCP and RCP, which enables us to “see” microscale chiral matter using CP light-based spectroscopy[13,14]. Furthermore, the capability of chiral substances to manipulate the polarization states of light provides an alternative method to enable a luminophore to emit LCP and RCP beams unequally, which is known as circularly polarized luminescence (CPL)[15]. A schematic description for CPL in chiral systems is shown in Figure 1E. In principle, a pair of CPL-active enantiomers exhibit the same photoluminescence (PL) spectra. However, the LCP and RCP components in the emission are unequal when chirality is taken into consideration, i.e., LCP is over than RCP in one enantiomer, while the reverse is true in the other enantiomer. By measuring the difference between LCP and RCP (denoted by “IL-IR”, where IL and IR are the intensity of LCP and RCP, respectively) at a given emission wavelength, a CPL spectrum can be drawn using “IL-IR” as the vertical axis and emission wavelength as the horizontal axis. It is noted that the value of “IL-IR” is expressed as an ellipse angle θ (mdeg) in many studies and some commercial CPL instruments. This is because the overall polarization trajectory for a mixture of LCP and RCP light becomes an ellipse when IL is unequal to IR. The CPL signals usually overlap with the corresponding PL signals, but a pair of enantiomers show opposing CPL spectra.
Another important index to evaluate CPL is the dissymmetry factor of glum:glum = [2(IL-IR)]/(IL+IR). For the values of glum ranging from -2 to +2, only a given handedness CP light is emitted in the extreme cases (+2 for LCP only and -2 for RCP only), while no circular polarization occurs (i.e., IL=IR) when glum is zero. Consequently, CPL spectroscopy affords a unique tool to study the chiral structures and properties of matter in excited states, is the emission analog of common circular dichroism (CD) spectroscopy and is supplementary to fluorescence spectroscopy. In return, the construction of various CPL-active chiral luminescent materials further expands the applications of CP light.
Over the past decade, notable progress has been made in the exploration of novel CPL-active systems, together with the advancement of CPL spectroscopy. However, much research has focused on organic substances, partially due to the fact that research on chirality has a long history in organic chemistry[16-21]. According to the principles of CPL in chiral systems, the choices of either luminescent or chiral unity should be diverse, thus allowing for a broad range of potential CPL-active systems beyond organics. On this basis, it is noteworthy that inorganic-based CPL has become a new and active research topic in recent years. For example, many inorganic luminophores are employed to create more colorful CPL candidates and emerging chiral inorganic nanomaterials are able to impart various luminophores with CPL activity[22,23]. Such research is significant for many reasons, including the fact that it brings a new perspective to explore the luminescence properties and mechanisms of inorganic luminophores, especially in chiral environments. In addition, it provides a toolbox to analyze the structures of chiral inorganic materials and is helpful in understanding the chirality transfer in “matter-matter” or “light-matter” interactions. Finally, it also offers more inorganic/organic functional materials with the power to manipulate light to broaden CPL-based applications[24-28].
In this short review, we consider two inorganic-associated CPL-active systems, namely, inorganic luminophore-containing and chiral inorganic host-based systems. For the former systems, various classes of inorganic luminophores are described, including metal ions (e.g., lanthanide and chromium ions), perovskite nanocrystals (NCs), metal clusters, carbon dots and semiconductors nanoparticles (NPs). Given that there are some recent well-written reviews focusing on lanthanide complexes, chromium complexes and perovskite NCs, they are discussed at length here. With regards to the latter systems, the utilization of chiral silica (SiO2) nanomaterials, chiral inorganic crystals and helical inorganic assemblies as hosts for CPL is discussed. To demonstrate the recent progress in these systems, some typical examples are selected and discussed. The properties (components, construction strategies and excitation and emission wavelengths, glum) of these examples are summarized in Table 1.
Components, construction strategies and CPL properties of some inorganic-based CPL-active systems
Luminophore | Construction strategy type [chiral sources] | Excitation wavelength (nm) | Emission wavelength (nm) | Quantum yield (%) | Magnitude of glum |
1. Inorganic luminophore-based CPL | |||||
Yb3+ | II [PrPyBox, PhPyBOx] | 365 | 972 | 0.60-0.70 | 10-2, Ref.[78] |
NaYF4:Yb/Tm, NaYF4:Yb/Er | III [L-/D-GAm assemblies] | 980 | 360, 476, 802 (Tm), 540, 654 ( Er) | N | 10-3, Ref.[88] |
CaAl2O4:Eu, Nd | II [L-/D-cysteine] | 320 | 440 | N | 10-2, Ref.[81] |
ZnO | I [L/D-methionine] | 325 | 510 | N | N , Ref.[36] |
CdSe | II [L-/D-cysteine] | 451 | 605 | N | 10-3, Ref.[41] |
CdSe/CdS | II [L-/D-cysteine] | 400 | 583-683 | 35-60 | 10-4, Ref.[64] |
Ag clusters | I [see Figure 6A] | 365 | 558 | 3.9 | 10-1, Ref.[57] |
Au clusters | I [see Figure 12A] | 365 | 500 | 36.7 | 10-3, Ref.[86] |
Ag clusters | I [see Figure 12A] | N | 574 | 56, 95 | 10-3, Ref.[80] |
AuAg clusters | III [G-quartet nanofiber] | 356 | 475 | 9.42 | 10-2, Ref.[82] |
Ag clusters | III [zeolite assembly] | 360 | 520 | N | 10-2, Ref.[60] |
Carbon dots | III [L-/D-GAm assemblies] | 350-370 | 445-455 | N | 10-3, ref.[54] |
Carbon dots | III [L-/D-GAm assemblies] | 380 | 546, 604 | 30–55 | 10-3, Ref.[55] |
Carbon dots | III [cellulose nanocrystal assembly] | N | 450-620 | 5.2 (FL), 0.16 (Phos) | 10-1, Ref.[52] |
2. Chiral inorganic host-based CPL | |||||
Tb3+ | III [helical silica] | 230 | 545 | N | 10-3, Ref.[70] |
CsPbBr3 | III [helical silica] | 365 | 517.5 | N | 10-3, Ref.[73] |
Tetraphenylethylene (TPE) derivative | III [helical silica] | 366 | 453 | 57 | 10-2, Ref.[75] |
TPE derivative | III [helical silica] | 365 | 500 | 20-27 | 10-3-10-4, Ref.[74] |
Tb2O3, Eu2O3 | III [chiral silica nanofibers] | 375 | 545 (Tb2O3), 615 (Eu2O3) | N | 10-3, Ref.[71] |
Porphyrin (TCPP), TPE, MAPbBr3 | III [chiral silica nanofibers] | 375 | 650 (TCPP), 476 (TPE), 525 (MAPbBr3) | N | 10-3, Ref.[72] |
Eu3+ | III [TbPO4·H2O] | 365 | 590-705 | N | 10-3-10-2, Ref.[26] |
DACT and TMD | III [assemblies of GdOOH nanowires] | N | 550 (DACT), 650 (TMD) | N | N, Ref.[76] |
We provide an overview of inorganic-based CPL. Firstly, several types of construction strategies for CPL-active systems are summarized, which are further clarified by selected examples of CPL-active systems containing inorganic luminophores or chiral inorganic nanomaterials. Next, CPL-active systems based on some special luminescent processes are listed, which are different from the common down-conversion luminescence in the visible range. Some typical applications are displayed, followed by a short conclusions and perspectives section.
CONSTRUCTION STRATEGIES FOR CPL-ACTIVE SYSTEMS
There are various strategies to achieve CPL in chiral systems. A straightforward strategy [type I, Figure 2A] is where the luminophore itself is chiral, which is known as an intrinsically chiral luminophore. However, unlike organic molecules with diverse chiral geometries, many inorganic luminescent substances are not chiral. Therefore, a subtle design of symmetry-breaking synthetic methods is often required to produce chiral structures[26,29]. Chirality transfer is widespread on various interfaces[30,31], which makes it more flexible and versatile to enable CPL, even on preformed achiral luminophores. More specifically, the chirality can be transferred from chiral ligands [type II, Figure 2B] and chiral hosts [type III, Figure 2C]. In Type I, to acquire chiral products, chiral ligands or hosts are also employed but are finally removed. Compared with type I, one characteristic in types II and III is that the chiral ligands or hosts are required to co-exist with the luminophore for chirality inducement.
Figure 2. Three types of construction strategies of CPL-active systems: (A) intrinsically chiral luminophores (type I); (B) chiral ligand-induced CPL (type II); (C) “host-guest”-induced CPL (type III). CPL: Circularly polarized luminescence.
Intrinsically chiral luminophores (type I)
The chirality in inorganic materials can appear in various forms. For crystalline materials, point and space groups are basic tools to describe crystal structures. There are 230 group spaces, which are created via the combination of 32 point groups and 14 types of Bravais lattices. Point and space groups are also useful to judge chirality and chiroptical activity. Chiral crystals can exist in 65 Sohncke space groups, which are divided into two categories: I) 22 chiral groups with helical operations that thus contain 11 enantiomeric pairs (e.g., P31 and P32); II) the remaining 43 groups are achiral; however, if the unit (i.e., the smallest collection of atoms from which the whole crystal is restored by the application of all symmetry operations of the space group and translation) is chiral, the crystals can be chiral[32]. Early in 1948, chiral crystals with CPL activity were demonstrated in sodium uranyl acetate Na[UO2(CH3COO)3] crystals formed from achiral molecular subunits. The space group of Na[UO2(CH3COO)3] crystals belongs to the chiral space group of P213 and the chirality is caused by the helical canting of the three [UO2(CH3COO)3] rings and the noncentrosymmetric spatial arrangement of four coordination clusters. Moreover, the crystallographic structure is cubic, which is advantageous to observe CPL with small interruptions caused by birefringence. With such unique structures, a high glum of 1.31 was observed in Na[UO2(CH3COO)3] crystals by Riehl and Richardson[33] and Moran et al.[34].
Another method to endow crystals with optical activity is by creating crystalline defects (e.g., screws and distortions), which are caused by chiral additives regulating the nucleation-growth process[35]. In addition to the lattice chirality described above, chiral nano-/micromorphologies (e.g., copying the helical patterns of chiral organic templates) on a higher length scale can also induce chiroptical activity in crystalline or amorphous materials[31]. Duan et al.[36] demonstrated chiroptically-active crystalline ZnO films with multiple chiralities on different scales. Using chiral amino acids of L-/D-methionine (Met) as both structure-directing and symmetry-breaking agents, chiral hydroxide zinc carbonate (HZC) nanostructures were formed on quartz substrates. After removing organic species by calcination at 550 °C in air, HZC was transformed into chiral nanostructured ZnO films (L-CNZF, obtained with L-Met) with three levels of chirality [Figure 3]. On the primary level, the crystal structure of the ZnO nanoplate shows a subtle helical distortion along the [10-11] axis in a left-handed coiled pattern together with some lattice defects, which is caused by the chiral center of the methionine molecules. On the secondary level, several dozens of ZnO nanoplates are bent into a ZnO particle with a right-handed helix form. On the tertiary level, the arrangement of several ZnO particles forms a left-handed circinate helical morphology on the microscale. For the ZnO films (D-CNZF) obtained using D-Met, the handedness one of each level is just opposite to the corresponding one of L-CNZF. These ZnO films showed various chiroptical activities in their CD, CPL and Raman optical activity spectra. Under a 325 nm excitation, D-CNZF and L-CNZF exhibited antipodal CPL signals centered at 510 nm, consistent with the fluorescence band maximum.
Figure 3. (A) SEM image of L-CNZF. (B) TEM image and (C) SAED patterns of a single ZnO nanoplate. (D) Schematic of hierarchical chirality in L-CNZF. (E) PL and CPL spectra of L- and R-CNZF. Reproduced with permission from Ref.[36]. Copyright (2015) John Wiley and Sons. SAED: Selected area electron diffraction; CPL: circularly polarized luminescence; PL: photoluminescence; CNZF: chiral nanostructured ZnO films.
Chiral ligand-induced CPL (type II)
Small chiral organic molecules are widely found and are powerful for producing chiroptical signals on inorganic substances. As shown in Figure 4, for example, CD signals are observed on plasmonic metal (Au and Ag) and semiconductor (e.g., CdS, CdSe and CdTe) NPs capped with chiral ligands[40-42]. The chirality transfer from ligands to inorganics can proceed through various mechanisms, including surface atom distortion with asymmetric clusters, the chiral arrangement of ligands on the surface, crystalline defects and the electronic interaction or coupling between chiral molecules and inorganic species[43,44].
Figure 4. (A) Optimized geometries and induced CD spectra. (B) CPL (top) and total luminescence (bottom) spectra of L- and D-cysteine-capped CdSe quantum dots. Reproduced with permission from Ref.[41]. Copyright (2013) American Chemical Society. (C) Chiral riboflavin molecule-induced CD optical activity in Au islands deposited on a glass substrate. Reproduced with permission from Ref.[42]. Copyright (2013) American Chemical Society. CD: Circular dichroism; CPL: circularly polarized luminescence.
The combination between inorganic nanomaterials and chiral ligands can be fulfilled by two synthetic routes, namely, ligand-guided one-step synthesis or post-modification by ligands exchanges. Although these methods appear easy to carry out, the chirality induction is dependent upon many factors, such as the types of ligands, the sizes/shapes of the NPs and the synthetic conditions. Furthermore, although the chirality is mainly judged by the CD signals, CD activity does not guarantee CPL activity. This is understandable based on the differences between the CD and CPL mechanisms, i.e., CD is associated with absorption from the ground states, while CPL is associated with emissions in the excited states.
“Host-guest”-induced CPL (type III)
Based on the concept of chirality induction in type II, it is found that various luminescent guests show chiroptical activity after being embedded in many chiral materials as chiral hosts. Moreover, several different guests can be simultaneously integrated into one host to acquire white CPL. In particular, with this method, emerging novel inorganic nanomaterials have found broad applications in CPL in recent years by encapsulating inorganic and/or organic luminescent guests. As a result, this strategy is more flexible for producing multicolor CPL by a rational combination between hosts and guests, thus widening the scope of inorganic CPL-active systems.
OVERVIEW OF INORGANIC-ASSOCIATED CPL-ACTIVE SYSTEMS
Inorganic luminophore-containing systems
Metal ion-based luminophores
Lanthanide ions [Ln(III)] are appealing for CPL because of their interesting optical properties, such as a large pseudo-Stokes shift, long lifetime and unique spectral fingerprint from the ultraviolet (UV) to near-infrared (NIR) range. In particular, the magnetic dipole allowed transitions in lanthanide ions are advantageous for a high glum; for example, a high glum value of 1.38 was reported for Eu(III) complexes[45]. Since lanthanide ions are isotropic, lanthanide-based CPL is predominantly demonstrated in various chiral lanthanide complexes, where the coordination orientation of chiral ligands can form left- or right-handed twists and helices. The CPL mechanism of these complexes can be interpreted based on multipole [Ln(III)]-point charge (chiral ligand) interactions and/or multipole [Ln(III)]-dipole (excited-ligand) coupling. Since there have been several recent reviews on CPL-active lanthanide complexes, they are not described here and the reader can refer to Refs.[46-48].
Other transition metal complexes with CPL activity have also been reported. For example, chromium(III) (Cr3+) is of interest due to its earth abundance, kinetic inertness and magnetically allowed but electric-dipole forbidden emissions (600–800 nm) from the Cr(2E) and Cr(2T1) states. In a recent report, helical [Cr(dqpR)2]3+ (dqp = 2,6-di(quinolin-8-yl)pyridine; R = OCH3, Br or C ≡ CH) complex enantiomers were resolved[49]. A high quantum yield (up to 17%), long-lived excited lifetimes (1.35 ms) at room temperature in aqueous solutions and a large glum (0.20) were found for these chiral complexes, thereby providing satisfactory CPL brightness. More reports on CPL-active chromium-based materials have been well summarized in a recent review[50], so this topic will not be discussed in detail here.
In addition to metal complexes, metal ion-containing luminophores can also be found in metal oxides (e.g., Eu2O3) and metal ion-doped inorganic matrixes (e.g., NaYF4:Yb/Tm, NaYF4:Yb/Er and CaAl2O4:Eu,Nd). As discussed below, they can also be engineered with CPL in highly thermal stable oxides for up-conversion, NIR emission and phosphorescence applications.
Carbon dots
Since the discovery of carbon dots in 2006, their luminescence has gained increasing attention as they possess many important features, such as easy production, rich raw materials, multicolor emission, high stability and versatile surface chemistry. In some early reports, carbon dots with chiroptical activity in their CD spectra were easily prepared using chiral molecular precursors; however, the CPL activity was not easily demonstrated[51]. The CPL of carbon dots was recently activated using the simple “host-guest” strategy[52-55].
One special characteristic of carbon dots is that their emission wavelength can be tuned by changing their excitation wavelength. Building on this phenomenon, Li et al.[54] showed an excitation-dependent CPL example. A chiral L-/D-glutamic acid gelator (L-/D-GAm) was assembled into a helical structure that was used as a chiral host to co-assemble with the nitrogen-doped carbon dot guest. The as-formed composites (L-and D-co-gels) appeared as spiral nanotubes [Figure 5A]. The maximum emission wavelength changed from 400 to 460 nm as the excitation wavelength increased from 300 to 400 nm [Figure 5B]. Similarly, the CPL signals were also red-shifted with increasing excitation wavelength from 350 to 370 nm [Figure 5C] and the |glum| was ~ 2×10−3-4×10−3 under a 360 nm irradiation.
Figure 5. Morphologies and optical properties of D-co-gel: (A) FE-SEM image; (B) PL; (C) CPL spectra with the fitting line (inset: plot of the maximum CPL emission wavelength versus the excitation wavelength) under different excitations. Reproduced with permission from Ref.[54]. Copyright (2020) American Chemical Society. (D) Mirror image CPL spectra of three carbon dot-doped co-gels. (E) PL spectra of white-emitting co-gel (insets: a photograph of the co-gel under 365 nm (top right) and its CIE coordinate value (bottom left). (F) glum of white-emitting co-gel from D-GAm (black) and L-GAm. Reproduced with permission from ref.[55]. Copyright (2021) John Wiley and Sons. PL: Photoluminescence; CPL: circularly polarized luminescence.
Again, using L-/D-GAm co-gels as the hosts to encapsulate three kinds of carbon dots with blue, yellow and red emission colors, Ru et al.[55] demonstrated a white CPL system. These carbon dots displayed CPL signals at 450, 550 and 610 nm, with a |glum| of ~10-2 [Figure 5D]. Furthermore, by simply blending these three kinds of carbon dots at a mass ratio of 4.63:15.75:1 (blue:yellow:red), white emission was fulfilled with a CIE chromaticity diagram coordinate value of (0.334, 0.329) [Figure 5E]. This as-obtained mixture showed circularly polarized white-light emission with a glum of ~ 2.5×10-3 [Figure 5F], which is smaller than the glum of the individual carbon dots.
Metal clusters
Fluorescent metal clusters composed of a few to a hundred atoms represent a class of special luminophores that provide a link between nanoparticles and the atomic behavior of metals. These ultrasmall metal clusters exhibit molecule-like properties, including size-regulated fluorescence and discrete electronic states, which are promising for a broad range of biological applications. The ultrasmall size of the metal clusters is also possibly advantageous for the combination between metal clusters and ligands (or hosts), meaning that diverse CPL-active metal clusters can be synthesized by the three strategies discussed above (types I-III)[56].
Wu et al.[57] synthesized spirocyclic Ag clusters [2-(R) and 2-(S), Figure 6A] using two types of chiral diide ligands and 2-(pyridin-2-yl)-1H-benzo[d]imidazole (PBZ). The intrinsic chirality origin of 2-(R) and 2-(S) can be ascribed to the chiral diides, the spirocyclic arrangement of a Ag9 cluster and the helical arrangement of PBZ molecules. In addition, 2-(R) and 2-(S) can crystalize into crystals with the chiral space group of P21. In a mixed solvent of water and methanol, with an increase in the water fraction, 2-(R) and 2-(S) assemble into μm-long helical nanofibers. Furthermore, the solid films of 2-(R) and 2-(S) show strong and mirror-imaged CPL signals centered at 558 nm, with a high glum of 0.16 [Figure 6B]. Such a high glum may benefit from the exciton couplings between the helically arranged PBZ molecules of individual spirocyclic clusters and the microscopic helical assembly. In other words, both the molecular-level and microscale chirality contribute to the high glum. The assembly- and aggregate-induced CPL of metal clusters is also found in gold clusters of Au3[R/S-BINAP]3Cl and copper clusters of [Cu14(R/S-DPM)8]6+ (DPM = R/S-2-diphenyl-2-hydroxylmethylpyrrolidine-1-propyne), which are CPL inactive when dispersed in good solvents (e.g., CH2Cl2) but become CPL active in the aggregate states after the addition of poor solvents (e.g., n-hexane)[58,59].
Figure 6. (A) Spirocyclic Ag cluster structure of 2-(S). (B) CPL spectra (solid line) and glum (dotted line) for film samples of 2-(R) and 2-(S). Reproduced with permission from ref.[57]. Copyright (2021) John Wiley and Sons. (C) SEM image of ZAS(180, 4.34)-80 showing helices with yellow blocks. (D) CPL spectra of AgNC/ZAS(180, 4.34)-T under different temperatures (T). Reproduced with permission from Ref.[60]. Copyright (2020) John Wiley and Sons. CPL: Circularly polarized luminescence; ZAS: zeolite LTA superball.
Using a host-guest method, Tao et al.[60] reported the CPL activity of luminescent Ag nanoclusters (AgNC) incorporated in colloidal zeolite LTA superball (ZAS) assemblies. The macroscopic porous films built from ZAS showed 3D long-range periodicity featuring a photonic band gap (PBG) [Figure 6C]. For one-dimensional PBG structures, the maximum PBG wavelength λmax is calculated as follows: λmax = nPsinθ, where n and P are the average refractive index and helical pitch of a PBG structure, respectively, θ is the angle of the incident light with respect to the PBG structures. A PBG structure with a helical periodic profile can selectively reflect CP light, especially at λmax, with the same handedness of the PBG structure while allowing the transmittance of the opposite-handed CP light[61-63]. For example, LCP is blocked while RCP is transmitted within a left-handed helical PBG structure, and therefore a large difference between LCP and RCP was detected with a high glum. By embedding luminescent AgNC, the AgNC/ZAS enabled the selective reflection of R-CP light and allowed L-CP light, resulting in a glum of 0.013 at a 520 nm emission. The glum can be tuned by the assembly temperature to change the helical pitches of PBG structures [Figure 6D].
Semiconductor nanocrystals
Semiconductor nanocrystals (NCs), mainly comprised of elements from the II-VI, III-V and IV-VI groups of the periodic table, show many promising optical properties, including exceptional brightness, high quantum yield, superior photostability and size-tunable emission. Colloidal semiconductor NCs are usually capped with organic ligands for the purposes of surface passivation and good dispersibility. In addition, surface ligands also influence the photoluminescent properties. By a ligand-exchange step, chiral ligands can be absorbed onto the surfaces of NCs to make semiconductor NCs CPL active[27,41,64].
As shown in the previous work by Tohgha et al.[41], CPL signals at ~ 605 nm (|glum| of 3-4×10-3) are observed on CdSe NPs modified with D- and L-cysteine. Furthermore, Cheng et al.[64] conducted a systematic study of the CPL properties of cysteine-modified CdSe/CdS core-shell NCs with different shapes [Figure 7A][27]. The CdS shell could improve the luminescent quantum yield (QY) of the CdSe core. Interestingly, even if the CdSe core is separated from chiral ligands by the CdS shell, CdSe still shows CPL bands from 550 to 650 nm with a |glum| of 1-9×10-4. The CPL performance may be caused by orbital coupling and Coulombic dipole interactions between CdSe and cysteine. The PL and CPL could be manipulated by the thickness and shapes of the CdS shell (glum increases with decreasing CdS shell thickness) but is proportional to the QY [Figure 7B and C]. This work confirmed that chiral ligands can control the whole wavefunction even though there exists an energy barrier of the thin CdS shell.
Figure 7. (A) Schematic anisotropic shapes of CdSe/CdS (gold-colored core: CdSe; orange-color shell: CdS). (B) Dependence of glum of D-Cys-CdSe/CdS, CdS shell thickness and (C) QY on different shapes. Reproduced with permission from Ref.[27]. Copyright (2020) American Chemical Society. QY: Quantum yield.
Perovskite nanocrystals
Luminescent metal halide perovskite colloidal NCs composed of inorganic octahedral layers and organic ligands represent a class of promising next-generation optoelectronic materials. As summarized in recent reviews[65-67], using type I and II strategies with the assistance of a series of chiral ligands, CPL is also extended to perovskite NCs with potential applications in CP light detection, quantum networks, ferroelectrics and spintronics. In addition to organic molecules as chirality sources, some inorganic nanomaterials are also able to endow perovskite NCs with CPL activity, thereby offering new CPL-active candidates.
Chiral inorganic host-based systems
Chiral silica
Over the past two decades, a variety of chiral silica nanomaterials have been reported and their chirality can be manifested by helical outwards or molecular-scale asymmetry on the siloxane framework[68]. Compared with chiral organic molecules, a prominent feature of silica is its thermal stability (close to 1,000 °C), which is desirable for high-temperature synthetic procedures. It has been confirmed that ions, molecules and nanoparticles incorporated within these chiral nano-silica materials can become CD active[69,70]. With the above success, it is of interest to explore chiral silica-based CPL-active systems.
Our group first demonstrated several entirely inorganic silica-lanthanide oxide CPL systems[71]. In our previous work, we found that linear polyethylenimine and L- (or D-) tartaric acids can co-assemble into catalytic chiral templates to direct the formation of chiral SiO2 nanofibers [Figure 8A]. Vibrational circular dichroism spectroscopic analysis revealed the asymmetric feature of Si-O-Si frameworks on the molecular scale, which can be maintained even up to 900 °C. These SiO2 nanofibers are used as chiral nanoreactors for the formation of sub-10 nm Eu2O3 (or Tb2O3) NPs, which are transformed from the Eu3+ (or Tb3+) trapped in SiO2 after heating at 900 °C in air [Figure 8B and C]. Interestingly, the CPL features of NPs are observed around the emission wavelengths of 615 nm (Eu2O3) and 545 nm (Tb2O3) with a |glum| of the order of 10-3. However, the excitation wavelength using homemade CPL spectroscopy is 375 nm, which deviates from the optimized excitation wavelength from PL spectra. The influence of the excitation wavelength on the CPL performance was not probed.
Figure 8. (A) Schematic of chiral SiO2 nanofiber synthesis using PEI/D-tart (or PEI/L-tart) as chiral catalytic templates and their usage to prepare chiral lanthanide oxide (M2O3) NPs. (B) TEM image of Tb2O3/D-SiO2 nanofibers. (C) TEM image of an individual Tb2O3 nanoparticle on Tb2O3/D-SiO2 nanofibers. Reproduced with permission from Ref.[71]. Copyright (2018) John Wiley and Sons. (D) Various CPL-active systems using chiral silica as hosts to incorporate organic fluorescent dyes, AIEgens and perovskites NCs. Reproduced with permission from Ref.[72]. Copyright (2020) John Wiley and Sons. CPL: Circularly polarized luminescence.
In addition to lanthanide oxides, other luminophores are also endowed with CPL after being embedded within these SiO2 nanofibers [Figure 8D][72]. As a unique luminescent phenomenon, aggregation-induced emission (AIE) has gained ever-increasing attention. Tetraphenylethene (TPE) and its derivatives are representative AIE luminogens (AIEgens) with high quantum yields and ready functionalization. TPE molecules can be easily absorbed onto SiO2 nanofibers modified with phenyl groups and have already demonstrated AIE-based CPL signals of ~ 476 nm in the aggregated state. When SiO2 was modified by amine groups, fluorescent carboxylic molecules (5,10,15,20-tetrakis(P-carboxyphenyl)porphyrin, 1-pyrenecarboxyl acid and fluorescein) were attached to SiO2 and then showed CPL signals at their characteristic emission bands with a |glum| of 2-5×10-3. In addition, these amine-modified SiO2 materials could work as chiral reactors for the in-situ formation of perovskite NCs with CPL activity. These results imply the significant potential of chiral SiO2 in designing CPL-related materials.
Harada et al.[70] found that cationic gemini surfactants of C2H4-1,2-[(CH3)2N+C16H33]2 self-assemble into helical structures in the presence of L- or D-tartrate counter ions. Using these organic helices as templates (dented by 16-2-16 L-tartrate or 6-2-16 D-tartrate), elegant right- and left-handed SiO2 nanohelices could be prepared and further used as chiral hosts to synthesize lanthanide ion- and perovskite-containing CPL systems [Figure 9A]. After the absorption of Tb3+ onto SiO2 nanohelices and calcination at 900 °C for 4 h in air, the small amount of Tb3+ (~ 0.31%) can display CPL outputs at ~ 545 nm (irradiated at 230 nm) with a |glum| of ~ 10-3 due to the helical-morphological chirality of SiO2. The chiroptical activity may be due to the 4f orbitals of Tb3+ being chirally distorted by the asymmetrically arranged Si-O framework. In addition, after modifying the surfaces of SiO2 helices with amine by 3-aminopropyl-triethoxysilane, perovskite CsPbBr3 nanocrystals (PNCs) are grafted onto SiO2[73]. When suspended in toluene, PNC-SiO2 do not exhibit CD and CPL signals. In contrast, after casting the above suspension on quartz and drying, the PNC-SiO2 in a dried film state became both CD and CPL active. The maximum CPL signals locate at 517.5 nm (under a 360 nm excitation) and the |glum| reaches 5.7-6.9×10-3. It was found that PNCs are closely packed and helically arranged around SiO2 helices during the solvent evaporation process, which facilitated the dipolar interaction between the PNCs and then produced CPL.
Figure 9. (A) Molecular structure of 16-2-16 L-tartrate (left) and TEM images of L-SiO2:Tb3+ calcined at 900 °C (middle) and nanohelices@PNCs in a dried film and the cross sections (yellow and red frames) obtained with 3D tomography reconstructions (right). Reproduced with permission from Ref.[70]. Copyright (2021) Royal Society of Chemistry. Reproduced with permission from Ref.[73]. Copyright (2020) American Chemical Society. (B) SEM image of TPES-P. Reproduced with permission from Ref. [74]. Copyright (2019) Royal Society of Chemistry. (C) SEM image of L-CASN. Reproduced with permission from Ref.[75]. Copyright (2019) Royal Society of Chemistry. TPES-P: TPE-silica with plus helice. L-CASN: left-handed chiral lamellar mesostructured AIEgen-silica hybrid hollow nanotubes;
The synthesis of the CPL-active materials above occurs via a two-step process, which requires the synthesis of SiO2 in advance. In fact, the luminescent components could also be one-step incorporated into a siloxane framework during the condensation of silica sources (e.g., tetraethyl orthosilicate and multisilylated organic molecules). For example, Cai et al.[74] designed TPE-containing bis(triethoxysilane) (BTSTPE) as the silica precursor and then prepared TPE-bridged polybissilsesquioxane (TPE-silica) nanotubes with single-handed helicity using the self-assemblies of a pair of chiral cationic low-molecular-weight gelators (L-ValPyBr and D-ValPyBr) as templates [Figure 9B]. Strikingly, the TPE-silica products showed high thermal stability with only a 5% weight loss at ~ 500 °C. The helical TPE-silica powders emit green light at ~ 500 nm with a quantum yield of over 21%, which is higher than that of its precursor of BTSTPE. The CPL outputs are found at 500 nm with a |glum| of 0.6-1.6×10-3. In another work, Zhang et al.[75] reported AIEgen-silica helical nanotubes displaying CPL signals at 453 nm with a glum up to 0.02 [under a 366 nm excitation, Figure 9C]. The CPL-active products were obtained by the co-assembly from 2-[4-(1,2,2-triphenylethenyl)-phenoxy]-acetic acid (as the luminophore), N-trimethoxysilylpropyl-N,N,N-trimethylammonium chloride (as a co-structure directing agent) and tetraethylorthosilicate (as a silica precursor).
Other chiral inorganic hosts
As discussed above, there are 22 chiral space groups in crystal structures, providing the possibility of generating intrinsically chiral crystalline materials. For example, the space groups of TbPO4·H2O crystals belong to the chiral group of P3121 or its enantiomorph P3221. Hananel et al.[26] employed tartaric acid enantiomers as chirality inducers to produce chiral TbPO4·H2O doped with Eu3+. Under a 365 nm excitation, Eu3+ in the TbPO4·H2O host showed strong CPL signals between 590 and 720 nm corresponding to the 0D5→7FJ transitions of Eu3+. The glum could be 0.06 at 612 nm and is comparable with many chiral Eu(III) complexes. In this work, CPL spectroscopy is also used as a tool to study the symmetry breaking behavior of chiral TbPO4·H2O crystals controlled by the enantiomeric excess (e.e.) of tartaric acid.
Unlike the case above where the symmetry breaking is regulated by chiral molecules, Zhang et al.[76] found that oleyamine-coated GdOOH nanowires with a diameter of less than 1 nm could randomly transform into macroscopic left- and right-handed helical assemblies (MHAs) at the centimeter level by an evaporation-induced self-assembly process without the assistance of a chiral dopant [Figure 10A,B][76]. The spontaneous formation of helices is a result of an energy-minimizing process and the handedness is uncontrollable. Fortunately, the left- and right-handed helices can be observed by the naked eye and easily separated. Achiral fluorescent molecule guests of DACT and TMD embedded into MHAs showed CPL signals at their emission bands at 500 and 625 nm, respectively.
Figure 10. (A) Cryo-electron microscopy image and its large-magnification version (upper inset) of the sub-1 nm GdOOH nanowires (bottom: a photograph of the dispersion of nanowires). (B) CPL spectra of DACT-MHA. Reproduced with permission from Ref.[76]. Copyright (2020) American Chemical Society. CPL: Circularly polarized luminescence; MHA: macroscopically spiral assemblies.
SELECTED SPECIAL CPL-ACTIVE SYSTEMS
Many luminophores show down-conversion fluorescence with emission bands in the visible range. In fact, there are other luminescent processes, including up-conversion PL, phosphorescence, thermally activated delayed fluorescence (TADF) and NIR emission. Studies of the CPL related to these processes could enrich our understanding of the origin of CPL and help us to explore more CPL-related applications. Therefore, some special CPL-active systems are presented in this section.
Up-conversion CPL
Up-conversion luminescence is capable of converting long-wavelength NIR light to shorter-wavelength narrow and sharp emission ranging from UV, to visible and even to shorter NIR light. Up-conversion luminescence is utilized widely in a broad range of areas, especially in biological and biomedical ones, due to low background fluorescence, weak photodamage, good penetration in tissues and high sensitivity.
Lanthanide ions (e.g., Yb3+, Er3+ and Tm3+) doped in fluoride-based matrixes (e.g., NaYF4 and NaGdF4) possess high up-conversion efficiencies because of sufficient metastable energy and excited states with a long lifetime. Yang et al.[77] offered a dexterous solution for an up-conversion CPL based on a radiative energy transfer process from NaYF4:Yb/Tm Upconverting nanoparticles (UCNPs) to CsPbBr3 perovskite nanocrystals (PKNCs) in the chiral nematic liquid crystal (N*LC) featured with PBG [Figure 11A]. A high |glum| is possible in a PBG structure due to strong handedness-selective reflection of CP light. However, the light loss may occur and the emission of emitters is boosted when the emission wavelength is located at the edge of the photonic bandgap, while the emission is suppressed at the center of the bandgap. Therefore, it is a challenge to achieve both a large glum and high emission efficiency. To address this issue, an achiral nematic liquid crystal SLC1717 and chiral dopant R-(or S-)811 were used to form left- or right-handed helical N*LC, where two different emitters of NaYF4:Yb/Tm and CsPbBr3 were embedded. Under a 980 nm excitation, NaYF4:Yb/Tm UCNPs showed emission bands at 450 and 475 nm near the edge of the bandgap of N*LC and these emissions were thus enhanced and the glum at 475 nm was 0.3. The emission of PKNCs was centered at 495 nm, which overlaps with the center of the bandgap. Fortunately, the emission of PKNCs could be enhanced via a radiative energy transfer with quenching the emission of NaYF4:Yb/Tm at 475 nm. Meanwhile, a very high glum of 1.1 was still achieved for PKNCs at 495 nm.
Figure 11. (A) Schematic representation of RET process from UCNPs to CsPbBr3 PKNCs in N*LC (the emissions of CsPbBr3 PKNCs and UCNPs were located at the center and the edge of the photonic bandgap, respectively, left) and UC-CPL spectra of CsPbBr3 PKNCs/UCNPs in N*LC with different incident power of a 980 nm laser (right). Reproduced with permission from Ref.[77]. Copyright (2020) John Wiley and Sons. (B) NIR-CPL spectra (top) of both the enantiomers of Yb(TTA)3iPrPybox (left) and Yb(TTA)3PhPybox (right) and total NIR emission (bottom). Reproduced with permission from Ref.[78]. Copyright (2019) Royal Society of Chemistry. RET: Radiative energy transfer; PKNCs: perovskite nanocrystals; UCNP: upconverting nanoparticles; TTA: 2-thenoyltrifluoroacetonate.
NIR emission CPL
The emission at the NIR range from luminophores is highly beneficial to optical applications in transparent biological windows because of the suppressed absorption or scattering from skin and blood. However, there is a lack of suitable, reliable detection instruments for weak NIR emission and reports on NIR-CPL are scarce. Yb3+ is a typical NIR emitter in the range of 900–1050 nm. The complex of Yb(TTA)3 (TTA = 2-thenoyltrifluoroacetonate) shows relatively good quantum yields due to the antenna effect of TTA ligands. On the basis of Yb(TTA)3, Zinna et al.[78] introduced additional chiral ligands (iPrPyBox or PhPyBox) to synthesize two groups of chiral Yb(TTA)3iPrPyBox and Yb(TTA)3PhPyBox complexes [Figure 11B]. To measure the NIR-CPL of these complexes, the authors conducted modifications on spectrofluoropolarimeter apparatus using an Ag-O-Cs photomultiplier tube as the detector and a photoelastic modulator coupled with an uncoated Glan–Thompson polarizer for polarization discrimination. For Yb(TTA)3iPrPyBox, relatively sharp peaks at 972 nm (2F5/2 → 2F7/2 transition of Yb3+) appeared on their CPL spectra appeared with a glum of -0.025 and +0.029 for (R,R) and (S,S) enantiomers, respectively. Similar results were also found for Yb(TTA)3PhPyBox enantiomers.
Thermally activated delayed fluorescence CPL
In principle, TADF enables an internal quantum efficiency of 100% by harvesting triplet (T1) excitons, which is fulfilled through the reverse intersystem crossing from the triplet (T1) to singlet (S1) state in a thermally activated process. Therefore, TADF has received widespread attention, especially in organic light-emitting diode displays. Recently, TADF-based CPL has also gained increasing interest in organic molecules[79]. In contrast, TADF-CPL in inorganic emitters is rare. Han et al.[80] synthesized octahedral Ag(I) clusters using chiral ligands named as L/D and PL/PD enantiomers [structures shown in Figure 12A]. In the case of PL/PD, Ag6 cores in the Ag clusters of Ag6PL6 and Ag6PD6 are distorted and deviate from the regular octahedral Ag6 skeleton, and these clusters crystallize in the chiral monoclinic space group P21. Based on chiroptical spectra and DFT simulations, the chiroptical activity of the clusters is mainly attributed to the asymmetric Ag6 core and the chiral interface between Ag and the coordinated C and N atoms. The PLQY of Ag6PL6/Ag6PD6 surpasses 95% at room temperature, which is a result of TADF confirmed by the temperature-dependent luminescence spectra and decays. Finally, these clusters display TADF-based CPL signals at 575 nm (excited at 370 nm) with a glum of ~ 4×10-3.
Figure 12. (A) Structures of Ag6L6/D6 and Ag6PL6/PD6 enantiomers, L/D, PL/PD and octahedral Ag6 framework in Ag6L6/D6. Reproduced with permission from Ref.[80]. (B) Images of various lanthanide-containing phosphor materials before and after modification with L/D-Cys via ball milling (top) and schematic of CaAl2O4:Eu,Nd phosphor attached by chiral Cys molecules on the surface and the induction of the phosphorescence-based CPL (bottom). Reproduced with permission from Ref.[81]. Copyright (2021) John Wiley and Sons. (C) Schematic of hybrid chiral photonic showing dual CPL and CPRTP (left), an experimental setup for the measurement of CPRTP (middle) and phosphorescence emission spectra of hybrid chiral photonic films recorded with an L-CPF or R-CPF (right). Reproduced with permission from Ref.[52]. Copyright (2020) American Chemical Society. CPL: Circularly polarized luminescence; CPRTP: circular polarized room-temperature phosphorescence; CPF: circularly polarized filter.
Phosphorescence CPL
As for the origin of photoluminescence, phosphorescence is an important complementary mechanism to fluorescence. Phosphorescence is the emission from triplet-excited states and its lifetime is longer than fluorescence. Therefore, phosphorescence is appealing in optical sensors, information security, optoelectronic devices and biomedical agents.
Phosphorescence can be observed in lanthanide and Cr ions at room temperature. As demonstrated in Figure 12B Hao et al.[81] reported a simple method to obtain colorful CPL-active inorganic persistent lanthanide-based phosphor materials with a long afterglow, including a yellow-green SrAl2O4:Eu,Dy, purple CaAl2O4:Eu,Nd, blue-green Sr4Al4O25:Eu,Dy, sky blue Sr2MgSi2O7 and red Y2O2S:Eu,Mg,Ti. These commercial phosphor powders are mixed with D- (or L-) cysteine (Cys) in ethanol and subjected to ball-milling for several hours and then the D-/L-Cys-modified phosphors are collected. Using this simple process, these phosphors became CPL-active with a |glum| of 10−3-10−2, of which a maximum value was observed in the CaAl2O4:Eu,Nd sample (2.10×10−2). The CD and CPL activity of these inorganic phosphors was induced by chiral Cys molecules, which were absorbed via metal-sulfur bonds.
Compared with lanthanides ions, the observation of room-temperature phosphorescence on other luminescent materials is not straightforward. Xu et al.[52] demonstrated a three-component system with both fluorescence- and phosphorescence-based CPL of carbon dots (CDs) at room temperature [Figure 12C]. Cellulose nanocrystals are used to form chiral photonic films, in which poly(vinyl alcohol) (PVA) and luminescent CDs are integrated by a co-assembly process. Because of the PBG effect, CPL was observed when the fluorescence wavelength (460 nm, excited by 365 nm) of the CDs located within the bandgap. The employment of PVA seemed to restrict molecular motions and inhibit oxygen quenching to maintain triplet excitons, thus leading to phosphorescence afterglow (480-500 nm) lasting for 2 s, even after turning off the UV (260 nm) irradiation. However, there is an overlap between the fluorescence spectrum (with a peak located at 450-470 nm) and the room-temperature phosphorescence (RTP) spectrum (with a peak at 480-500 nm). In other words, the fluorescence bands are close to the RTP bands. Therefore, it is difficult to differentiate FL-based CPL from RTP-induced CPL on the as-used JASCO CPL-200 spectrophotometer. These authors designed an analytical device [shown in Figure 12C, middle] to measure circular polarized room-temperature phosphorescence (CPRTP), which is fulfilled by combining a fluorospectrophotometer and an L- (or R-) circularly polarized filter (CPF). The CPF was covered on the detector at the phosphorescence measurement mode to record the CPRTP spectra. In principle, L-CPRTP would be blocked by the RCPF, while R-CPRTP would be blocked by the LCPF. On this basis, it was found that the CPRTP intensity recorded with LCPF was weaker than RCPF. By borrowing the definition of glum, a so-called gRTP is defined as gRTP = [2×(IL-IR)]/(IL+IR), where IL and IR are the intensity of RTP recorded under LCPF and RCPF, respectively. The highest absolute value of gRTP could reach 0.461.
APPLICATIONS OF INORGANIC-ASSOCIATED CPL
With success in the synthesis of various inorganic-associated CPL-active systems, their potential applications are also explored. Here, we list several examples related to sensors, security, electroluminescent devices, optical storage and asymmetric synthesis.
Sensors
Fluorescence sensing is an important analysis technique with many merits, such as rapidness, sensitivity, nondestructiveness and the ability to obtain diverse information regarding molecular interactions and microenvironments. Since fluorescence is a prerequisite for CPL, the change in fluorescent properties leads to the variation of CPL signals. Therefore, the principles of fluorescence sensing may be borrowed to design CPL sensors. For example, Suo et al.[82] reported a AuAg bimetallic cluster-based CPL sensing method by virtue of the fluorescence quenching effect [Figure 13A]. In the presence of metal ions (Sr2+ and K+), guanosine 5′-monophosphate can self-assemble into left- or right-handed helical nanofibers (g-fibers) with a width of 200 nm and a length of several micrometers. These g-fibers are used as chiral hosts to embed AuAg clusters stabilized with adenosine 5′-monophosphate. Consequently, helical nanostructured g-fiber-AuAg composites were obtained and showed CPL at ~ 475 nm under a 356 nm excitation, with a |glum| of 1-3×10-2. Because of the fluorescence quenching of AuAg NCs by L-Cys, g-fiber-AuAg NCs could be used as CPL sensors for the selective detection of L-Cys (in the range of 0.1 to 8 μmol L−1) with a limit of detection down to 95.7 nM, which is comparable to other common fluorometric and electrochemical methods.
Figure 13. Applications of inorganic-associated CPL-active systems. (A) CPL sensors of Cys. Reproduced with permission from Ref.[82]. Copyright (2020) American Chemical Society. (B) A security paint based on CPL-active Eu(III) lumino-glass. Reproduced with permission from Ref.[83]. (C) Electroluminescent device architecture containing chiral Eu(III) complexes and their circularly polarized electroluminescence (CPEL) spectra. Reproduced with permission from Ref.[84]. Copyright (2015) John Wiley and Sons. (D) Conceptual description of illuminating PL/CPL dual-mode rewritable optical storage and real images of PL/CPL dual-mode patterns. Reproduced with permission from Ref. [87]. Copyright (2020) Royal Society of Chemistry. (E) Schematic of enantioselective photopolymerization setup of HA. Reproduced with permission from Ref.[88]. Copyright (2019) American Chemical Society. PL: Photoluminescence; CPL: circularly polarized luminescenc.
Anticounterfeiting
Luminescent nanomaterials are widely employed in anticounterfeiting applications. To improve the security level, it is highly desirable that a luminescent material can possess more features. CPL-active materials encode hidden chiral fingerprint information into luminescence, which is not easily decoded by common optical read-out instruments. As a result, CPL offers a novel advanced anticounterfeiting technique with high expertise in chirality.
Kitagawa et al.[83] firstly synthesized europium complexes with chiral (+/-)-3-(trifluoroacetyl)camphor (+/-tfc) ligands and then cast them on a glass substrate together with the achiral glass promoter tris(2,6-dimethoxyphenyl)phosphine oxide (tmpo) ligand [Figure 13B]. After solvent evaporation, two enantiomeric Eu(+tfc)-tmpo(2) and Eu (−tfc)-tmpo(2) transparent films with an extra-large |glum| of 1.2 at a 594 nm emission (the magnetic dipole transition 5D0 → 7F1 of Eu3+) were produced. Furthermore, the authors demonstrated a CP light-based security device model to visualize the CPL image by a camera equipped with a linear polarizer, a rotatable λ/4 plate and a bandpass filter (594 nm). Eu(+tfc)-tmpo(2) was cast into a sun pattern and Eu(−tfc)-tmpo(2) into a moon. Without the λ/4 plate, both the sun and moon are observed. By rotating the λ/4 plate 90° clockwise or anticlockwise using an angle controller, the photographs of the emission using L-CP light (“sun”) and R-CP light (“moon”), respectively, are exhibited.
Electroluminescent devices
Electroluminescent devices, where an emissive layer is sandwiched between an anode and a cathode, are promising for artificial light sources. In these devices, the charge carriers (electrons and holes) are generated at the electrodes under an external voltage, transported to and then recombined at the emissive layer to produce electroluminescence. Various luminescent materials are used as the emissive layers to construct electroluminescent devices, such as organic, CD-based and perovskite-based light emitting diodes. Furthermore, these devices, which are capable of directly emitting CP light, are of significant interest for their promising potential in displays with high-resolution, 3D imaging ability, improved brightness and lower energy consumption.
Zinna et al.[84] used chiral europium complexes of CsEu(hfbc)4 (hfbc = 3-heptafluoro-butylyrylcamphorate) with the highest available glum (glum = 1.38 at 595 nm) as circularly polarized emitters in electroluminescent devices [Figure 13C]. To reduce the emission from the matrix, the Eu(III) complexes were blended with a polyvinylcarbazole host and a soluble additive of 1,3-bis[2-(4-tert-butylphenyl)-1,3,4-oxadiazo-5-yl]benzene to form the emitting layer. To minimize the internal reflection of the emitted light on the back electrode, a Ba/Al cathode (50% transmittance at 612 nm) was employed. With these strategies, a good polarization degree of emission (as measured by the dissymmetry factor of gEL in electroluminescence) is achieved, with gEL values of ±0.75 and −0.09/+0.15 for the Eu 5D0→7F1 (at 595 nm) and Eu 5D0→7F2 transitions (at 612 nm), respectively. Furthermore, by optimizing the emissive layer formulations and fine-tuning the architectures of the devices, the absolute value of gEL could even be increased to 1.0 (for Eu 5D0→7F1 at 595 nm)[85].
Han et al.[86] reported two enantiomeric gold clusters (Au4L4)n/(Au4D4)n consisting of a Au4 core and a chiral ligand, (S)-/(R)-4-isopropylthiazolidine-2-thione [abbreviated as L and D, structures shown in Figure 12A]. Through an aggregation- and crystallization-induced emission process, these aggregated gold nanoclusters showed a strong green emission centered at 507 nm with a photoluminescence quantum yield of 41.4% and intense CPL signals with |glum| of 7.0×10−3. These nanoclusters were employed as the chiral emissive layer to fabricate electroluminescent devices. The as-obtained device exhibited obvious green CPEL signals (driven at a voltage of 10 V) with a maximum external quantum efficiency of 1.5% and a dissymmetry factor (|gEL|) of 4-7×10−3.
Optical storage
The usage of CP light for optical storage is attractive due to the orthogonal characteristics of the polarization and uncomplicated optical system structures. Juan et al.[87] proposed an optical storage model based on 980 nm NIR-light triggered reversible CPL switching. A cholesteric liquid crystal with a self-organized helical superstructure was used as the host to load lanthanide (Yb3+, Er3+ or Tm3+)-doped NaYF4-based UCNPs and a chiral fluorescent photoswitch of 6 [Figure 13D]. When the UCNPs are irradiated by 980 nm at a low power (980-l), the 520 nm green emission (Yb3+ → Er3+ energy transfer process) dominated, while the 365 and 450 nm emissions (Yb3+ → Tm3+ energy transfer process) appeared at a high laser power density (980 h). Switch 6 showed a trans to cis photoisomerization under 520 nm light and the reverse isomerization upon irradiation at 365 or 450 nm. A strong emission peak at ~ 580 nm appeared for the trans state of switch 6 and greatly decreased for the cis state. Correspondingly, both the CPL signal and its absolute glum of ~ 580 nm decreased with low-power 980 nm NIR light, while partly recovering when exposed to high-power 980 nm light. Based on this switchable process controlled by laser power, a PL/CPL-encryption dual-mode rewritable optical storage device can be designed. The fluorescent dot array can be written on the cell using a photomask with a high-power laser and the information is erased under a low-power mode.
Asymmetric synthesis
CP light-induced asymmetric photochemical synthesis is an important type of absolute synthesis and even accounts for the homochirality origin on earth. Because of the unbalance between L- and R-CP emissions, CPL-active emitters are potential CP light sources to initiate enantioselective synthesis. Jin et al.[88] showed an up-conversion CPL to trigger the enantioselective polymerization of diacetylene [Figure 13E]. Achiral lanthanide-doped UCNPs (NaYF4:Yb/Er or NaYF4:Yb/Tm) were co-assembled with chiral lipid gelator L-/D-GAm to create helical nanotubes. After excitation with a 980 nm laser, the emission peaks of UCNPs-Tm are mainly located at 360 nm (1D2 → 3H6 transition), 476 nm (1G4 → 3H6) and 802 nm (3H4 → 3H6). Interestingly, the UV part of CPL from UCNPs-Tm could induce the polymerization of 2,4-heneicosadiynoic acid into polydiacetylene (PDA). PDA formed using L-GAm/UCNPs-Tm showed positive CD signals, while negative CD signals were found with D-GAm/UCNPs-Tm. Therefore, the molecular chirality of the gelator controlled the handedness of the UC-CPL from lanthanide ions and then subsequently regulated the enantioselective polymerization of diacetylene.
SUMMARY AND OUTLOOK
After a survey of the recent literature, it is without doubt that we have a toolbox to engineer multiple inorganic species (lanthanide ions, metal clusters, semiconductor NPs, carbon dots, perovskite NCs, nano-silica and so on) to undergo CPL, although they are not supposed to be chiral from our common sense. CPL in these inorganic materials is also found in various luminescence processes (e.g., up-conversion, TADF, phosphorescence and NIR emission) and is useful for many applications in sensing, optical storage, security and asymmetric synthesis. Because CPL is related to both chirality and luminescence, the achievements above prompt us to believe that two enantiomeric forms are allowed both in the structures and luminescence of inorganic substances and will open up new chirality-related horizons in inorganic chemistry and light science.
However, there are still many issues to be addressed to comprehend CPL in inorganics, including:
Reliable CPL data free from artifacts: CPL measurements are traditionally conducted on various homemade instruments and have recently been performed on some commercial CPL spectrophotometers. However, there are some artifacts possibly existing in as-measured CPL signals, which can be caused by many factors (e.g., instrumental limitations, linear dichroism effect and scattering/absorption phenomena). In particular, solid-state or anisotropic samples should be treated carefully. To this end, some experimental guides and data analysis methods can be found to obtain true CPL signals[89-93]. In addition, it is encouraging to give CD/PL spectra and some experimental details (e.g., sample concentrations and spectral test parameters) together with CPL data. It will be helpful to check the reliability and reproducibility of CPL signals.
Enhancement of glum: As shown in Table 1, the glum values of many kinds of inorganic-associated CPL are ~ 10-3 or lower, although some metal ions (e.g., lanthanide and chromium(III)) can potentially present high glum due to magnetically allowed but electric-dipole forbidden emissions. The small glum value means that the differences between LCP and RCP only account for 0.3% of the total emission. Hence, there is a significant potential to increase glum. Some enhancement strategies have been proposed, which are fulfilled by ingenious molecular design, energy transfer processes, plasmonic metal-assisted enhancement and assembly-/aggregation-induced CPL[19,94-97].
Evaluation indexes for CPL: Basically, CPL is based on luminescence and therefore the indexes for luminescence, such as quantum yield, brightness, excitation and emission wavelengths, should be taken into consideration together with glum. For example, Eu complexes showed a highest glum of 1.38 but with a very low emission quantum yield of less than 1.0%, which restricts their applications, especially in displays. The choice of excitation wavelength is also important in improving the CPL performance in some luminescent systems involved with energy transfer (e.g., metal complexes). Moreover, the relationships between glum and the luminescence parameters above are underexplored. Some researchers have suggested several indexes, such as a figure of merit (FM) (FM = Φ×glum, where Φ is the emission efficiency), asymmetric quantum efficiency (φa) (φa = IL/I0 or IR/I0, where I0 is the incident light intensity) and a brightness indicator (BF) (BCPL = ελ×Φ×glum/2, where ελ is the molar coefficient measured at the excitation wavelength)[21,98,99]. Nevertheless, the application of the indexes above is underexplored for different purposes.
Multiple-component CPL-active systems: In many cases, the system is constructed as a binary-component mode, i.e., only one chiral entity and only a luminescent one. However, it is not easy to achieve an ideal CPL effect (e.g., high glum, strong emission and tunable emission bands) only by two components. Consequently, multiple components can be introduced to manipulate the optical and chiral properties.
Revealing the detailed mechanisms for CPL: Although we know how to synthesize CPL-active materials, the underlying mechanism is not well explained in many publications. Often, the chirality transfer/induction mechanisms proposed for CD activity are roughly extended to CPL activity, but it is unsuitable in some cases because CD and CPL belong to two different transition processes. In addition, the theories and simulation methods addressing luminescence in a chiral environment are currently inadequate.
Exploration of CPL-based applications: The applications described here are still in their infancy but are inspirational nonetheless. With success in materials synthesis, exploration of their real applications is an important follow-up work.
DECLARATIONS
Authors’ contributionsPrepared and corrected the manuscript: Liu X, Jin RH
Availability of data and materialsNot applicable.
Financial support and sponsorshipThe research was supported in part by the JSPS KAKENHI, grant number: JP16H06515 (Coordination Asymmetry) and by the JSPS KAKEHI Grantin-Aid for Scientific Research (B), 19H02767.
Conflicts of interestBoth authors declared that there are no conflicts of interest.
Ethical approval and consent to participateNot applicable.
Consent for publicationNot applicable.
Copyright© The Author(s) 2022.
REFERENCES
1. Guijarro A, Yus M. The origin of chirality in the molecules of life: a revision from awareness to the current theories and perspectives of this unsolved problem. Cambridge: Royal Society of Chemistry; 2009.
2. Carreira EM, Yamamoto H. Comprehensive chirality. Elsevier: London; 2012. Available from: https://www.elsevier.com/books/comprehensive-chirality/yamamoto/978-0-08-095167-6. [Last accessed on 17 Mar 2022].
3. Stanciu CD, Hansteen F, Kimel AV, et al. All-optical magnetic recording with circularly polarized light. Phys Rev Lett 2007;99:047601.
4. Kim S, Kim E. Performance analysis of stereoscopic three-dimensional projection display systems. 3D Res 2010;1:1-16.
5. Kunnen B, Macdonald C, Doronin A, Jacques S, Eccles M, Meglinski I. Application of circularly polarized light for non-invasive diagnosis of cancerous tissues and turbid tissue-like scattering media. J Biophotonics 2015;8:317-23.
6. Krupová M, Kapitán J, Bouř P. Induced lanthanide circularly polarized luminescence as a probe of protein fibrils. ACS Omega 2019;4:1265-71.
7. Yang G, Zhang S, Hu J, Fujiki M, Zou G. The chirality induction and modulation of polymers by circularly polarized light. Symmetry 2019;11:474.
8. Qu A, Sun M, Kim JY, et al. Stimulation of neural stem cell differentiation by circularly polarized light transduced by chiral nanoassemblies. Nat Biomed Eng 2021;5:103-13.
9. Nishizawa N, Munekata H. Lateral-type spin-photonics devices: development and applications. Micromachines (Basel) 2021;12:644.
10. Zhan X, Xu FF, Zhou Z, Yan Y, Yao J, Zhao YS. 3D laser displays based on circularly polarized lasing from cholesteric liquid crystal arrays. Adv Mater 2021;33:e2104418.
11. Jiang S, Xiong X, Hu Y, et al. High-efficiency generation of circularly polarized light via symmetry-induced anomalous reflection. Phys Rev B 2015:91.
12. Zinna F, Pescitelli G, Di Bari L. Circularly polarized light at the mirror: caveats and opportunities. Chirality 2020;32:765-9.
13. Ranjbar B, Gill P. Circular dichroism techniques: biomolecular and nanostructural analyses- a review. Chem Biol Drug Des 2009;74:101-20.
14. MacKenzie LE, Pålsson LO, Parker D, Beeby A, Pal R. Rapid time-resolved circular polarization luminescence (CPL) emission spectroscopy. Nat Commun 2020;11:1676.
15. Riehl JP, Muller G. Circularly Polarized Luminescence Spectroscopy and Emission-Detected Circular Dichroism. In: Berova N, Polavarapu PL, Nakanishi K, Woody RW, editors. Comprehensive Chiroptical Spectroscopy. John Wiley & Sons, Inc.; 2011. p.65-90.
16. Sánchez-Carnerero EM, Agarrabeitia AR, Moreno F, et al. circularly polarized luminescence from simple organic molecules. Chemistry 2015;21:13488-500.
17. Ma JL, Peng Q, Zhao CH. Circularly polarized luminescence switching in small organic molecules. Chemistry 2019;25:15441-54.
18. Nitti A, Pasini D. Aggregation-induced circularly polarized luminescence: chiral organic materials for emerging optical technologies. Adv Mater 2020;32:e1908021.
19. Sang Y, Han J, Zhao T, Duan P, Liu M. Circularly polarized luminescence in nanoassemblies: generation, amplification, and application. Adv Mater 2020;32:e1900110.
20. Zhang DW, Li M, Chen CF. Recent advances in circularly polarized electroluminescence based on organic light-emitting diodes. Chem Soc Rev 2020;49:1331-43.
21. Deng Y, Wang M, Zhuang Y, Liu S, Huang W, Zhao Q. Circularly polarized luminescence from organic micro-/nano-structures. Light Sci Appl 2021;10:76.
22. Gong Z, Zhu X, Zhou Z, et al. Frontiers in circularly polarized luminescence: molecular design, self-assembly, nanomaterials, and applications. Sci China Chem 2021;64:2060-104.
23. Jiang S, Kotov NA. Circular polarized light emission in chiral inorganic nanomaterials. Adv Mater 2022:e2108431.
24. Sun M, Xu L, Qu A, et al. Site-selective photoinduced cleavage and profiling of DNA by chiral semiconductor nanoparticles. Nat Chem 2018;10:821-30.
25. Vinegrad E, Hananel U, Markovich G, Cheshnovsky O. Determination of handedness in a single chiral nanocrystal via circularly polarized luminescence. ACS Nano 2019;13:601-8.
26. Hananel U, Ben-Moshe A, Diamant H, Markovich G. Spontaneous and directed symmetry breaking in the formation of chiral nanocrystals. Proc Natl Acad Sci U S A 2019;116:11159-64.
27. Hao J, Li Y, Miao J, et al. Ligand-induced chirality in asymmetric CdSe/CdS nanostructures: a close look at chiral tadpoles. ACS Nano 2020;14:10346-58.
28. Krishnadas KR, Sementa L, Medves M, et al. Chiral functionalization of an atomically precise noble metal cluster: insights into the origin of chirality and photoluminescence. ACS Nano 2020;14:9687-700.
29. Ben-Moshe A, Govorov AO, Markovich G. Enantioselective synthesis of intrinsically chiral mercury sulfide nanocrystals. Angew Chem Int Ed Engl 2013;52:1275-9.
30. Morrow SM, Bissette AJ, Fletcher SP. Transmission of chirality through space and across length scales. Nat Nanotechnol 2017;12:410-9.
31. Kim H, Im SW, Kim RM, et al. Chirality control of inorganic materials and metals by peptides or amino acids. Mater Adv 2020;1:512-24.
34. Moran DM, Metcalf DH, Richardson FS. Chiroptical luminescence spectra of uranyl ion in cubic sodium tris(acetato)dioxouranate(1-) crystals. Inorg Chem 1992;31:819-25.
35. Mukhina MV, Maslov VG, Baranov AV, et al. Intrinsic chirality of CdSe/ZnS quantum dots and quantum rods. Nano Lett 2015;15:2844-51.
36. Duan Y, Han L, Zhang J, et al. Optically active nanostructured ZnO films. Angew Chem Int Ed Engl 2015;54:15170-5.
38. Gautier R, Klingsporn JM, Van Duyne RP, Poeppelmeier KR. Optical activity from racemates. Nat Mater 2016;15:591-2.
39. Zhao J, Zhang T, Dong XY, et al. Circularly polarized luminescence from achiral single crystals of hybrid manganese halides. J Am Chem Soc 2019;141:15755-60.
40. Kumar J, Thomas KG, Liz-Marzán LM. Nanoscale chirality in metal and semiconductor nanoparticles. Chem Commun (Camb) 2016;52:12555-69.
41. Tohgha U, Deol KK, Porter AG, et al. Ligand induced circular dichroism and circularly polarized luminescence in CdSe quantum dots. ACS Nano 2013;7:11094-102.
42. Maoz BM, Chaikin Y, Tesler AB, et al. Amplification of chiroptical activity of chiral biomolecules by surface plasmons. Nano Lett 2013;13:1203-9.
43. Ben-Moshe A, Maoz BM, Govorov AO, Markovich G. Chirality and chiroptical effects in inorganic nanocrystal systems with plasmon and exciton resonances. Chem Soc Rev 2013;42:7028-41.
44. Cao Z, Gao H, Qiu M, et al. Chirality transfer from sub-nanometer biochemical molecules to sub-micrometer plasmonic metastructures: physiochemical mechanisms, biosensing, and bioimaging opportunities. Adv Mater 2020;32:e1907151.
45. Lunkley JL, Shirotani D, Yamanari K, Kaizaki S, Muller G. Extraordinary circularly polarized luminescence activity exhibited by cesium tetrakis(3-heptafluoro-butylryl-(+)-camphorato) Eu(III) complexes in EtOH and CHCl3 solutions. J Am Chem Soc 2008;130:13814-5.
46. Wong H, Lo W, Yim K, Law G. Chirality and chiroptics of lanthanide molecular and supramolecular assemblies. Chem 2019;5:3058-95.
47. Staszak K, Wieszczycka K, Marturano V, Tylkowski B. Lanthanides complexes – Chiral sensing of biomolecules. Coordination Chemistry Reviews 2019;397:76-90.
48. Mackenzie LE, Pal R. Circularly polarized lanthanide luminescence for advanced security inks. Nat Rev Chem 2021;5:109-24.
49. Jiménez JR, Poncet M, Míguez-Lago S, et al. Bright long-lived circularly polarized luminescence in chiral chromium(III) complexes. Angew Chem Int Ed Engl 2021;60:10095-102.
50. Poncet M, Benchohra A, Jiménez J, Piguet C. Chiral chromium(III) complexes as promising candidates for circularly polarized luminescence. ChemPhotoChem 2021;5:880-92.
51. Ru Y, Ai L, Jia T, et al. Recent advances in chiral carbonized polymer dots: From synthesis and properties to applications. Nano Today 2020;34:100953.
52. Xu M, Wu X, Yang Y, et al. Designing hybrid chiral photonic films with circularly polarized room-temperature phosphorescence. ACS Nano 2020;14:11130-9.
53. Chekini M, Prince E, Zhao L, Mundoor H, Smalyukh II, Kumacheva E. Chiral carbon dots synthesized on cellulose nanocrystals. Adv Optical Mater 2020;8:1901911.
54. Li A, Zheng D, Zhang M, Wu B, Zhu L. Chirality transfer in carbon dot-composited Sol-gel systems for excitation-dependent circularly polarized luminescence. Langmuir 2020;36:8965-70.
55. Ru Y, Sui L, Song H, et al. Rational design of multicolor-emitting chiral carbonized polymer dots for full-color and white circularly polarized luminescence. Angew Chem Int Ed Engl 2021;60:14091-9.
56. Zhang M, Li K, Zang S. Progress in atomically precise coinage metal clusters with aggregation-induced emission and circularly polarized luminescence. Adv Optical Mater 2020;8:1902152.
57. Wu H, He X, Yang B, Li CC, Zhao L. Assembly-induced strong circularly polarized luminescence of spirocyclic chiral silver(I) clusters. Angew Chem Int Ed Engl 2021;60:1535-9.
58. Zhang MM, Dong XY, Wang ZY, et al. AIe triggers the circularly polarized luminescence of atomically precise enantiomeric copper(I) Alkynyl Clusters. Angew Chem Int Ed Engl 2020;59:10052-8.
59. Shi L, Zhu L, Guo J, et al. Self-assembly of chiral gold clusters into crystalline nanocubes of exceptional optical activity. Angew Chem Int Ed Engl 2017;56:15397-401.
60. Tao J, Li B, Lu Z, et al. Endowing zeolite lta superballs with the ability to manipulate light in multiple ways. Angew Chem Int Ed Engl 2020;59:19684-90.
61. Bobrovsky A, Mochalov K, Oleinikov V, et al. Optically and electrically controlled circularly polarized emission from cholesteric liquid crystal materials doped with semiconductor quantum dots. Adv Mater 2012;24:6216-22.
62. Yan J, Ota F, San Jose BA, Akagi K. Chiroptical resolution and thermal switching of chirality in conjugated polymer luminescence via selective reflection using a double-layered cell of chiral nematic liquid crystal. Adv Funct Mater 2017;27:1604529.
63. Wang C, Chen K, Xu P, Yeung F, Kwok H, Li G. Fully chiral light emission from Cspbx 3 perovskite nanocrystals enabled by cholesteric superstructure stacks. Adv Funct Mater 2019;29:1903155.
64. Cheng J, Hao J, Liu H, et al. Optically active CdSe-Dot/CdS-Rod nanocrystals with induced chirality and circularly polarized luminescence. ACS Nano 2018;12:5341-50.
65. Dong Y, Zhang Y, Li X, Feng Y, Zhang H, Xu J. Chiral perovskites: promising materials toward next-generation optoelectronics. Small 2019;15:e1902237.
66. Long G, Sabatini R, Saidaminov MI, et al. Chiral-perovskite optoelectronics. Nat Rev Mater 2020;5:423-39.
67. Ma S, Ahn J, Moon J. Chiral perovskites for next-generation photonics: from chirality transfer to chiroptical activity. Adv Mater 2021;33:e2005760.
69. Matsukizono H, Jin RH. High-temperature-resistant chiral silica generated on chiral crystalline templates at neutral pH and ambient conditions. Angew Chem Int Ed Engl 2012;51:5862-5.
70. Harada T, Yanagita H, Ryu N, et al. Lanthanide ion-doped silica nanohelix: a helical inorganic network acts as a chiral source for metal ions. Chem Commun (Camb) 2021;57:4392-5.
71. Sugimoto M, Liu XL, Tsunega S, et al. Circularly polarized luminescence from inorganic materials: encapsulating guest lanthanide oxides in chiral silica hosts. Chemistry 2018;24:6519-24.
72. Tsunega S, Jin RH, Nakashima T, Kawai T. Transfer of chiral information from silica hosts to achiral luminescent guests: a simple approach to accessing circularly polarized luminescent systems. Chempluschem 2020;85:619-26.
73. Liu P, Chen W, Okazaki Y, et al. Optically active perovskite Cspbbr3 nanocrystals helically arranged on inorganic silica nanohelices. Nano Lett 2020;20:8453-60.
74. Cai X, Du J, Zhang L, et al. Circularly polarized luminescence of single-handed helical tetraphenylethylene-silica nanotubes. Chem Commun (Camb) 2019;55:12176-9.
75. Zhang W, Chang H, Ai J, Che S, Duan Y, Han L. Spontaneous chiral self-assembly of achiral AIEgens into AIEgen-silica hybrid nanotubes. Chem Commun (Camb) 2019;55:14438-41.
76. Zhang S, Shi W, Rong S, Li S, Zhuang J, Wang X. Chirality evolution from Sub-1 nanometer nanowires to the macroscopic helical structure. J Am Chem Soc 2020;142:1375-81.
77. Yang X, Zhou M, Wang Y, Duan P. Electric-field-regulated energy transfer in chiral liquid crystals for enhancing upconverted circularly polarized luminescence through steering the photonic bandgap. Adv Mater 2020;32:e2000820.
78. Zinna F, Arrico L, Di Bari L. Near-infrared circularly polarized luminescence from chiral Yb(iii)-diketonates. Chem Commun (Camb) 2019;55:6607-9.
79. Frédéric L, Desmarchelier A, Favereau L, Pieters G. Designs and applications of circularly polarized thermally activated delayed fluorescence molecules. Adv Funct Mater 2021;31:2010281.
80. Han Z, Dong XY, Luo P, et al. Ultrastable atomically precise chiral silver clusters with more than 95% quantum efficiency. Sci Adv 2020;6:eaay0107.
81. Hao W, Li Y, Liu M. Endowing phosphor materials with long-afterglow circularly polarized phosphorescence via ball milling. Adv Optical Mater 2021;9:2100452.
82. Suo Z, Hou X, Chen J, et al. Highly chiroptical detection with gold-silver bimetallic nanoclusters circularly polarized luminescence based on G-quartet nanofiber self-assembly. J Phys Chem C 2020;124:21094-102.
83. Kitagawa Y, Wada S, Islam MDJ, et al. Chiral lanthanide lumino-glass for a circularly polarized light security device. Commun Chem 2020:3.
84. Zinna F, Giovanella U, Di Bari L. Highly circularly polarized electroluminescence from a chiral europium complex. Adv Mater 2015;27:1791-5.
85. Zinna F, Pasini M, Galeotti F, Botta C, Di Bari L, Giovanella U. Design of Lanthanide-based oleds with remarkable circularly polarized electroluminescence. Adv Funct Mater 2017;27:1603719.
86. Han Z, Zhao X, Peng P, et al. Intercluster aurophilicity-driven aggregation lighting circularly polarized luminescence of chiral gold clusters. Nano Res 2020;13:3248-52.
87. Juan A, Sun H, Qiao J, Guo J. Near-infrared light-controlled circularly polarized luminescence of self-organized emissive helical superstructures assisted by upconversion nanoparticles. Chem Commun (Camb) 2020;56:13649-52.
88. Jin X, Sang Y, Shi Y, et al. Optically Active Upconverting nanoparticles with induced circularly polarized luminescence and enantioselectively triggered photopolymerization. ACS Nano 2019;13:2804-11.
89. Longhi G, Castiglioni E, Koshoubu J, Mazzeo G, Abbate S. circularly polarized luminescence: a review of experimental and theoretical aspects. Chirality 2016;28:696-707.
90. Chen W, Tian Z, Li Y, Jiang Y, Liu M, Duan P. Long-persistent circularly polarized phosphorescence from chiral organic ionic crystals. Chemistry 2018;24:17444-8.
91. Tanaka H, Inoue Y, Mori T. Circularly polarized luminescence and circular dichroisms in small organic molecules: correlation between excitation and emission dissymmetry factors. ChemPhotoChem 2018;2:386-402.
92. Castiglioni E, Abbate S, Lebon F, Longhi G. Ultraviolet, circular dichroism, fluorescence, and circularly polarized luminescence spectra of regioregular poly-[3-((S)-2-methylbutyl)-thiophene] in solution. Chirality 2012;24:725-30.
93. Harada T, Kuroda R, Moriyama H. Solid-state circularly polarized luminescence measurements: theoretical analysis. Chemical Physics Letters 2012;530:126-31.
94. Kumar J, Nakashima T, Tsumatori H, Kawai T. Circularly polarized luminescence in chiral aggregates: dependence of morphology on luminescence dissymmetry. J Phys Chem Lett 2014;5:316-21.
95. Zhang C, Li S, Dong X, Zang S. Circularly polarized luminescence of agglomerate emitters. Aggregate 2021:2.
96. Zhao S, Yu Y, Zhang B, et al. Metal-Enhanced circularly polarized luminescence of self-assembled Au@SiO2 triangular nanoprisms and fluorophores in chiral cellulose nanocrystal films. Adv Optical Mater 2021;9:2100907.
97. Greenfield JL, Wade J, Brandt JR, Shi X, Penfold TJ, Fuchter MJ. Pathways to increase the dissymmetry in the interaction of chiral light and chiral molecules. Chem Sci 2021;12:8589-602.
98. Yao L, Niu G, Li J, et al. Circularly polarized luminescence from chiral tetranuclear copper(I) iodide clusters. J Phys Chem Lett 2020;11:1255-60.
Cite This Article

How to Cite
Download Citation
Export Citation File:
Type of Import
Tips on Downloading Citation
Citation Manager File Format
Type of Import
Direct Import: When the Direct Import option is selected (the default state), a dialogue box will give you the option to Save or Open the downloaded citation data. Choosing Open will either launch your citation manager or give you a choice of applications with which to use the metadata. The Save option saves the file locally for later use.
Indirect Import: When the Indirect Import option is selected, the metadata is displayed and may be copied and pasted as needed.
About This Article
Copyright
Author Biographies
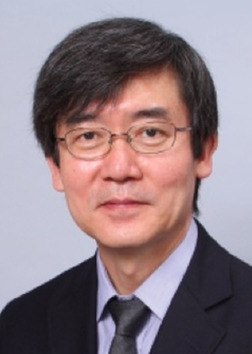
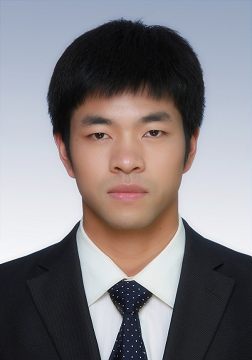
Comments
Comments must be written in English. Spam, offensive content, impersonation, and private information will not be permitted. If any comment is reported and identified as inappropriate content by OAE staff, the comment will be removed without notice. If you have any queries or need any help, please contact us at [email protected].