Genetics of Huntington’s disease and related disorders: beyond triplet repeats
Abstract
Huntington’s disease (HD) is an autosomal dominant neurodegenerative disorder due to a triplet repeat expansion in the HTT gene. The identification of this gene variation was a lengthy process, but it has since provided an explanation of clinical observations including the variability in age at onset observed across generations (phenomenon of anticipation). Further molecular genetic investigations have allowed the discovery of genes modifying the phenotype presenting differences in terms of age at the onset and course of the disease. Pathogenic gene variations have also been found in other diseases with a similar presentation, such as HD, allowing precise genetic diagnosis. This narrative review examines these data in the context of their historical development. Their implication in our understanding of these disorders and treatment modalities is also highlighted.
Keywords
INTRODUCTION
Huntington’s disease (HD) is a complex syndrome with chorea and other motor signs including dystonia, bradykinesia, rigidity, tremor, balance and gait disorder, and myoclonus, which are present in variable intensity during the course of the disease[1]. The name previously used as a diagnostic label, Huntington’s chorea, covered only one feature of the disorder, and chorea is often not the most disturbing symptom for the patient. Cognitive and psychiatric troubles predominate and may cause more significant impairment in daily life. The former include executive function disorders, which may impair work and quality of life early in the disease, even before motor changes appear. Other aspects of cognitive function may also be impaired, such as psychomotor and information processing speed, emotion recognition, social cognition, visuospatial processing, visuomotor integration, attention, apathy, typically progressive, and, to a lesser extent, memory. Psychiatric problems include depression, which may improve over the course, anxiety, irritability, compulsive behavior, perseveration, and paranoia. The diagnosis is made after the recognition of the typical symptoms and signs in the context of a family history and/or after genetic testing following appropriate genetic counseling. In sporadic cases, or when the family history is not conclusive, additional laboratory investigations and brain imaging may be needed to search for other causes. The disorder is caused by an expansion of a triple repeat (CAG) in the gene encoding huntingtin on chromosome 4p16[2].
Beyond HD, other hereditary disorders have similarities in their presentation[3]. The so-called “Huntington-like diseases” (HDL) or “HD phenocopies” are found in about 1% of patients with HD presentation having no CAG expansion[4]. Guidance to assess these disorders has been recently published[5].
The earliest and most prominently affected part of the brain in HD and most related disorders (phenocopies) is the striatum. Group-wise volumetric analysis allows the detection of changes as early as a decade before motor onset[6]. The molecular pathology is highly complex, with an accumulation of abnormal proteins and sometimes the formation of inclusion bodies accompanied by inflammation leading to cell degeneration. Many other aspects of the pathogenic process, including loss of protein function, have been discovered in the context of an extensive number of cellular interactions with other nucleotides, proteins, and organelles. This knowledge has paved the way for the development of translational models of the disease, which has in turn led to the initiation of a number of clinical trials[7].
Therapy for HD and phenocopies is currently purely symptomatic[8]. The multiple disturbances lead to a progressive function impairment from inability to work in the usual occupational context to gradual loss of independence and need for care up to comprehensive multidisciplinary nursing settings. Complications such as pneumonia after aspiration due to swallowing impairments, skeletal and head trauma after falls, and side effects of drugs accumulate over the course of the disease. The trajectory of the relentlessly progressive disease typically culminates in death about 20 years after diagnosis.
The present narrative review is designed to examine the evolution of genetic knowledge about HD and related disorders, from gene discovery to findings on genetic variations associated with the phenotype. The pathogenic gene variations are presented. Age at the onset of HD and other disorders with repeat expansion is correlated with the number of repetitive elements. New data suggesting that repeat expansion may also influence phenotype in other diseases are presented. The topic of phenotype variation not explained by such intrinsic aspects follows, including a discussion of the present status of genetic modifiers. Further, epigenetic changes are also examined. Some of these discoveries have led to the implementation of clinical trials aimed at slowing the neurodegenerative process. Examples of such trials are presented.
The search for the pathogenic variations in HD
In his description of the different types of diseases with chorea, including infections, and rheumatism, and inflammation, George Huntington described the hereditary form with a typical adult phenotype[9]. Even before the discovery of the Mendelian rules of inheritance, he described the occurrence of families with affected individuals in all generations. He also recognized the fact that people who live without symptoms long enough after the typical age at onset would not transmit the disease to their children. The development of the search for the gene mutated in HD took a long time after the autosomal dominant inheritance of the disease was established and has been extensively described[10]. The search for the gene locus using blood group and protein markers was unsuccessful, but the discovery of restriction fragment length polymorphisms and exon trapping led to the cloning of the gene[2]. A polymorphic CAG repeat was found in exon 1 with expansion on disease chromosomes. Normal triplet numbers are below 36, while 40 or more triplets are associated with a fully penetrant disorder, and numbers falling between the two are linked to a lower penetrance[11]. Individuals with intermediate ranges, specifically between 27 and 36, will not be affected but may have children with higher triplet counts. Age at disease onset varies across generations, typically earlier in subsequent ones, particularly in paternal transmission[12]. This concept of anticipation had already been mentioned early in the 20th century[13]. The age at onset, defined mainly by the first manifestation of typical motor signs, is inversely correlated with the number of CAG repeats[14]. The CAG repetitive sequence is interrupted by CAA triplets, which also code for glutamine; however, the age at onset is correlated with the uninterrupted CAG repeat numbers and not with the polyglutamine size[15].
Other diseases with Huntington-like presentation
HD-like disease 1 (HDL1)[16] is a rare disease due to 6 or 8 octapeptide repeat insertions in the prion protein (PRPN) gene. However, other phenotypes have been seen with the same octapeptide numbers. Furthermore, other PRPN variations[17] may lead to a range of different presentations, including Creutzfeld-Jacob disease and Gerstmann-Sträussler-Scheinker syndrome. Moreover, interactions between gene variations associated with heterogeneous clinical manifestations are also observed in acquired forms of the disease and the search for a comprehensive mechanistic understanding is still ongoing. CTG repeat expansions in the junctophilin-3 (JPH3) gene result in HD-like disease 2 (HDL2) in people of African descent[18]. The pathogenic variation is highly penetrant. A common haplotype has been traced back to 2,000 years, suggesting a founder effect[19]. Age at onset is also inversely correlated with the number of repeats[20]. The phenotype is variable, including asymptomatic carriers. HDL3 has been used as a designation for another very rare recessive disorder, suggested to be linked to 4 p15.3[21]. The rarity of these diseases makes further differential analysis of gene and phenotype correlation challenging.
Chorea, together with psychiatric and cognitive disturbances, is part of the variable phenotype found in SCA17, due to TATA-binding protein gene (ATX/TBP) CAA/CAG repeat expansions[22]. HDL4 has been used for cases with prominent chorea[23]; the disease with its variable presentations is now called ATX-TBP[24]. A systematic review using the methodology established by the Movement Disorders Society (MDS) to assess genotype-phenotype correlation suggests a phenotype-independent inverse correlation between age at onset and the number of repeats on the expanded allele, with no contribution from the normal allele size[24]. No effect of CAA interruptions on the expanded repeat was found. Reduced penetrance was found with repeats between 41 and 45, with pure parkinsonism being more frequent than in the group with higher numbers leading to full penetrance. Chorea was more frequent in the full penetrance group with fewer repeats. Pure chorea without other movement disorders such as ataxia or parkinsonism was rare.
An autosomal dominant inherited hexanucleotide repeat (GGGGCC) expansion in the C9orf72 gene was first described in large families with fronto-temporal lobar degeneration (FTLD) associated with amyotrophic lateral sclerosis (ALS)[25,26]. Further studies expanded the phenotype, for example, in a large UK cohort including 2,974 cases with Alzheimer’s disease (AD), ALS, FTLD, sporadic Creutzfeldt-Jakob disease (sCJD), HD phenocopies, and non-specific neurodegeneration, compared with 7,579 controls[27]. Large expansions in c9orf72 (more than 32 repeats) were found in 85 cases, with frequencies of 0.081 in ALS, 0.075 in FTLD, 0.02 in non-specific neurodegeneration, 0.017 in HD phenocopies, 0.012 in AD, and 0.002 in sCJD. There was a wide variation in the number of repeats, typically above 400 and, in rare instances, up to 4,400. The mean age at onset was 54.6 years, which is similar for all presentations and correlated with the modal point of expansion size[27]. Expansions were found in 2% of the 514 patients included in a HD phenocopy cohort[28]. Chorea, when present, may be associated with other movement disorders, including dystonia, myoclonus, tremor, and rigidity[28]. In a thorough meta-analysis with a systematic review of HD-like syndromes, including 1,123 cases, 1% carried the C9orf72 expansion, and 3% had intermediate alleles[29]. The age at onset was 46.8 years. Besides chorea, the predominant symptoms were psychiatric disorders and parkinsonism. The mechanisms underlying such a variable of phenotype remain unclear. RNA and protein toxicity, as well as protein loss-of-function, have been suggested to trigger neurodegeneration[30].
Chorea, most commonly associated with other neurological or even systemic signs and symptoms, may be present in dentato-rubro-pallido-luysian atrophy (DRPLA)[31], a triplet repeat expansion disorder in exon 5 of atrophin 1, which is mainly found in the Japanese population. Other disorders with sometimes predominant chorea include McLeod syndrome, chorea-acanthocytosis, neuroferritinopathy, and RNF216-mediated neurodegeneration[25]. Recently discovered genes also associated with chorea include AMPA receptor outer-core protein, FRRS1L[32], and ADCY5[33], and it is to be expected that others will be discovered in the context of comprehensive molecular-genetic studies aimed at assessing rare neurological disorders.
Modifying genetic factors in HD
The number of triplet repeats in HD only explains about 60% of the onset age variation[34]. In order to explore the genetic component of the remaining onset age variance not explained by the number of triplet repeats, studies were performed to examine its association with other gene sequence variations. An association of age at onset with variations in the GluR6 has been suggested[35], but the finding could not be replicated[36]. This was also the case for a number of further suggestions [Table 1]. One of the shortcomings of most of these studies was the low number of cases and the insufficient correction for multiple comparisons. Furthermore, they were biased by a preconceived choice of the candidate genes. Unbiased studies avoid these shortcomings, but they require a large number of participants. Genome-wide association studies have been performed by several groups including the GeM-HD consortium [Table 2]. Large patient cohorts have been used, including Pharos and Cohort, run by the Huntington Study Group (HSG), and the EHDN Registry, expanded in Enroll-HD, both sponsored by the CHDI Foundation. An initial association study of age at motor onset by the GeM-HD consortium involving around 4,000 participants found three associated loci[37]. These were confirmed by a replication study with about 3,000 participants. Among the identified loci, two loci, situated on chromosomes 15 and 8, were linked to an earlier age at motor onset, leading to a respective advancement of 6 years and 1.6 years in motor onset; conversely, another locus, also on chromosome 15, was associated with a delay of 1.4 years in motor onset[15]. A larger follow-up study with more than 9,000 individuals confirmed and further extended the findings [Table 2][38]. These findings suggest a DNA maintenance modification mechanism by genes, including MSH3. A variation of more than 6 years is quite relevant for the patients, even if the disease presents in a subtle manner. These data are also relevant in genetic counseling as they may allow a general explanation that there are genetic factors explaining variability. The counselee does not need to expect similarities with age at onset in other members of his family, even with the same number of CAG repeats. In the future, it might be possible to actually provide some figures on the predicted age at onset in the clinical setting, but more data are needed.
Chronology of published association studies with candidate genes and age at motor onset in Huntington’s disease
Year of publication | Gene | N of patients | Association | Author |
1997 | GRIK2 | 293 | Positive | Rubinsztein et al.[35] |
1999 | GRIK2 | 258 | Positive | MacDonald et al.[75] |
2001 | TCERG1 | 432 | Positive | Holbert et al.[76] |
2002 | UCHL1 | 138 | Positive | Nazé et al.[77] |
2005 | GRIN2A | 167 | Positive | Arning et al.[78] |
2006 | GRIK2 | 622 | Positive | Zeng et al.[79] |
2006 | UCHL1 | 946 | Positive | Metzger et al.[80] |
2006 | APOE4, ACE | 114 | Positive | Panegyres et al.[81] |
2006 | BDNF | 557 | Negative | Kishikawa et al.[82] |
2007 | GRIN2B, GRING2A | 250 | Positive | Arning et al.[83] |
2007 | Panel of 9 candidate genes | 443 | Positive for GRIN2A | Andresen et al.[84] |
2008 | HAP1 | 980 | Positive | Metzger et al.[85] |
2008 | panel of 304 candidate genes | 151 | Positive for ASK1 and MAP2K6 | Arning et al.[86] |
2009 | PGC-1alpha | 447 | Positive | Weydt et al.[87] |
2009 | ADORA2A | 791 | Positive | Dhaenens et al.[88] |
2010 | Atg1 | 952 | Positive | Metzger et al.[89] |
2012 | Kalirin | 680 | Negative | Tsai et al.[90] |
2022 | TCERG1 | 506 | Positive | Lobanov et al.[91] |
2012 | GRIK2 | 2,911 | Negative | Lee et al.[36] |
2012 | HAP1 | 298 | Negative | Karadima et al.[92] |
2013 | CNR1 | 473 | Positive | Kloster et al.[93] |
2015 | Panel of 20 candidate genes | 284 | Positive for E2F2 | Valcárcel-Ocete et al.[94] |
Search for modifying genes associated with the residual variation in the age at onset not explained by the number of CAG repeats: genome-wide association studies
Year of publication | Number of cases | Association | Gene | Author |
2003 | 695 | 4p16, 6p21-23 6q24-26 | Not determined | Li et al.[95] |
2006 | 836 | 6q23-24 18q22 | Not determined | Li et al.[96] |
2008 | 1,332 | 2p25 2q35 4p16 4q21 5p14 5q32 6q22 12q15 19p13 | Not determined | Gayán et al.[97] |
2015 | 4,082 | Locus on Ch 8 Locus on Ch 15 Locus on Ch 3 (?) | Not determined | GenM-HD[37] |
2017 | 3,314 | Locus on Ch 8 Locus on Ch 15 Locus on Ch 3 | MLH1 Other not determined | Lee et al.[38] |
2019 | 9,064 | Locus on Ch 1 Locus on Ch 2 Locus on Ch 3 Locus on Ch 3 Locus on Ch 5 Locus on Ch 5 Locus on Ch 5 Locus on Ch 7 Locus on Ch 8 Locus on Ch 11 Locus on Ch 11 Locus on Ch 12 Locus on Ch 15 Locus on Ch 15 Locus on Ch 15 Locus on Ch 15 Locus on Ch 16 Locus on Ch 18 Locus on Ch 18 Locus on Ch 19 Locus on Ch 19 | Not determined PMS1 MLH1 Not determined MSH3, DHFR Not determined TCERG1 PMS2 RRM2B, UBR5 CCDC82 SYT9 Not determined Not determined FAN1 Not determined Not determined GSG1L Not determined Not determined LIG1 Not determined | GenM-HD[15] |
2021 | 9,058 | Locus on Xq12 (suggestive) | Meosin? | Hong et al.[46] |
TRACK-HD, a prospective three-year cohort study including controls, premanifest and early manifest gene carriers has collected an extensive set of phenotype data enabling precise disease progression description[6]. Data from this study, combined with those from the EHDN Registry study[39], have allowed the assessment of genetic modifiers of disease progression. A signal was found on chromosome 5, with MSH3 being the most significant contribution among the possible genes, even after correction for age at motor onset[40]. MSH3 variations, specifically in exon 1, are related to somatic CAG expansion[41]. Expansion occurs in somatic cells, such as striatum[42]and blood[43]. This phenomenon appears to be age-related in animal models[44] and in the human brain[43]. Based on this information, a two-step sequential component model of disease pathogenesis[45] has been suggested. Mechanisms related to the inherited CAG repeat expansion underlie the disease with further modification of onset and progression by variable somatic expansion. To complete the search, a GWAS was performed on the X chromosome. No significant signal was found, but a signal for a possible association at Xq12 was suggested, with moesin found in the region[46].
In order to examine the genetic factors influencing other aspects of the phenotype, including behavior and cognition, a comparison with modifying factors found in psychiatric disorders, particularly depression and schizophrenia, was performed[47]. Data from association studies in psychiatric and cognitive phenotypes were used to construct polygenic scores, which were then used to test whether they also showed a correlation with the HD phenotypes in a cohort of more than 5,000 patients. Associations were found between polygenic scores for major depression and increased risk of depression in HD, and between polygenic scores for apathy and cognitive impairment and a reduced polygenic risk score for intelligence[47].
Modifying genetic factors in HD-related disorders
The precise mechanisms underlying phenotypic variations in ATX-TBP are not known, but complex interactions between TBP and the STUB1 genes seem to modify the phenotype[48], at least in patients with intermediate alleles. Furthermore, chorea frequency in the full penetrance group was less prominent in Asians, pointing to ethnic differences[24]. It is interesting to note that HD is less common in East Asians, and that interactive mechanisms, possibly linked to variable haplotypes, may play a role, as exemplified in a recent case involving expansion in both HTT and TBP[49].
Data that enable the understanding of the large phenotype variation in C9orf72-related disorders are still scarce. A 9–base pair duplication in the 2-gene ATXN2 sense/antisense region has been found in a Swedish family with spinocerebellar ataxia 3 and parkinsonism with an age at onset unexplained by the concomitant presence of ATXN2 intermediate alleles. Similarly, this duplication was found to be correlated with earlier age at onset in ALS due to C9orf72[50]. However, no such data are available for chorea linked to C9orf72 expansion.
No data on potential modifier genes are available for the other HD phenocopies. The reason is the low number of cases known so far. However, it may be possible to assess the effect of the already established modifiers in HD in smaller populations.
A renewed interest in haplotypes
The haplotype studies, which had been carried out in the search for the HD gene, have been much greatly enhanced and enriched by the profound technical developments in molecular genetic studies. This has enabled them to be used to assess further aspects of the disease. A comprehensive study of HD patients of European ancestry disclosed a set of 22 single nucleotide polymorphisms (SNP), which could define specific haplogroups[51]. These could be further refined[52] and have been used to assess cohorts from different populations. A systematic review has found that higher frequencies of two haplotypes (referred to as A1 or A2) are found in populations with a high HD prevalence[53]. The question then arises of whether populations with lower incidence have a different haplotype specifically linked to the disease. In our study on a Chinese HD cohort, the A1 and the A2 haplotypes were absent, which is in line with the low prevalence of the disease in East Asia. In a search for a specific haplogroup, a newly defined haplogroup I was found on more than 61% of HD chromosomes compared to 34% in control ones[54]. In a large kindred from Oman with 54 manifest HD, haplotype analysis was able to trace ancestry back to sub-Saharan Africa[55]. Furthermore, association of this haplotype with large CAG repeat expansions seems to be related to juvenile-onset HD.
High haplotype diversity may be due to population admixture, as suggested in a study of a Spanish cohort that also found haplotype-dependent germline instability[56]. A similar population-specific modification linked to a particular haplotype has been found in individuals with African ancestry[57]. Specific SNP are important for targeted gene therapy, for example, with CRISPR-Cas9[58], and for the use of antisense oligonucleotides to selectively decrease mutant allele without altering the expression of the normal allele[59]. A more recent study using long-read sequencing has found one single SNP in 30% of HD cases[60]. Combinatorial SNP targeting of these individual SNPs would allow for 57% of HD cases to be candidates for allele-specific targeting[60].
The contribution of epigenetics
Variations in gene expression not encoded by nucleotide sequences[61] are inherited through epigenetic mechanisms. These may modify phenotypic presentation and include DNA methylation, histone modification, and interaction with non-coding mRNA. So, research into these mechanisms in HD has been intensified. A number of changes in methylation have been found, for example, arginine methylation of RNA-binding proteins, which is also impaired in HD, leading to abnormal interactions with mutant huntingtin[62]. Changes in several microRNAs have been suggested to modulate the HP phenotype[63]. Brain tissue DNA methylation levels increase with age and an acceleration of methylation has been reported in tissues from several brain region in HD64, this may also modify the phenotype[64]. An interplay between epigenetic and genetic factors has been suggested based on common findings in triplet disorders, further linking triplet number instability to altered function of repair genes and to methylation[65]. Further research combining high-throughput assessment of epigenetic processes in cells and tissues from patients and animal models of HD promises future insights into the molecular pathogenesis of the disease, which may open up new therapeutic avenues.
CAG repeats as modifying factors in other conditions
CAG repeats are also variable within the normal ranges found in non-affected people.
A study involving five European-based cohorts with a total of more than 16,000 participants examined CAG triplets in 9 genes, including AR, ATN1, ATXN1, ATXN2, ATXN3, ATXN7, CACNA1A, HTT, and TBP were examined. Intermediate ranges include CAG numbers that fall below those associated with disease but carry a higher risk of being associated with amplification-induced expansions leading to disease in the next generation. Intermediate ranges were found in 10% of cases, while pathologically low ranges, typically associated with late onset and/or slower progression of the respective disease, were found in 1.3%[66].
Based on these findings, several studies have examined the correlation between CAG repeats and other disorders or phenotypes. Higher numbers within the normal range are associated with negative changes in the lipoprotein profile[67]. An inverse correlation between body mass index and the number of CAG repeats was found in the TBP gene and in 5 other genes[68]. Cognitive function in old age is related to the number of CAG repeats in HTT, TBP, and AR (encoding the androgen receptor, with increased CAG numbers in spinal and bulbar muscular atrophy)[69]. As the authors mention, this study has limitations. Findings in this homogenous, Northern European population cannot be generalized to other groups. Additionally, it is possible that participants might have not yet reached the age at which they would be symptomatic. Moreover, the increase in CAG repeats has been associated with a lifetime risk of depression in a non-linear way[70]. These results must be confirmed by independent replication studies, but they open up interesting avenues to better understand genotype-phenotype correlations.
Openings for neuroprotective approaches
Advances in our understanding of the genetic background of HD and related disorders have facilitated the development of strategies to modify the course of the neurodegenerative process. This is particularly true for HD, with several clinical trials now pursuing the strategy of decreasing the expression of HTT, which leads to a decrease in protein. A first study using antisense oligonucleotides (ASO) has been completed and data have recently been published[71]. The study involved 791 participants in three equal groups, including one with Tominersen at a dose of 120 mg, one with alternate Tominersen and placebo, and the third with placebo delivered intrathecally every 8 weeks by lumbar puncture. Target engagement was confirmed by a decrease in Huntingtin protein in the cerebrospinal fluid. However, clinical outcome measures worsened in the Tominersen group in a dose-dependent manner, more than in the placebo group. This led to a cessation of drug application while the study was continued in order to obtain follow-up data. In a post hoc analysis, a subgroup with clinical improvement was identified, which led the company to start a new double-blind phase II study to assess safety, biomarkers, and efficacy with narrower inclusion criteria (Roche, Generation HD2, NCT05686551). These include age between 25-50 years and a disease score defining late prodromal and early disease states. This approach decreased expression in both the pathological and the normal alleles. Allele-selective approaches are being developed in order to decrease only pathological allele expression. The ASOs used in this approach target an additional SNP, which is found only on the mutated allele. Such a study is now in progress (Wave, Select-HD, NCT05032196). Intrathecal delivery may not be sufficient to achieve enough brain penetration and may need to be performed on a regular basis. In order to overcome these two problems, another approach is to directly insert viral vectors with interfering RNA sequences into appropriate brain targets by stereotactic delivery. Such a study using AAV5-miHTT is now in progress at several centers in Europe and North America (Uniqure, AMT-130 NCT04120493). Approaches to target genes associated with phenotype modification are also in preclinical preparation. Similar approaches are underway in other triplet disorders, allowing synergistic approaches, such as the use of an ASO that directly targets the expanded CAG sequence in different disorders. A Phase 1/2a, open-label trial has been started with the aim to investigate the safety, tolerability, pharmacokinetics, and pharmacodynamics of such an ASO (Vico Therapeutics, VO659, NCT05822908). Patients with SCA1, SCA3, and HD receive multiple ascending ASO doses by intrathecal administration.
It is expected that future trials will use data from the genetic modifier studies for stratification. For example, including the size of the uninterrupted CAG sequence and the number of CAA in HTT would provide means for more homogeneous groups with improved power and the need for a smaller number of participants[72].
CONCLUSION
So far, the data presented have been helpful in improving precise and more comprehensive diagnostic procedures, specifically in recent years in rare HD phenocopies. They may also be useful in better predicting the age at onset, but more data are needed in this respect.
Many of the studies cited above have been performed on people of European descent. One reason for this is that the prevalence of HD is higher in Europe as compared to estimates in Asia and Africa. Fortunately, other cohorts are now under construction, for example, in Korea[73]. So far, the numbers of CAG repeats in healthy and diseased people in Korea[73] and in China[74] are the same as in people of European ancestry.
A long research path had to be followed in order to find the gene variation in HD. However, thanks to new molecular techniques and intensive clinical work leading to the establishment of large cohorts, the genetic background of the disease is now better understood. This has led to the development of clinical trials aimed at modifying the course of the disease, which are now being implemented. Further work will be needed to better understand the environmental and epigenetic contributions to the phenotype, and this will hopefully open additional therapeutic avenues.
DECLARATIONS
Authors’ contributions
The author contributed solely to the article.
Availability of data and materials
Not applicable.
Financial support and sponsorship
None.
Conflicts of interest
The author declared that there are no conflicts of interest.
Ethical approval and consent to participate
Not applicable.
Consent for publication
Not applicable.
Copyright
© The Author(s) 2024.
REFERENCES
2. MacDonald ME, Ambrose CM, Duyao MP, et al. A novel gene containing a trinucleotide repeat that is expanded and unstable on Huntington’s disease chromosomes. Cell 1993;72:971-83.
4. Kremer B, Goldberg P, Andrew SE, et al. A worldwide study of the Huntington’s disease mutation: the sensitivity and specificity of measuring CAG repeats. N Engl J Med 1994;330:1401-6.
5. Nguyen QTR, Ortigoza Escobar JD, Burgunder JM, et al. Combining literature review with a ground truth approach for diagnosing Huntington’s disease phenocopy. Front Neurol 2022;13:817753.
6. Tabrizi SJ, Scahill RI, Owen G, et al. TRACK-HD Investigators. Predictors of phenotypic progression and disease onset in premanifest and early-stage Huntington’s disease in the TRACK-HD study: analysis of 36-month observational data. Lancet Neurol 2013;12:637-49.
7. Ferguson MW, Kennedy CJ, Palpagama TH, Waldvogel HJ, Faull RLM, Kwakowsky A. Current and possible future therapeutic options for Huntington’s disease. J Cent Nerv Syst Dis 2022;14:11795735221092517.
8. Bachoud-Lévi AC, Ferreira J, Massart R, et al. International guidelines for the treatment of Huntington’s disease. Front Neurol 2019;10:710.
9. Huntington G. On Chorea. 1872. Available from: https://hdsa.org/wp-content/uploads/2017/08/On-Chorea.pdf. [Last accessed on 27 Feb 2024].
10. Bates GP. History of genetic disease: the molecular genetics of Huntington disease - a history. Nat Rev Genet 2005;6:766-73.
11. Rubinsztein DC, Leggo J, Coles R, et al. Phenotypic characterization of individuals with 30-40 CAG repeats in the Huntington disease (HD) gene reveals HD cases with 36 repeats and apparently normal elderly individuals with 36-39 repeats. Am J Hum Genet 1996;59:16-22.
12. Kremer B, Almqvist E, Theilmann J, et al. Sex-dependent mechanisms for expansions and contractions of the CAG repeat on affected Huntington disease chromosomes. Am J Hum Genet 1995;57:343-50.
13. Monckton DG. The contribution of somatic expansion of the CAG repeat to symptomatic development in Huntington’s disease: a historical perspective. J Huntingtons Dis 2021;10:7-33.
14. Duyao M, Ambrose C, Myers R, et al. Trinucleotide repeat length instability and age of onset in Huntington’s disease. Nat Genet 1993;4:387-92.
15. Modifiers of Huntington’s Disease (GeM-HD) Consortium. CAG repeat not polyglutamine length determines timing of Huntington’s disease onset. Cell 2019;178:887-900.e14.
16. Kim MO, Takada LT, Wong K, Forner SA, Geschwind MD. Genetic PrP prion diseases. Cold Spring Harb Perspect Biol 2018;10:a033134.
17. Nafe R, Arendt CT, Hattingen E. Human prion diseases and the prion protein - what is the current state of knowledge? Transl Neurosci 2023;14:20220315.
18. Margolis RL, Holmes SE, Rosenblatt A, et al. Huntington’s disease-like 2 (HDL2) in North America and Japan. Ann Neurol 2004;56:670-4.
19. Krause A, Mitchell C, Essop F, et al. Junctophilin 3 (JPH3) expansion mutations causing Huntington disease like 2 (HDL2) are common in South African patients with African ancestry and a Huntington disease phenotype. Am J Med Genet B Neuropsychiatr Genet 2015;168:573-85.
20. Margolis RL, O’Hearn E, Rosenblatt A, et al. A disorder similar to Huntington’s disease is associated with a novel CAG repeat expansion. Ann Neurol 2001;50:373-80.
21. Kambouris M, Bohlega S, Al-Tahan A, Meyer BF. Localization of the gene for a novel autosomal recessive neurodegenerative Huntington-like disorder to 4p15.3. Am J Hum Genet 2000;66:445-52.
22. Nakamura K, Jeong SY, Uchihara T, et al. SCA17, a novel autosomal dominant cerebellar ataxia caused by an expanded polyglutamine in TATA-binding protein. Hum Mol Genet 2001;10:1441-8.
23. Stevanin G, Brice A. Spinocerebellar ataxia 17 (SCA17) and Huntington’s disease-like 4 (HDL4). Cerebellum 2008;7:170-8.
24. Rossi M, Hamed M, Rodríguez-Antigüedad J, et al. Genotype-phenotype correlations for ATX-TBP (SCA17): MDSGene systematic review. Mov Disord 2023;38:368-77.
25. DeJesus-Hernandez M, Mackenzie IR, Boeve BF, et al. Expanded GGGGCC hexanucleotide repeat in noncoding region of C9ORF72 causes chromosome 9p-linked FTD and ALS. Neuron 2011;72:245-56.
26. Renton AE, Majounie E, Waite A, et al. ITALSGEN Consortium. A hexanucleotide repeat expansion in C9ORF72 is the cause of chromosome 9p21-linked ALS-FTD. Neuron 2011;72:257-68.
27. Beck J, Poulter M, Hensman D, et al. Large C9orf72 hexanucleotide repeat expansions are seen in multiple neurodegenerative syndromes and are more frequent than expected in the UK population. Am J Hum Genet 2013;92:345-53.
28. Hensman Moss DJ, Poulter M, Beck J, et al. C9orf72 expansions are the most common genetic cause of Huntington disease phenocopies. Neurology 2014;82:292-9.
29. Alva-Diaz C, Alarcon-Ruiz CA, Pacheco-Barrios K, et al. C9orf72 hexanucleotide repeat in Huntington-like patients: systematic review and meta-analysis. Front Genet 2020;11:551780.
30. Sattler R, Traynor BJ, Robertson J, et al. Attendees of the inaugural C9ORF72 FTD/ALS Summit. Roadmap for C9ORF72 in frontotemporal dementia and amyotrophic lateral sclerosis: report on the C9ORF72 FTD/ALS summit. Neurol Ther 2023;12:1821-43.
31. Wardle M, Morris HR, Robertson NP. Clinical and genetic characteristics of non-Asian dentatorubral-pallidoluysian atrophy: a systematic review. Mov Disord 2009;24:1636-40.
32. Madeo M, Stewart M, Sun Y, et al. Loss-of-function mutations in FRRS1L lead to an epileptic-dyskinetic encephalopathy. Am J Hum Genet 2016;98:1249-55.
33. van der Knaap MS, Barth PG, Gabreëls FJ, et al. A new leukoencephalopathy with vanishing white matter. Neurology 1997;48:845-55.
34. Snell RG, MacMillan JC, Cheadle JP, et al. Relationship between trinucleotide repeat expansion and phenotypic variation in Huntington’s disease. Nat Genet 1993;4:393-7.
35. Rubinsztein DC, Leggo J, Chiano M, et al. Genotypes at the GluR6 kainate receptor locus are associated with variation in the age of onset of Huntington disease. Proc Natl Acad Sci U S A 1997;94:3872-6.
36. Lee JH, Lee JM, Ramos EM, et al. Registry Study of the European Huntington’s Disease Network; Huntington Study Group COHORT project. TAA repeat variation in the GRIK2 gene does not influence age at onset in Huntington’s disease. Biochem Biophys Res Commun 2012;424:404-8.
37. Genetic Modifiers of Huntington’s Disease (GeM-HD) Consortium. Identification of genetic factors that modify clinical onset of Huntington’s disease. Cell 2015;162:516-26.
38. Lee JM, Chao MJ, Harold D, et al. A modifier of Huntington’s disease onset at the MLH1 locus. Hum Mol Genet 2017;26:3859-67.
39. Orth M, Handley OJ, Schwenke C, et al. European Huntington’s Disease Network. Observing Huntington’s disease: the European Huntington’s Disease Network’s REGISTRY. J Neurol Neurosurg Psychiatry 2011;82:1409-12.
40. Moss DJH, Pardiñas AF, Langbehn D, et al. TRACK-HD investigators; REGISTRY investigators. Identification of genetic variants associated with Huntington’s disease progression: a genome-wide association study. Lancet Neurol 2017;16:701-11.
41. Flower M, Lomeikaite V, Ciosi M, et al. TRACK-HD Investigators; OPTIMISTIC Consortium. MSH3 modifies somatic instability and disease severity in Huntington’s and myotonic dystrophy type 1. Brain 2019;142:1876-86.
42. Kennedy L, Evans E, Chen CM, et al. Dramatic tissue-specific mutation length increases are an early molecular event in Huntington disease pathogenesis. Hum Mol Genet 2003;12:3359-67.
43. Kacher R, Lejeune FX, Noël S, et al. Propensity for somatic expansion increases over the course of life in Huntington disease. Elife 2021;10:e64674.
44. Kennedy L, Shelbourne PF. Dramatic mutation instability in HD mouse striatum: does polyglutamine load contribute to cell-specific vulnerability in Huntington’s disease? Hum Mol Genet 2000;9:2539-44.
45. Hong EP, MacDonald ME, Wheeler VC, et al. Huntington’s disease pathogenesis: two sequential components. J Huntingtons Dis 2021;10:35-51.
46. Hong EP, Chao MJ, Massey T, et al. Association analysis of chromosome X to identify genetic modifiers of Huntington’s disease. J Huntingtons Dis 2021;10:367-75.
47. Ellis N, Tee A, McAllister B, et al. Genetic risk underlying psychiatric and cognitive symptoms in Huntington’s disease. Biol Psychiatry 2020;87:857-65.
48. Barbier M, Davoine CS, Petit E, et al. Intermediate repeat expansions of TBP and STUB1: genetic modifier or pure digenic inheritance in spinocerebellar ataxias? Genet Med 2023;25:100327.
49. Li A, Yao S, Liu J, Qi X, Duan F, Sun C. Dilemma in differentiation of spinocerebellar ataxia type 17 from Huntington’s disease: comorbidity or independent disease? Int J Neurosci 2023:1-9.
50. Laffita-Mesa JM, Nennesmo I, Paucar M, Svenningsson P. A novel duplication in ATXN2 as modifier for spinocerebellar ataxia 3 (SCA3) and C9ORF72-ALS. Mov Disord 2021;36:508-14.
51. Warby SC, Montpetit A, Hayden AR, et al. CAG expansion in the Huntington disease gene is associated with a specific and targetable predisposing haplogroup. Am J Hum Genet 2009;84:351-66.
52. Warby SC, Visscher H, Collins JA, et al. HTT haplotypes contribute to differences in Huntington disease prevalence between Europe and East Asia. Eur J Hum Genet 2011;19:561-6.
53. Apolinário TA, Rodrigues DC, Lemos MB, Antão Paiva CL, Agostinho LA. Distribution of the HTT gene A1 and A2 haplotypes worldwide: a systematic review. Clin Med Res 2020;18:145-52.
54. Li XY, Li HL, Dong Y, et al. Haplotype analysis encompassing HTT gene in Chinese patients with Huntington’s disease. Eur J Neurol 2020;27:273-9.
55. Squitieri F, Mazza T, Maffi S, et al. Tracing the mutated HTT and haplotype of the African ancestor who spread Huntington disease into the Middle East. Genet Med 2020;22:1903-8.
56. Ruiz de Sabando A, Urrutia Lafuente E, Galbete A, et al; Spanish HD Collaborative group. Spanish HTT gene study reveals haplotype and allelic diversity with possible implications for germline expansion dynamics in Huntington disease. Hum Mol Genet 2023;32:897-906.
57. Dawson J, Baine-Savanhu FK, Ciosi M, Maxwell A, Monckton DG, Krause A. A probable cis-acting genetic modifier of Huntington disease frequent in individuals with African ancestry. HGG Adv 2022;3:100130.
58. Kolli N, Lu M, Maiti P, Rossignol J, Dunbar GL. CRISPR-Cas9 mediated gene-silencing of the mutant Huntingtin gene in an in vitro model of Huntington’s disease. Int J Mol Sci 2017;18:754.
59. Helm J, Schöls L, Hauser S. Towards personalized allele-specific antisense oligonucleotide therapies for toxic gain-of-function neurodegenerative diseases. Pharmaceutics 2022;14:1708.
60. Fang L, Monteys AM, Dürr A, et al. Haplotyping SNPs for allele-specific gene editing of the expanded huntingtin allele using long-read sequencing. HGG Adv 2023;4:100146.
61. Berger SL, Kouzarides T, Shiekhattar R, Shilatifard A. An operational definition of epigenetics. Genes Dev 2009;23:781-3.
62. Ratovitski T, Kamath SV, O’Meally RN, et al. Arginine methylation of RNA-binding proteins is impaired in Huntington’s disease. Hum Mol Genet 2023;32:3006-25.
63. Ghafouri-Fard S, Khoshbakht T, Hussen BM, Taheri M, Ebrahimzadeh K, Noroozi R. The emerging role of long non-coding RNAs, microRNAs, and an accelerated epigenetic age in Huntington’s disease. Front Aging Neurosci 2022;14:987174.
64. Horvath S, Langfelder P, Kwak S, et al. Huntington’s disease accelerates epigenetic aging of human brain and disrupts DNA methylation levels. Aging 2016;8:1485-512.
65. Barbé L, Finkbeiner S. Genetic and epigenetic interplay define disease onset and severity in repeat diseases. Front Aging Neurosci 2022;14:750629.
66. Gardiner SL, Boogaard MW, Trompet S, et al. Prevalence of carriers of intermediate and pathological polyglutamine disease-associated alleles among large population-based cohorts. JAMA Neurol 2019;76:650-6.
67. Faquih TO, Aziz NA, Gardiner SL, et al. Normal range CAG repeat size variations in the HTT gene are associated with an adverse lipoprotein profile partially mediated by body mass index. Hum Mol Genet 2023;32:1741-52.
68. Gardiner SL, de Mutsert R, Trompet S, et al. Repeat length variations in polyglutamine disease-associated genes affect body mass index. Int J Obes 2019;43:440-9.
69. Gardiner SL, Trompet S, Sabayan B, et al. Repeat variations in polyglutamine disease-associated genes and cognitive function in old age. Neurobiol Aging 2019;84:236.e17-28.
70. Gardiner SL, van Belzen MJ, Boogaard MW, et al. Huntingtin gene repeat size variations affect risk of lifetime depression. Transl Psychiatry 2017;7:1277.
71. McColgan P, Thobhani A, Boak L, et al. GENERATION HD1 Investigators. Tominersen in adults with manifest Huntington’s disease. N Engl J Med 2023;389:2203-5.
72. Goldman JS, Uhlmann WR, Naini AB, Klitzman RL, Marder KS. Genetic testing of HTT modifiers for Huntington’s disease: considerations for clinical guidelines. Mov Disord 2023;38:2151-4.
73. Kim R, Seong MW, Oh B, et al. Analysis of HTT CAG repeat expansion among healthy individuals and patients with chorea in Korea. Parkinsonism Relat Disord 2024;118:105930.
74. Jiang H, Sun YM, Hao Y, et al. Chinese HD Network. Huntingtin gene CAG repeat numbers in Chinese patients with Huntington’s disease and controls. Eur J Neurol 2014;21:637-42.
75. MacDonald ME, Vonsattel JP, Shrinidhi J, et al. Evidence for the GluR6 gene associated with younger onset age of Huntington’s disease. Neurology 1999;53:1330-2.
76. Holbert S, Denghien I, Kiechle T, et al. The Gln-Ala repeat transcriptional activator CA150 interacts with huntingtin: neuropathologic and genetic evidence for a role in Huntington’s disease pathogenesis. Proc Natl Acad Sci U S A 2001;98:1811-6.
77. Nazé P, Vuillaume I, Destée A, Pasquier F, Sablonnière B. Mutation analysis and association studies of the ubiquitin carboxy-terminal hydrolase L1 gene in Huntington’s disease. Neurosci Lett 2002;328:1-4.
78. Arning L, Kraus PH, Valentin S, Saft C, Andrich J, Epplen JT. NR2A and NR2B receptor gene variations modify age at onset in Huntington disease. Neurogenetics 2005;6:25-8.
79. Zeng W, Gillis T, Hakky M, et al. Genetic analysis of the GRIK2 modifier effect in Huntington’s disease. BMC Neurosci 2006;7:62.
80. Metzger S, Bauer P, Tomiuk J, et al. The S18Y polymorphism in the UCHL1 gene is a genetic modifier in Huntington’s disease. Neurogenetics 2006;7:27-30.
81. Panegyres PK, Beilby J, Bulsara M, Toufexis K, Wong C. A study of potential interactive genetic factors in Huntington’s disease. Eur Neurol 2006;55:189-92.
82. Kishikawa S, Li JL, Gillis T, et al. Brain-derived neurotrophic factor does not influence age at neurologic onset of Huntington’s disease. Neurobiol Dis 2006;24:280-5.
83. Arning L, Saft C, Wieczorek S, Andrich J, Kraus PH, Epplen JT. NR2A and NR2B receptor gene variations modify age at onset in Huntington disease in a sex-specific manner. Hum Genet 2007;122:175-82.
84. Andresen JM, Gayán J, Cherny SS, et al. US-Venezuela Collaborative Research Group. Replication of twelve association studies for Huntington’s disease residual age of onset in large Venezuelan kindreds. J Med Genet 2007;44:44-50.
85. Metzger S, Rong J, Nguyen HP, et al. Huntingtin-associated protein-1 is a modifier of the age-at-onset of Huntington’s disease. Hum Mol Genet 2008;17:1137-46.
86. Arning L, Monté D, Hansen W, et al. ASK1 and MAP2K6 as modifiers of age at onset in Huntington’s disease. J Mol Med 2008;86:485-90.
87. Weydt P, Soyal SM, Gellera C, et al. The gene coding for PGC-1alpha modifies age at onset in Huntington’s disease. Mol Neurodegener 2009;4:3.
88. Dhaenens CM, Burnouf S, Simonin C, et al. Huntington French Speaking Network. A genetic variation in the ADORA2A gene modifies age at onset in Huntington’s disease. Neurobiol Dis 2009;35:474-6.
89. Metzger S, Saukko M, Van Che H, et al. Age at onset in Huntington’s disease is modified by the autophagy pathway: implication of the V471A polymorphism in Atg7. Hum Genet 2010;128:453-9.
90. Tsai YC, Metzger S, Riess O, Soehn AS, Nguyen HP. Genetic analysis of polymorphisms in the kalirin gene for association with age-at-onset in European Huntington disease patients. BMC Med Genet 2012;13:48.
91. Lobanov SV, McAllister B, McDade-Kumar M, et al. REGISTRY Investigators of the European Huntington’s disease network; PREDICT-HD Investigators of the Huntington Study Group. Huntington’s disease age at motor onset is modified by the tandem hexamer repeat in TCERG1. NPJ Genom Med 2022;7:53.
92. Karadima G, Dimovasili C, Koutsis G, Vassilopoulos D, Panas M. Age at onset in Huntington’s disease: replication study on the association of HAP1. Parkinsonism Relat Disord 2012;18:1027-8.
93. Kloster E, Saft C, Epplen JT, Arning L. CNR1 variation is associated with the age at onset in Huntington disease. Eur J Med Genet 2013;56:416-9.
94. Valcárcel-Ocete L, Alkorta-Aranburu G, Iriondo M, et al. REGISTRY investigators of the European Huntington’s Disease Network. Exploring genetic factors involved in Huntington disease age of onset: E2F2 as a new potential modifier gene. PLoS One 2015;10:e0131573.
95. Li JL, Hayden MR, Almqvist EW, et al. A genome scan for modifiers of age at onset in Huntington disease: the HD MAPS study. Am J Hum Genet 2003;73:682-7.
96. Li JL, Hayden MR, Warby SC, et al. Genome-wide significance for a modifier of age at neurological onset in Huntington’s disease at 6q23-24: the HD MAPS study. BMC Med Genet 2006;7:71.
Cite This Article
How to Cite
Download Citation
Export Citation File:
Type of Import
Tips on Downloading Citation
Citation Manager File Format
Type of Import
Direct Import: When the Direct Import option is selected (the default state), a dialogue box will give you the option to Save or Open the downloaded citation data. Choosing Open will either launch your citation manager or give you a choice of applications with which to use the metadata. The Save option saves the file locally for later use.
Indirect Import: When the Indirect Import option is selected, the metadata is displayed and may be copied and pasted as needed.
About This Article
Copyright
Data & Comments
Data
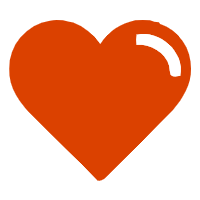
Comments
Comments must be written in English. Spam, offensive content, impersonation, and private information will not be permitted. If any comment is reported and identified as inappropriate content by OAE staff, the comment will be removed without notice. If you have any queries or need any help, please contact us at [email protected].