Neuropathological insights from SHANK3 mutant animal models
Abstract
SHANK3 is a protein primarily found in the postsynaptic density (PSD) of excitatory synapses in the brain. Heterozygous mutations in the shank3 gene have been linked to autism spectrum disorder (ASD) and intellectual disability. There are various animal models carrying mutant SHANK3 that have provided valuable insights into the pathogenesis of ASD. In this review, we will discuss these animal models, with a specific focus on the neuropathology observed in shank3 mouse and monkey models. These models are particularly important as they share closer similarities to humans and are capable of more accurately recapitulating the neuropathological features observed in individuals with ASD. Mice with mutations in the shank3 gene exhibit deficits in social behavior, communication, and repetitive behaviors, which are core features of ASD and support the link between SHANK3 and ASD. However, studies of monkey models with SHANK3 targeting by CRISPR/Cas9 have demonstrated that, unlike mice with completely knocked-out shank3 genes, the monkey model with complete deletion of SHANK3 displays a reduction in the number of neuronal cells. This review discusses the species-specific neuropathology in SHANK3/shank3 knockout mice and monkeys. The differences in neuropathology in SHANK3/shank3 mutant mouse and monkey models suggest that non-human primate models are highly valuable for investigating the mechanism of neurodegeneration that may selectively occur in primate brains.
Keywords
INTRODUCTION
The SH3 and multiple ankyrin repeat domains 3 (SHANK3) is a scaffold protein primarily expressed in the postsynaptic density of excitatory synapses in the brain. The shank3 gene is located on mouse chromosome 15E3, rat chromosome 7q34, and human chromosome 22q13.3[1]. It consists of 22 exons that encode an
The expression of different isoforms in SHANK3 appears to be driven by various promoters in the SHANK3 gene, which contains at least six intragenic promoters in humans and rodents and produces various types of SHANK3 transcripts and several promoter-specific isoforms (SHANK3a-f)[9-12]. These different isoforms have distinct structural domains and perform different biological functions. In the brain, these isoforms show significant temporal and regional differences. SHANK3a is expressed in the cortex, striatum, and hippocampus during the early developmental stages of synaptogenesis, whereas the SHANK3c/d isoform is predominantly presented in the cerebellum and SHANK3e is weakly expressed in all brain regions[13-15]. Interestingly, human SHANK3 mRNA is also highly expressed in the heart, with moderate expression levels in the brain and spleen[9,16,17]. Moreover, the expression of SHANK3 is characterized by selective isoform compensation[18]. Therefore, it remains to be elucidated how the isoform-specific expression of different SHANK3 isoforms is regulated.
In the mouse brain, SHANK3 protein is predominantly found in the postsynaptic density of excitatory synapses, where it has been widely reported to function in the formation of dendritic spines, synaptic transmission and plasticity, and cytoskeleton regulation[3,5-7,10,16-19]. In addition to the major role of shank3 in regulating synaptic formation and function, the expression of SHANK3 in cells of the oligodendrocyte lineage plays a crucial role in the regulation of myelinating cell maturation[20]. Additionally, SHANK3 is expressed in multiple tissues, including skeletal muscle and intestinal epithelial cells, where it is involved in the regulation of barrier function[12,21-23]. Although SHANK3 is also found in the nucleus and cytoplasm[14,24], the precise biological roles of SHANK3 in these subcellular compartments remain inadequately characterized. Future investigations of the expression patterns of various SHANK3 isoforms across diverse tissues and subcellular organelles, as well as their corresponding functional implications, will be pivotal in elucidating the function of SHANK3 and its involvement in ASD.
SHANK3 MUTATIONS AND ASD
ASD is a neurodevelopmental condition that is clinically heterogeneous and highly heritable. Its main clinical features include impaired social interaction and repetitive behaviors. Heterozygous mutations in the SHANK3 gene have been found to be closely associated with ASD, with truncating mutations in the SHANK gene family accounting for approximately 1% of autism cases[25]. Phelan-McDermid Syndrome (PMDS) is the first reported neurodevelopmental disorder associated with heterozygous SHANK3 mutations. It is characterized by global developmental delay, absent or delayed speech, dysmorphic features, hypotonia, and ASD. PMDS is linked to SHANK3 mutations (22q13.3 deletions) that result in SHANK3 haploinsufficiency. While SHANK3 haploinsufficiency resulting from point mutations is sufficient to produce the extensive phenotypic characteristics linked to PMDS, genotype-phenotype correlation analysis has demonstrated that the magnitude of the deletion is positively associated with the severity of clinical manifestations[17,26-28]. Additionally, individuals with smaller terminal deletions may exhibit more favorable developmental trajectories compared to those with larger deletions. The size of SHANK3 deletion fragments also correlates with disease severity.
Similarly, a cohort study conducted on a Brazilian population revealed a positive correlation between the size of SHANK3 deletion and the severity of renal abnormalities, lymphedema, and language impairment in PMDS patients[29]. Another cohort study from a Chinese population reported additional significant phenotypes, including heightened pain tolerance, impulsivity, repetitive behaviors, regression, and incessant crying[30]. Furthermore, imaging techniques have been extensively utilized to identify specific characteristics and structural brain abnormalities in ASD patients[31]. From a pathological perspective, young children diagnosed with ASD exhibit a notable decrease in neuronal and cytoplasmic volumes in the majority of regions examined compared to controls of the same age[32]. Conversely, a separate study revealed a 67% increase in the number of neurons in the entire prefrontal cortex (PFC) among male individuals with autism[33]. However, this datum is estimated to be the volume of reference for the regions of interest in order to derive total cell counts. It is important to exercise caution in interpreting these findings. The pathological and imaging reports of individuals with ASD commonly exhibit limitations such as small sample sizes and variations in the statistical methodologies employed. Therefore, it is crucial to establish a suitable animal model with SHANK3 mutations in order to effectively simulate ASD and investigate its pathogenesis.
SMALL ANIMAL MODELS WITH SHANK3 MUTATIONS
Various small animal models carrying shank/shank3 mutations, such as drosophila, zebrafish, mice, and rats, have been generated to extensively investigate the molecular phenotypes related to shank3 mutations. Due to their high efficiency in genetic manipulations and rapid generation, drosophila and zebrafish have proven to be valuable tools for investigating the function of shank3. In one study, shank null drosophila models were found to be fully viable and fertile, showing no apparent morphological or developmental defects. However, these models did exhibit an abnormal structure of the central mushroom body calyx and reduced olfactory acuity[34]. Another shank null drosophila model demonstrated defects in synaptic bouton number and maturation[35].
Similar synaptic defects related to shank3 deficiency were observed in shank3 null zebrafish models. The adult brains of shank3b deficient zebrafish showed significantly reduced levels of both postsynaptic homer1 and presynaptic synaptophysin. These reductions were accompanied by a decrease in locomotor activity, impaired social interaction, and significant repetitive swimming behaviors[36]. Another shank3b mutant zebrafish model also exhibited reductions in synaptic proteins and locomotor activity[37]. Furthermore, reduced sensory responsiveness in shank3ab-/- zebrafish was associated with decreased activity in sensory processing brain regions[38]. In zebrafish, CRISPR-Cas9-mediated mutations in the shank3a gene led to the downregulated expression of neuroligins, which are crucial for synaptogenesis. This downregulation could contribute to deficits in attention[39].
It is important to acknowledge that zebrafish and drosophila are evolutionarily distant from humans; the expression of shank3 in these small animals is distinct from that in mammals. For example, the zebrafish gene corresponding to human SHANK3 exists as two duplicates. A number of mouse models carrying shank3 mutations have been established and extensively investigated. The mouse model with exon 21 deletion displays a reduced N-methyl-D-aspartate/alpha-amino-3-hydroxy-5-methyl-4-isoxazolepropionic acid (NMDA/AMPA) ratio in the hippocampal synapses, resulting in a significant rise in mGluR5 localization to synaptosomes, motor-coordination deficits, abnormal behaviors, and social interaction[40]. However, the phenotypes of shank3 mutant mice appear to be complex. Mice with shank3 mutations in exon 9 did not display autistic behaviors, although they exhibited a reduction in the frequency of miniature inhibitory postsynaptic currents (mIPSCs) in pyramidal neurons of the medial prefrontal cortex (mPFC), which contrasts with the observed increase in mIPSC frequency in the hippocampus[41]. The InsG3680 mutation in mice is associated with early-onset striatal synaptic transmission defects and impaired social interaction, as well as distinct alterations in synaptic transmission that were also seen in the prefrontal cortex of R1117X mutant mice[42]. Additionally, there is a notable alteration in the composition of PSD in adult R1117X and InsG3680 mutant mice. InsG3680 mutant mice exhibit a significant deficit in social interaction at an early disease stage compared with R1117X mutant mice[42]. The shank3G mouse model demonstrates deficiencies in motor learning and coordination, while exhibiting typical grooming behavior and an aversion to inanimate objects. However, social interaction deficits and anxiety-like behaviors are not observed in this model, although synaptic transmission and plasticity are impaired in these mouse models[43]. Mice with Δe4-22-/- (deletions of exons 4-22) exhibited a deficiency of shank3 that can hinder the metabotropic glutamate receptor 5 (mGluR5) - Homer scaffolding, leading to abnormalities in the cortico-striatal circuitry that are responsible for learning deficits and the manifestation of core behavioral characteristics associated with ASD[44]. The deficiency of shank3 exon 9 in rats results in impaired long-term social memory and attention deficits. Consistently, synaptic plasticity deficits are observed in these rats, from the hippocampus to the prefrontal cortex[45]. In line with the critical role of shank3 in synaptic function, shank3-deficient rats (exons 11-21) exhibited a decrease in spine density and alterations in synaptic proteins[46]. However, these mutant rats display normal social interaction behavior, despite impaired social memory, as well as learning and memory deficits in this rat model[46].
Rodent models with shank3 mutations provide a valuable tool for understanding the function of shank3 and its role in ASD. For instance, these models have demonstrated that mutations or deletions of the shank3 gene affect synaptic signal transmission and synaptic protein expression in various brain regions, such as the striatum, hippocampus, and prefrontal cortex. However, one notable finding is that unlike the severe clinical symptoms observed in humans with SHANK3 haploinsufficiency, shank3+/- mice show no or only very mild phenotypes. The abnormal behavioral phenotypes in mice are almost exclusively found in homozygous mutants[44,47,48]. Additionally, the inconsistent behavioral phenotypes of rodent models with shank3 mutations suggest that mutations in different exons of the shank3 gene and the nature of mutations may account for variations in behavioral phenotypes [Table 1]. Despite the closer phylogenetic proximity of rodents, such as mice and rats, to humans compared to fruit flies and zebrafish, significant disparities persist in terms of rodent brain development, neural circuitry, and anatomy compared to the human brain. As a result, several phenotypes associated with ASD display inconsistencies or contradictions across different mouse or rat models. Additionally, it is important to recognize the potential impact of diverse genetic backgrounds within the same species on the ASD-like phenotype triggered by shank3 mutations. Therefore, there is a pressing need for more extensive research into the intricate pathogenic mechanisms that underlie the neurodevelopmental impairment associated with ASD, which may be unraveled by using non-human primate models that are closer to humans than small animals.
SHANK3 mutant animal models
Species/Background | Modifications approach | Targeted exons and isoforms | Molecular phenotype | Behavioral phenotype | Ref. |
Drosophila/mef2-Gal4 | KO | - Minos transposable element (shankD101 homozygotes) - Loss of 97% coding region of the shank gene | - Glutamate receptors and active zones are not affected - A defect in postsynaptic development - Downregulation of Wnt signaling pathway - Defective NMJ bouton formation and maturation | - No reported | [35] |
Drosophila/W1118 | KO | - Exons4-12 (shank8k) - Exon10 (shank749) - Exon5 (shank138) - Loss of all known SHANK protein isoforms | - Developmental defects of synapse in the calyx - Ultrastructural defects of synaptic boutons in the adult calyx - Normal NMJ development | - Reduced motor ability and defective olfactory acuity | [34] |
Zebrafish/Tu | KO | - Exon2 (ANK)/homozygous - Loss of shank3b - Exon9 (SH3, PDZ, SAM)/homozygous - Loss of shank3a | - Reduced protein expression of NeuN, homer1, and synaptophysin | - Reduced alertness or reduced danger awareness - Impaired social interactions and repetitive and stereotyped behaviors | [37] |
Zebrafish/The Gulbenkian Institute of Science | KO | - Exon2 (ANK, SH3, PDZ, SAM)/homozygous - Loss of shank3a | - Downregulation of neuroligin gene expression - Upregulation of BDNF and NeuroD gene expression | - Social contagion effects and attention-recognition deficits | [39] |
Zebrafish/The University of Miami zebrafish core facility | KO | - shankabΔN-/- (ANK)/homozygous - Loss of shank3a/b - shank3abΔC-/- (near the PDZ)/homozygous - Loss of shank3a/b | - The absence of SHANK3 puncta is observed in the cerebellum and along the ventral neural tracts of the brainstem, while PSD-95 synaptic puncta remain unaffected | - Downstream brain regions fail to integrate and respond to dark transitions in larvae, both shank3abΔN-/- and shank3abΔC-/- | [38] |
Zebrafish/Tu | KO | - Exon2 (ANK, SH3, PDZ)/homozygous - Loss of shank3b | - Reduced homer1 and synaptophysin protein levels in the adult zebrafish brain | - Impaired locomotor activity - Abnormal repetitive movements and impaired social preference behaviors in the adult zebrafish | [36] |
Mouse/Bruce4 C57BL/6 | KO | - Exons4-9 (ANK)/heterozygous - Loss of shank3a and shank3b | - Reductions in glutamatergic synaptic transmission and plasticity - A reduced number of GluR1 immunoreactive puncta in the striatum radiatum | - Social interaction and social communication deficits | [49] |
Mouse/C57BL/6J | KO | - Exons4-9 (ANK)/homozygous - Loss of shank3a and shank3b | - Altered dendritic spine morphology - Impaired synaptic plasticity - Impaired activity-dependent GluA1 redistribution - Intact basal transmission function | - Abnormal social behavior - Aberrant motor behaviors and ultrasonic vocalizations - Repetitive behaviors - Deficient learning and memory | [10] |
Mouse/C57 (Jackson) | KO | - Exons4-7 (ANK)/homozygous - Loss of shank3a | - Defects at striatal synapses and cortico-striatal circuits | - No lesions or anxiety-like behavior | [50] |
Mouse/C57 (Jackson) | KO | - Exons13-16 (PDZ)/homozygous - Loss of shank3a and shank3b | - Reduced spine density, PSD length and thickness | - Anxiety-like behavior and excessive - Self-injurious grooming - Dysfunctional social interaction behavior | [50] |
Mouse/C57BL/6 | KO | - Exon 21 (PRO)/homozygous - Loss SHANK3 protein (> 150 Kd) | - Neuronal morphology appeared unaltered - No differences in spine density and shape - Impaired long-term potentiation | - Impaired spatial learning, poor motor coordination, avoidance towards inanimate objects, and excessive self-grooming in advanced adulthood - No alterations in ultrasonic vocalizations | [40] |
Mouse/C57BL/6N | KO | - Exon 9 (ANK)/heterozygous - Loss of shank3a | - Reduced excitatory transmission and increased mIPSC frequency in hippocampus - Decreased mIPSC frequency but normal mEPSCs in mPFC | - Not autistic-like behavior -Increased rearing in a novel environment - Mildly impaired spatial memory | [41] |
Mouse/C57BL/6J | KO | -Exon 21 (PRO)/homozygous -Loss of SHANK3 isoforms (> 150 Kd) | -No change in glutamate receptor or Homer1b/c expression in whole shank3G/G mice hippocampal lysates -Decreased phosphorylation of the GluN2B Tyr1472 site | -Impaired motor learning and coordination -An avoidance phenotype towards inanimate objects -Aberrant locomotor activity in response to novelty -Not express an anxiety-like phenotype -Minimal spatial learning differences -Not display social interaction deficits -Normal grooming | [43] |
Mouse/C57BL6/S129sv | KO | - Exon 21 (PRO)/homozygous - Expressed SHANK3 (122 kDa) - R1117X mutation | - No change of mEPSC frequency and AMPA to NMDA current ratio - Reduced Homer protein, PSD93, SynGAP - Reduced NMDA receptor subunits - Not see a trend of upregulation of any of the synaptic proteins tested - Reduction of mEPSC amplitude - Reduction of the spine density layer 2/3 pyramidal neurons in the frontal association area | - Social interaction deficit, but it did not reach statistical significance | [42] |
Mouse/C57BL6/S129s | KO | - Exon 21 (PRO)/homozygous - An almost complete loss of SHANK3 protein - InsG3680 mutation | - No change of mEPSC and AMPA to NMDA current ratio frequency - Increase of mEPSC amplitude - Reduced Homer protein, GluR1, SynGAP, SHANK2, and NMDA receptor subunits - No significant differences in either frequency or amplitude of mEPSC - Reduction of the spine density of layer 2/3 pyramidal neurons in the frontal association area | - An early-onset social interaction deficit | [42] |
Mouse/C57BL/6J | KO | - Exons4-22 (ANK, PDZ, PRO)/homozygous - Loss of all known SHANK3 protein isoforms | - NAC firing deficit - Abnormalities in brain structure - Dysfunctional striatal synapses | - Core behavioral features of ASDs - Intact fear learning - Mildly perturbed hippocampal spatial memory - Severely impaired striatal learning - Abnormal ultrasonic vocalizations | [44] |
Rat/Sprague Dawley | KO | - Exon 6 (ANK)/heterozygous - Loss of shank3a | - Induced LTP | - Impairs long-term social memory - Normal social interaction -Attention deficits | [45] |
Rat/Charles River Laboratories | KO | - Exons11-21 (PDZ, SH3, PRO)/homozygous - Loss of all known SHANK3 protein isoforms | - Reduced spine density, PSD-95 - Reduced Homer and NR1 in homogenates from the striatum - Increased PSD-95 in the hippocampal PSD fraction of heterozygous rats - NR1 increased in the hippocampal homogenate fraction of heterozygous rats | - Normal social interaction behavior - Impaired social memory and learning memory - Increased anxiety behavior and pain threshold - Self-grooming behavior and skin lesions | [46] |
Monkey/Cynomolgus monkeys | KO | - Exons 6-12 (ANK, PDZ)/mosaic -All known SHANK3 protein isoforms reduced | - Loss of neuronal cells - Increase of GFAP+ astrocytes - Altered expressions of postsynaptic receptors and scaffold proteins | - Impaired social interaction and apparent stereotypical locomotion - Delayed vocalization - No obvious structural abnormality - Lower glucose metabolism - Fluoxetine can alleviate the behavioral deficits | [51] |
Monkey/Cynomolgus monkeys | KO | - Exon 21 (PRO)/F0: mosaic; F1: heterozygous - All known SHANK3 protein isoforms reduced | Not reported | - Reduced overall sleep efficiency and muscle strength - Increased repetitive behaviors - Reduced exploration and social interaction - Fewer vocalizations - Decreased grey matter - Abnormal functional connectivity | [52] |
Monkey models with SHANK3 mutations
Macaca monkeys possess high-order cognitive and social functions thanks to their well-developed neocortex. They are closer to humans in terms of their specialized brain function and structures than rodents[47,53,54]. The value of using primate models to study ASD has been supported by the generation of monkeys with altered expressions of MECP2 using lentivirus[55] and TALEN[56]-based methods. However, a SHANK3-edited monkey model was not reported until 2017. To date, two monkey models of SHANK3 mutants have been established using CRISPR/Cas9-mediated gene-editing technology in cynomolgus monkeys[51,52]. Despite the limited number of SHANK3 mutant monkeys generated, they provide important insights into the function of SHANK3 and its role in ASD, which deserves a thorough discussion below.
Targeted exons 6 and 12 of the monkey SHANK3 gene
In 2017, Zhao et al. generated the first SHANK3-edited monkey[51]. Since a large deletion of shank3 in the mouse gene did not yield obvious phenotypes and pathological changes, Zhao et al. aimed to establish a monkey model with a large SHANK3 gene deletion[51]. They targeted two sites (exons 6 and 12) of the monkey SHANK3 gene, which could completely disrupt all three longest SHANK3 isoforms in the fertilized eggs of cynomolgus monkeys. Although three SHANK3 mutant monkeys were obtained, two (M1, M2) died before or right after birth, and one (M3) survived. A higher-than-expected embryonic and perinatal lethality associated with SHANK3 targeting was observed, suggesting that SHANK3 is important for early development in primates. Analysis of SHANK3-targeted monkeys revealed that the M1 monkey brains showed almost complete deletion of SHANK3 protein, accompanied by a dramatic reduction in many postsynaptic receptors and scaffold proteins, such as GluN2B/mGluR5/PSD95. Meanwhile, the SHANK3-deficient monkey showed a significant loss of NeuN+ neurons co-exhibited by an increase of GFAP+ astrocytes [Figure 1]. These findings provide strong evidence for neuronal loss resulting from SHANK3 depletion, which has not been reported in any line of shank3-knockout mice. This demonstrates that SHANK3 plays a unique and critical role in early brain development in primate brains.
Figure 1. CRISPR/Cas9-mediated SHANK3 deletion results in neuronal loss in the monkey brain. (A) Western blot analysis of SHANK3 protein in the PFC and striatum of SHANK3-mutant monkeys. Ctl1 and Ctl2 are age-matched controls at gestational day 135 and full-term gestation, respectively; (B) Altered expressions of postsynaptic receptors and scaffold proteins in SHANK3M1 brain. Normal expressions of GluN1, GluA2, Homer1b/c, and pan-Homer but decreased expressions of GluN2B, mGluR5, and PSD95 were observed in the PFC of SHANK3M1; (C) Western blot analysis of NeuN, GFAP, and DCX in whole-cell lysates from PFC. SHANK3M1 showed decreased levels of NeuN and DCX but increased levels of GFAP in the PFC. Western blot analysis was repeated at least three times independently; (D) NeuN and GFAP staining (brown) in layers I-VI of PFC. NeuN staining showed a strong signal in the nucleus and a weak signal in the cytosol. Nuclei were stained with hematoxylin (blue). There were fewer NeuN+ neurons and more GFAP+ astrocytes in the PFC of SHANK3M1. Scale bar, 100 μm[51]. DCX: Doublecortin; GFAP: glial fibrillary acidic protein; NeuN: neuronal nuclei; PFC: prefrontal cortex; PSD: postsynaptic density; SHANK3: SH3 and multiple ankyrin repeat domains 3.
For the living SHANK3-targeted monkey (M3), a longitudinal and repeated investigation over a 2-year period had been performed[57]. This investigation revealed that the SHANK3-targeted monkey developed the core features of behavioral phenotypes of ASD, including impaired social interaction, apparent stereotypical locomotion, and delayed vocalization[57]. Although the exact meaning of delayed vocalization is not clear, the defect in vocalization may resemble delayed speech in many young patients with SHANK3 mutations. To investigate possible structural and functional changes, the authors conducted positron emission computed tomography (PET) and magnetic resonance imaging (MRI) in mutant and control monkeys. Morphological T1-weighted 3-dimensional MRI demonstrated that there were no obvious structural abnormalities in SHANK3-mutant monkeys. However, the 18F-FDG-PET study showed that glucose metabolism in SHANK3-mutant brain tissues was significantly lower than in control brains. This phenotype is consistent with the lower glucose metabolic activity in the brains of some ASD patients[58,59]. To explore the therapeutic potential, they treated SHANK3-mutant monkeys with fluoxetine, a medication for major depression and obsessive-compulsive disorder. After treatment for 2 weeks, fluoxetine markedly alleviated repetitive behaviors, significantly increased active social interaction duration and frequency, and improved brain glucose metabolism in SHANK3-targeted monkeys[57].
Targeted exons 21 of the monkey SHANK3 gene
Another SHANK3-targeted monkey model was generated to target exon 21 only[52] to create SHANK3 mutations similar to the InsG3680 mutation found in humans[60]. The authors not only conducted comprehensive behavioral experiments to analyze this monkey model but also obtained F1-generation heterozygous SHANK3-mutant monkeys to ensure germline transmission of the SHANK3 mutations[52]. The behavioral assessment scores showed that overall sleep efficiency and muscle strength were reduced in SHANK3-mutant monkeys. However, the stereotyped or repetitive behaviors (such as licking fingers and cage bars) revealed a substantial increase compared to controls. Meanwhile, SHANK3-mutant monkeys displayed reduced levels of exploration, social interaction, and vocalization, which parallels some of the phenotypes found in children with ASD or PMDS[61,62]. Contrary to the previous SHANK3-targeted monkey model[57], structural MRI analysis of the new SHANK3-mutant monkeys revealed that the volume of gray matter had decreased. In addition, reduced global connectivity and greater local connectivity were also observed in the new SHANK3 mutant monkeys.
Although there are small differences in behavioral phenotypes between the two SHANK3-targeted monkey models, most of the behavioral alterations in SHANK3-targeted monkeys were similar to the clinical characteristics of autism patients. However, unlike monkeys with a large SHANK3 gene deletion[51], the monkeys with exon 21 targeting were not reported to have neuronal loss[52]. Thus, it is likely that complete loss of SHANK3 can more severely affect neuronal survival and development, whereas partial loss of SHANK3 or depletion of some isoforms of SHANK3 may lead to haploinsufficiency as seen in heterozygous SHANK3 mutations in humans.
Behavioral phenotypes of SHANK3 mutant monkey models highlight the complex regulation of SHANK3 expression and its multifaceted functions in large animals. Thus, large animals with SHANK3 mutations may provide unique pathogenesis insights that have not been identified in small animals. Indeed, dog models with SHANK3 mutations were also created and provided some interesting findings. The electrocorticograms (ECoG) revealed that SHANK3 mutant dogs responded to sound stimulation more intensely and were more sensitive to frequencies (1-4 kHz) that are sensitive to the human auditory system[63]. Stem cells from human exfoliated deciduous teeth transplantation improved social novelty preference, reduced social stress, and decreased serum IF-γ levels in heterozygous SHANK3 mutant beagle dogs[64]. Considering that non-human primates are closer to humans than other animals, SHANK3-mutant monkeys would be a more faithful animal model for investigating the pathogenesis of human ASD.
SPECIES-SPECIFIC NEURODEGENERATION
In heterozygous shank3+/- knockout mice, there are no or only very mild phenotypes, which is in contrast to the more severe clinical symptoms observed in patients with SHANK3 heterozygous mutations. Abnormal behavioral phenotypes in mice are almost exclusively found in homozygous mutants[44,47,48]. However, homozygous SHANK3 mutations have not been reported in humans, possibly because such mutations are embryonic lethal or can severely affect early brain development. In support of this idea, a large deletion of the monkey SHANK3 gene can cause remarkable neuronal loss[51]. The absence of such neuronal loss in complete shank3 knockout mice clearly indicates that species-dependent differences are important for the development of neurodegeneration seen in humans.
Analogous to other neurodegenerative diseases such as Alzheimer’s, Parkinson’s, and Huntington’s diseases, genetically modified mouse models fail to recapitulate the overt and selective neurodegeneration observed in patient brains[65]. For example, homozygous mutations in PINK1, a kinase that is thought to be involved in mitophagy, can cause neurodegeneration in Parkinson’s disease via the loss of function mechanism. However, the complete loss of Pink1 in knockout mice does not lead to neurodegeneration or obvious phenotypes[66-68]. In contrast, CRISPR/Cas9-mediated deletion of the PINK1 gene in monkeys results in severe neurodegeneration[69]. Furthermore, this primate-specific neurodegeneration caused by PINK1 deficiency is due to reduced protein phosphorylation rather than mitochondrial homeostasis defects[65]. Thus, non-human primate models may enable us to identify the pathogenesis that occurs in the human brain but is hardly replicated in small animals.
The identification of neuronal loss in SHANK3 mutant monkeys also highlights the important function of SHANK3 for neuronal survival during early brain development. It is highly likely that in primate brains, SHANK3 is required for neuronal differentiation and maturation, such that the complete loss of SHANK3 would affect the survival of neurons. However, further studies are needed to pinpoint the mechanisms of neuronal loss in SHANK3-deficient primates. On the other hand, partial deficiency in the expression or function of SHANK3 due to heterozygous mutations or deficiency in some isoforms is more likely to affect synaptic function and neuronal circuitry, leading to autistic phenotypes without neurodegeneration. Given that the expression of SHANK3 is driven by multiple promoters to yield various isoforms, the use of non-human primates would allow for investigating the relationship between neuronal loss and different isoforms. Such studies would provide new insight into the function of SHANK3 and a better understanding of how SHANK3 mutations alter behaviors to cause ASD.
It is important to note that in Zhao et al.’s report, only one SHANK3-targeted monkey exhibited almost complete deletion of the SHANK3 gene and severe neuronal loss[51]. The authors did not find any evidence suggesting that the CRISPR/Cas9 targeting used in their study resulted in off-target events responsible for such severe neurodegeneration. While the phenotypes observed in this unique SHANK3 mutant monkey support the notion that complete loss of SHANK3 can lead to severe neuronal loss, further studies utilizing additional SHANK3 mutant monkeys are necessary to validate this concept. Additionally, the neuronal loss observed in the newborn SHANK3 mutant monkey could be attributed to neurodegeneration, impairment of neurogenesis or neuronal differentiation, which warrants further investigation. A recent study demonstrated that SHANK3+/- brain organoids are significantly smaller and contain fewer neurons compared to wild-type controls[70]. Thus, an alternative model for investigating the crucial role of SHANK3 in neuronal development could involve utilizing human brain organoids with CRISPR targeting SHANK3-/-.
THE LIMITATIONS OF THIS ARTICLE
This article presents a comprehensive analysis of various animal models exhibiting shank3 mutations, including zebrafish, Drosophila, mice, rats, dogs, and monkeys, with a focus on molecular mechanisms, behavior, and neuropathology. However, it lacks in-depth exploration regarding specific biological distinctions, such as variations in the manifestation of stereotyped behaviors. The discussion is primarily exploratory, attributing these variances to the diversity within the autism spectrum, disparities in animal model targeting strategies, species distinctions, and other contributing factors. From a clinical perspective, there is a dearth of publicly available data concerning autistic individuals with SHANK3 mutations, thus limiting this article to discussions centered around existing animal models. The primary emphasis lies on the neuropathology caused by SHANK3 deletion, an occurrence observed exclusively in primates but constrained by sample size. This finding underscores the need for future validation, necessitating further research to unravel the neuropathological mechanism of autism.
DISCUSSION
The utilization of large animal models in research is hindered by high costs, lengthy breeding cycles, and operational complexities, resulting in small sample sizes. Nonetheless, large animals, particularly non-human primates, offer distinct advantages in studying brain structure and nerve cell development, enabling researchers to mimic human neuropathies.
Due to the scarcity of neuropathological studies on autism patients and the heterogeneity of autism itself, animal models of autism face significant challenges. Shank3 is among the numerous genes associated with autism. A number of small animal models have demonstrated that SHANK3 is linked to autism-like behaviors and have elucidated its role in synapse formation. However, synaptic dysfunction alone may not adequately explain the complex pathogenic mechanism of SHANK3 mutations. The observation that the onset heterozygosity observed in patients differs from the partial homozygosity seen in small animal models underscores the significance of utilizing non-human primates to investigate the mechanisms of ASD.
It is important to consider the various promoters and forms of the shank3 gene and protein when selecting antibodies and interpreting results. Further research is necessary to understand how different forms of Shank3 contribute to autism and their roles in nerve cells and organs at different developmental stages, which will require validation through animal models. Despite their limited sample sizes, non-human primate models with the deletion of the Shank3 gene display neuronal loss not observed in small animal models. Non-human primates can spontaneously develop Alzheimer's disease (AD) and Parkinson's disease (PD), displaying unique neuronal loss patterns in their models. However, despite advancements, non-human primate models for ASD remain underutilized. Additional research is imperative to confirm and supplement existing data due to the scarcity of animal studies. Specifically, further investigation is needed to understand the mechanisms underlying neuronal loss in non-human primate models lacking Shank3. Addressing this inquiry will not only illuminate the novel functionalities of SHANK3, but also aid in advancing clinical investigations pertaining to individuals with Shank3 mutations.
DECLARATIONS
Authors’ contributions
Wrote the first draft of the commentary: Zhang JW, He DJ
Edited and contributed to the final draft: Li XJ
Availability of data and materials
Not applicable.
Financial support and sponsorship
This study was supported by the National Natural Science Foundation of China (81830032, 82071421, 82271902) and the Natural Science Foundation of Guangdong Province (2022A1515012651, 2022A1515012301).
Conflicts of interest
All authors declared that there are no conflicts of interest.
Ethical approval and consent to participate
Not applicable.
Consent for publication
Not applicable.
Copyright
© The Author(s) 2023.
REFERENCES
1. Delling JP, Boeckers TM. Comparison of SHANK3 deficiency in animal models: phenotypes, treatment strategies, and translational implications. J Neurodev Disord 2021;13:55.
2. Böckers TM, Mameza MG, Kreutz MR, et al. Synaptic scaffolding proteins in rat brain: ankyrin repeats of the multidomain Shank protein family interact with the cytoskeletal protein alpha-fodrin. J Biol Chem 2001;276:40104-12.
3. Sala C, Piëch V, Wilson NR, Passafaro M, Liu G, Sheng M. Regulation of dendritic spine morphology and synaptic function by Shank and Homer. Neuron 2001;31:115-30.
4. Gundelfinger ED, Boeckers TM, Baron MK, Bowie JU. A role for zinc in postsynaptic density asSAMbly and plasticity? Trends Biochem Sci 2006;31:366-73.
5. Liebau S, Proepper C, Schmidt T, Schoen M, Bockmann J, Boeckers TM. ProSAPiP2, a novel postsynaptic density protein that interacts with ProSAP2/Shank3. Biochem Biophys Res Commun 2009;385:460-5.
6. Haeckel A, Ahuja R, Gundelfinger ED, Qualmann B, Kessels MM. The actin-binding protein Abp1 controls dendritic spine morphology and is important for spine head and synapse formation. J Neurosci 2008;28:10031-44.
7. Arons MH, Lee K, Thynne CJ, et al. Shank3 is part of a zinc-sensitive signaling system that regulates excitatory synaptic strength. J Neurosci 2016;36:9124-34.
8. Sarowar T, Grabrucker AM. Actin-dependent alterations of dendritic spine morphology in shankopathies. Neural Plast 2016;2016:8051861.
9. Lim S, Naisbitt S, Yoon J, et al. Characterization of the Shank family of synaptic proteins. Multiple genes, alternative splicing, and differential expression in brain and development. J Biol Chem 1999;274:29510-8.
10. Wang X, McCoy PA, Rodriguiz RM, et al. Synaptic dysfunction and abnormal behaviors in mice lacking major isoforms of Shank3. Hum Mol Genet 2011;20:3093-108.
11. Zhu L, Wang X, Li XL, et al. Epigenetic dysregulation of SHANK3 in brain tissues from individuals with autism spectrum disorders. Hum Mol Genet 2014;23:1563-78.
12. Monteiro P, Feng G. SHANK proteins: roles at the synapse and in autism spectrum disorder. Nat Rev Neurosci 2017;18:147-57.
13. Waga C, Asano H, Sanagi T, et al. Identification of two novel Shank3 transcripts in the developing mouse neocortex. J Neurochem 2014;128:280-93.
14. Wang X, Xu Q, Bey AL, Lee Y, Jiang Y. Transcriptional and functional complexity of Shank3 provides a molecular framework to understand the phenotypic heterogeneity of SHANK3 causing autism and Shank3 mutant mice. Mol Autism 2014;5:30.
15. Bouquier N, Sakkaki S, Raynaud F, et al. The Shank3Venus/Venus knock in mouse enables isoform-specific functional studies of Shank3a. Front Neurosci 2022;16:1081010.
16. Kim Y, Ko TH, Jin C, et al. The emerging roles of Shank3 in cardiac function and dysfunction. Front Cell Dev Biol 2023;11:1191369.
17. Wilson HL, Wong ACC, Shaw SR, et al. Molecular characterisation of the 22q13 deletion syndrome supports the role of haploinsufficiency of SHANK3/PROSAP2 in the major neurological symptoms. J Med Genet 2003;40:575-84.
18. Jin C, Kang HR, Kang H, et al. Unexpected compensatory increase in Shank3 transcripts in Shank3 knock-out mice having partial deletions of exons. Front Mol Neurosci 2019;12:228.
19. Naisbitt S, Kim E, Tu JC, et al. Shank, a novel family of postsynaptic density proteins that binds to the NMDA receptor/PSD-95/GKAP complex and cortactin. Neuron 1999;23:569-82.
20. Malara M, Lutz AK, Incearap B, et al. SHANK3 deficiency leads to myelin defects in the central and peripheral nervous system. Cell Mol Life Sci 2022;79:371.
21. Lutz AK, Pfaender S, Incearap B, et al. Autism-associated SHANK3 mutations impair maturation of neuromuscular junctions and striated muscles. Sci Transl Med 2020;12:eaaz3267.
22. Sauer AK, Bockmann J, Steinestel K, Boeckers TM, Grabrucker AM. Altered Intestinal morphology and microbiota composition in the autism spectrum disorders associated SHANK3 mouse model. Int J Mol Sci 2019;20:2134.
23. Wei SC, Yang-Yen HF, Tsao PN, et al. SHANK3 regulates intestinal barrier function through modulating ZO-1 expression through the PKCε-dependent pathway. Inflamm Bowel Dis 2017;23:1730-40.
24. Grabrucker S, Proepper C, Mangus K, et al. The PSD protein ProSAP2/Shank3 displays synapto-nuclear shuttling which is deregulated in a schizophrenia-associated mutation. Exp Neurol 2014;253:126-37.
25. Leblond CS, Nava C, Polge A, et al. Meta-analysis of SHANK mutations in autism spectrum disorders: a gradient of severity in cognitive impairments. PLoS Genet 2014;10:e1004580.
26. Misceo D, Rødningen OK, Barøy T, et al. A translocation between Xq21.33 and 22q13.33 causes an intragenic SHANK3 deletion in a woman with Phelan-McDermid syndrome and hypergonadotropic hypogonadism. Am J Med Genet A 2011;155:403-8.
27. Sarasua SM, Dwivedi A, Boccuto L, et al. Association between deletion size and important phenotypes expands the genomic region of interest in Phelan-McDermid syndrome (22q13 deletion syndrome). J Med Genet 2011;48:761-6.
28. Luciani JJ, de Mas P, Depetris D, et al. Telomeric 22q13 deletions resulting from rings, simple deletions, and translocations: cytogenetic, molecular, and clinical analyses of 32 new observations. J Med Genet 2003;40:690-6.
29. Samogy-Costa CI, Varella-Branco E, Monfardini F, et al. A Brazilian cohort of individuals with Phelan-McDermid syndrome: genotype-phenotype correlation and identification of an atypical case. J Neurodev Disord 2019;11:13.
30. Xu N, Lv H, Yang T, et al. A 29 Mainland Chinese cohort of patients with Phelan-McDermid syndrome: genotype-phenotype correlations and the role of SHANK3 haploinsufficiency in the important phenotypes. Orphanet J Rare Dis 2020;15:335.
31. Liu C, Li D, Yang H, et al. Altered striatum centered brain structures in SHANK3 deficient Chinese children with genotype and phenotype profiling. Prog Neurobiol 2021;200:101985.
32. Wegiel J, Flory M, Kuchna I, et al. Neuronal nucleus and cytoplasm volume deficit in children with autism and volume increase in adolescents and adults. Acta Neuropathol Commun 2015;3:2.
33. Wegiel J, Flory M, Kuchna I, et al. Brain-region-specific alterations of the trajectories of neuronal volume growth throughout the lifespan in autism. Acta Neuropathol Commun 2014;2:28.
34. Wu S, Gan G, Zhang Z, et al. A presynaptic function of shank protein in Drosophila. J Neurosci 2017;37:11592-604.
35. Harris KP, Akbergenova Y, Cho RW, Baas-Thomas MS, Littleton JT. Shank modulates postsynaptic wnt signaling to regulate synaptic development. J Neurosci 2016;36:5820-32.
36. Liu CX, Li CY, Hu CC, et al. CRISPR/Cas9-induced shank3b mutant zebrafish display autism-like behaviors. Mol Autism 2018;9:23.
37. Liu C, Wang Y, Deng J, et al. Social deficits and repetitive behaviors are improved by early postnatal low-dose VPA intervention in a novel shank3-deficient zebrafish model. Front Neurosci 2021;15:682054.
38. Kozol RA, James DM, Varela I, Sumathipala SH, Züchner S, Dallman JE. Restoring Shank3 in the rostral brainstem of shank3ab-/- zebrafish autism models rescues sensory deficits. Commun Biol 2021;4:1411.
39. Kareklas K, Teles MC, Dreosti E, Oliveira RF. Autism-associated gene shank3 is necessary for social contagion in zebrafish. Mol Autism 2023;14:23.
40. Kouser M, Speed HE, Dewey CM, et al. Loss of predominant Shank3 isoforms results in hippocampus-dependent impairments in behavior and synaptic transmission. J Neurosci 2013;33:18448-68.
41. Lee J, Chung C, Ha S, et al. Shank3-mutant mice lacking exon 9 show altered excitation/inhibition balance, enhanced rearing, and spatial memory deficit. Front Cell Neurosci 2015;9:94.
42. Zhou Y, Kaiser T, Monteiro P, et al. Mice with Shank3 mutations associated with ASD and schizophrenia display both shared and distinct defects. Neuron 2016;89:147-62.
43. Speed HE, Kouser M, Xuan Z, et al. Autism-associated insertion mutation (InsG) of Shank3 exon 21 causes impaired synaptic transmission and behavioral deficits. J Neurosci 2015;35:9648-65.
44. Wang X, Bey AL, Katz BM, et al. Altered mGluR5-Homer scaffolds and corticostriatal connectivity in a Shank3 complete knockout model of autism. Nat Commun 2016;7:11459.
45. Harony-Nicolas H, Kay M, du Hoffmann J, et al. Oxytocin improves behavioral and electrophysiological deficits in a novel Shank3-deficient rat. Elife 2017;6:e18904.
46. Song TJ, Lan XY, Wei MP, et al. Altered behaviors and impaired synaptic function in a novel rat model with a complete Shank3 deletion. Front Cell Neurosci 2019;13:111.
47. Jennings CG, Landman R, Zhou Y, et al. Opportunities and challenges in modeling human brain disorders in transgenic primates. Nat Neurosci 2016;19:1123-30.
49. Bozdagi O, Sakurai T, Papapetrou D, et al. Haploinsufficiency of the autism-associated Shank3 gene leads to deficits in synaptic function, social interaction, and social communication. Mol Autism 2010;1:15.
50. Peça J, Feliciano C, Ting JT, et al. Shank3 mutant mice display autistic-like behaviours and striatal dysfunction. Nature 2011;472:437-42.
51. Zhao H, Tu Z, Xu H, et al. Altered neurogenesis and disrupted expression of synaptic proteins in prefrontal cortex of SHANK3-deficient non-human primate. Cell Res 2017;27:1293-7.
52. Zhou Y, Sharma J, Ke Q, et al. Atypical behaviour and connectivity in SHANK3-mutant macaques. Nature 2019;570:326-31.
53. Bauman MD, Schumann CM. Advances in nonhuman primate models of autism: integrating neuroscience and behavior. Exp Neurol 2018;299:252-65.
54. Izpisua Belmonte JC, Callaway EM, Caddick SJ, et al. Brains, genes, and primates. Neuron 2015;86:617-31.
55. Liu Z, Li X, Zhang JT, et al. Autism-like behaviours and germline transmission in transgenic monkeys overexpressing MeCP2. Nature 2016;530:98-102.
56. Chen Y, Yu J, Niu Y, et al. Modeling rett syndrome using TALEN-edited MECP2 Mutant cynomolgus monkeys. Cell 2017;169:945-55.e10.
57. Tu Z, Zhao H, Li B, et al. CRISPR/Cas9-mediated disruption of SHANK3 in monkey leads to drug-treatable autism-like symptoms. Hum Mol Genet 2019;28:561-71.
58. Haznedar MM, Buchsbaum MS, Hazlett EA, LiCalzi EM, Cartwright C, Hollander E. Volumetric analysis and three-dimensional glucose metabolic mapping of the striatum and thalamus in patients with autism spectrum disorders. Am J Psychiatry 2006;163:1252-63.
59. Buchsbaum MS, Hollander E, Haznedar MM, et al. Effect of fluoxetine on regional cerebral metabolism in autistic spectrum disorders: a pilot study. Int J Neuropsychopharmacol 2001;4:119-25.
60. Durand CM, Betancur C, Boeckers TM, et al. Mutations in the gene encoding the synaptic scaffolding protein SHANK3 are associated with autism spectrum disorders. Nat Genet 2007;39:25-7.
61. Richards C, Powis L, Moss J, Stinton C, Nelson L, Oliver C. Prospective study of autism phenomenology and the behavioural phenotype of Phelan-McDermid syndrome: comparison to fragile X syndrome, Down syndrome and idiopathic autism spectrum disorder. J Neurodev Disord 2017;9:37.
62. Phelan K, McDermid HE. The 22q13.3 deletion syndrome (phelan-mcdermid syndrome). Mol Syndromol 2012;2:186-201.
63. Wu L, Mei S, Yu S, Han S, Zhang YQ. Shank3 mutations enhance early neural responses to deviant tones in dogs. Cereb Cortex 2023;33:10546-57.
64. Zhao L, Li Y, Kou X, et al. Stem cells from human exfoliated deciduous teeth ameliorate autistic-like behaviors of SHANK3 mutant beagle dogs. Stem Cells Transl Med 2022;11:778-89.
65. Yang W, Guo X, Tu Z, et al. PINK1 kinase dysfunction triggers neurodegeneration in the primate brain without impacting mitochondrial homeostasis. Protein Cell 2022;13:26-46.
66. Kitada T, Pisani A, Porter DR, et al. Impaired dopamine release and synaptic plasticity in the striatum of PINK1-deficient mice. Proc Natl Acad Sci U S A 2007;104:11441-6.
67. Akundi RS, Huang Z, Eason J, et al. Increased mitochondrial calcium sensitivity and abnormal expression of innate immunity genes precede dopaminergic defects in Pink1-deficient mice. PLoS One 2011;6:e16038.
68. Xiong H, Wang D, Chen L, et al. Parkin, PINK1, and DJ-1 form a ubiquitin E3 ligase complex promoting unfolded protein degradation. J Clin Invest 2009;119:650-60.
69. Yang W, Liu Y, Tu Z, et al. CRISPR/Cas9-mediated PINK1 deletion leads to neurodegeneration in rhesus monkeys. Cell Res 2019;29:334-6.
Cite This Article
How to Cite
Download Citation
Export Citation File:
Type of Import
Tips on Downloading Citation
Citation Manager File Format
Type of Import
Direct Import: When the Direct Import option is selected (the default state), a dialogue box will give you the option to Save or Open the downloaded citation data. Choosing Open will either launch your citation manager or give you a choice of applications with which to use the metadata. The Save option saves the file locally for later use.
Indirect Import: When the Indirect Import option is selected, the metadata is displayed and may be copied and pasted as needed.
About This Article
Copyright
Data & Comments
Data
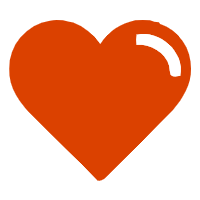
Comments
Comments must be written in English. Spam, offensive content, impersonation, and private information will not be permitted. If any comment is reported and identified as inappropriate content by OAE staff, the comment will be removed without notice. If you have any queries or need any help, please contact us at [email protected].