The role of inflammatory response in the development of atherosclerosis, myocardial infarction, and remodeling
Abstract
Inflammation is an intrinsic part of the body’s immune response, significantly influencing a myriad of physiological and pathological processes. There is now clinical and experimental evidence suggesting that inflammation accelerates atherosclerosis and its associated complications. The presence of macrophages, T and B cells inside the atherosclerotic plaque fueled this concept and steered subsequent research endeavors toward understanding the pathophysiology of atherosclerosis including plaque formation and destabilization leading to plaque rupture resulting in myocardial injury and remodeling. Understanding the mechanism behind atherosclerosis will aid in developing appropriate treatment interventions. Shifting research and drug development from a singular focus on cholesterol-lowering agents to include adjunctive anti-inflammatory therapies is crucial. Targeting a root cause, i.e., inflammation, will help decrease the incidence and progression of atherosclerosis and improve patient outcomes. In this review, we aim to discuss the current understanding of the intricate role of inflammation in the pathogenesis of atherosclerosis, myocardial infarction, and cardiac remodeling. This synthesis will encompass an exploration of the various inflammatory cells involved, the intricate network of chemokines orchestrating inflammatory responses, and the pathways that underpin these cardiovascular conditions. Furthermore, we will explore promising diagnostic and therapeutic strategies aimed at addressing inflammation in cardiovascular diseases. These include interventions such as colchicine, monoclonal antibodies, and nanoparticles designed to deliver and accumulate drugs at the molecular level within cells.
Keywords
INTRODUCTION
Atherosclerotic, a chronic inflammatory disease affecting large and medium-sized arteries, is a significant contributor to cardiovascular morbidity and mortality worldwide. Despite the importance of controlling risk factors like hypertension, diabetes, dyslipidemia, and cigarette smoking, it is crucial to understand and investigate the pathophysiology behind atherosclerosis. This chronic inflammatory process primarily affects coronary, cerebral, and peripheral vessels. The importance of inflammation in atherosclerosis is further highlighted by the fact that patients with inflammatory conditions such as rheumatoid and other inflammatory arthritis are at significantly higher risk of developing cardiovascular disease[1]. Traditionally, atherosclerosis was viewed as a process of lipid accumulation, mainly driven by low-density lipoprotein (LDL) in the arterial intima[2]. However, recent evidence from the last 25 years suggests that atherogenesis is an active inflammatory response involving both innate and adaptive immune responses, rather than a passive cholesterol accumulation process[3]. The initiation of atherosclerosis is controversial, with theories proposing lipid deposition followed by oxidation and leukocyte recruitment, or leukocyte attachment to inflamed endothelial cells, leading to lipid accumulation and macrophage foam cell formation[4,5]. Macrophage foam cells not only serve as a reservoir, but also as a source of proinflammatory and inflammatory mediators such as interleukins (IL) and tumor necrosis factor-α (TNF-α), which promote atherosclerotic plaque progression, mineralization and rupture[6].
This atherosclerotic process progresses over years and patients are usually asymptomatic during this phase. When the plaque size exceeds the capacity of the artery to accommodate outwards, the arterial lumen becomes narrow, leading to flow restriction and/or potential complications such as acute coronary syndrome due to plaque rupture or erosion. Matrix metalloproteinases secreted by macrophages cause the destruction of the extracellular matrix within the plaque, resulting in a weak fibrous cap that is prone to rupture[7]. Additionally, T-cell-mediated interferon gamma (IFN-γ) secretion from within the plaque inhibits collagen synthesis, further predisposing plaques to rupture. In several studies, toll-like receptor 2 (TLR-2) signaling has been implicated in plaque erosion by altering endothelial function[8,9].
In addition to the role of inflammation in atherosclerosis and myocardial infarction (MI), inflammation plays a crucial role in cardiac remodeling. As a result of myocardial ischemia, apoptotic and necrotic myocardial cells activate immune cells to repair damaged tissue, leading to an initial inflammatory response followed by a healing phase characterized by fibroblast activation and the release of anti-inflammatory mediators to promote tissue repair and scar formation[10]. This reparative phase is characterized by fibroblast activation and proliferation in addition to the release of inhibitory mediators such as IL-10 and transforming growth factor (TGF) to suppress inflammation and promote a profibrotic environment[11]. Understanding the complex interplay between inflammation, immune responses, and plaque stability is crucial in developing targeted therapies to prevent atherosclerotic plaque progression and reduce the risk of acute cardiovascular events like MI. Further research into the molecular and cellular mechanisms underlying atherosclerosis initiation, progression, and plaque destabilization is essential for advancing preventive and therapeutic strategies in cardiovascular medicine. This review will delve into the pivotal role of inflammatory responses in the development of atherosclerosis, myocardial infarction, and cardiac remodeling.
ATHEROSCLEROSIS, INFLAMMATION, AND THE ROLE OF THE IMMUNE RESPONSE
Atherosclerotic cardiovascular disease has a complex and incompletely understood pathogenesis, which extends beyond mere cholesterol accumulation in arterial intima[12-14]. Recent research indicates that chronic vascular inflammation, coupled with both innate and adaptive immune responses, contributes to the development of this disease[15]. A hallmark of atherosclerosis is leukocyte recruitment and penetration of endothelial cells (EC), mainly macrophages and monocytes, through leucocyte adhesion molecules (LAM) on the surface of the EC[16] such as vascular cell adhesion molecule-1 (VCAM-1) and intercellular adhesion molecule-1 (ICAM-1). This recruitment and invasion process has its roots in a complicated chain of biological responses. Oxidation of LDL secondary to hypertension, diabetes, or smoking, in combination with endothelial activation, triggers an immune response resulting in upregulation of these LAM, attracting more macrophages and monocytes to take up oxidized LDL and become foam cells[17]. This process is mediated by scavenger receptors, particularly scavenger receptor A (SR-A) and CD36, which recognize and internalize oxidized LDL[18]. This is followed by the secretion of chemokines and growth factors by both the ECs and macrophages to induce proliferation in the smooth muscle cells (SMC), which stimulates the formation of atheroma. Activated SMCs become fibroblasts, fibro-myocytes, and osteoblast-like cells[19]. These cells receive stimuli from platelet-derived growth factor and TGF-β secreted by T cells for collagen production, which contributes to fibrous cap formation. Furthermore, neutrophils increase tissue damage and enhance plaque vulnerability by initiating SMC lysis and death[20]. Cytokines, specifically TNF-α, IL-1B, IL-6, and IFN-γ, are not mere bystanders in this process. They play an integral role in every stage of atherogenesis - from the initial endothelial activation phase all through to plaque rupture. This underlines the importance of these small protein molecules in inflammation and immune responses, signifying them as potential therapeutic targets for combating atherosclerotic disease[17].
INFLAMMATION AND MYOCARDIAL INFARCTION
Acute coronary syndrome is often a consequence of a plaque rupture or ulceration within the coronary vessels. Studies have identified specific features of vulnerable plaques that predispose them to rupture, such as a necrotic core exceeding 30% of the plaque, a thin fibrous cap (< 65 µm), and significant infiltration of inflammatory cells like lymphocytes and macrophages[21].
The enlarged necrotic core is due to a lack of collagen with an accumulation of cholesterol in the center, likely due to the death of foam cells and release of the large lipid content. Necrotic cells leak intracellular components, which triggers an inflammatory response; one of these components is high-mobility group box 1 (HMGB1) molecules[22]. These molecules bind to TLR2 and TLR4, stimulating macrophages to release proinflammatory cytokines such as IL-1α, IL-1β, IL-6, and TNF-α, which in return causes more necrosis[23,24]. The stability of the plaque is dependent on a balance between the formation and degradation of the fibrous cap; if the breakdown of the ECM and collagen exceeds their formation, a thin fibrous cap is formed, which has a higher chance of rupture[19].
Thrombosis is activated upon fibrous cap rupture, superficial erosion, and coronary vasospasm. Fibrous cap rupture is the most common and accounts for 76% of men and 55% of women with fatal MI[25]. When plaque disruption occurs, thrombogenic substances will be exposed, triggering thrombus formation on the affected area[26]. It can be silent if the thrombosis is not occlusive; however, completely occlusive thrombi leads to myocardial injury in the area supplied by the occluded artery.
Moreover, studies suggest a link between sympathetic nervous system activation and myocardial infarction, with beta-adrenergic stimulation promoting emergency hematopoiesis, leucocyte migration, and mobilization, thereby contributing to the inflammatory response and myocardial healing[27,28]. In addition, it increases leucocyte migration and mobilization from medullary and extramedullary reservoirs. This emergency hematopoiesis will participate in the inflammatory response caused by myocardial ischemia and also aid in myocardial healing. This intricate interplay between inflammation, plaque vulnerability, and thrombosis underscores the multifaceted pathophysiology of myocardial infarction.
INFLAMMATION AND REMODELING
Cardiac remodeling post MI is a multifaceted process extending over months or even years that can significantly alter the structure and functionality of the heart[29]. This process has substantial prognostic implications due to its close association with heart failure. Inflammation is an essential component of tissue healing but has also been linked to pathological remodeling and the development of structural and functional changes.
Immediately after MI, ischemia leads to a rapid buildup of intracellular calcium, sodium, and hydrogen, resulting in tissue acidosis. This induces energy depletion through mitochondrial damage and triggers the release of proapoptotic signals to initiate and maintain the inflammatory process that later aids in the remodeling process[30].
Repair post MI can be categorized into three overlapping phases: the inflammatory phase, the proliferative phase, and the maturation phase[31]. During the inflammatory phase, apoptotic and necrotic cells, along with the damaged extracellular matrix, initiate an inflammatory cascade reaction, leading to the release of cytokines and chemokines[32]. Subsequently, more leucocytes get attracted to the inflammatory site to aid in the clearing dead cells and digesting extracellular matrix tissue. During the proliferative phase, macrophages and fibroblasts secrete extracellular matrix proteins to restore structural integrity and start scar formation. Switching from the activation to the suppression of inflammatory signals is not a passive process; it likely requires the intervention of inhibitory molecules that activate suppressive pathways[33]. Finally, during the maturation phase, scar maturation takes place due to the deactivation of reparative cells and the withdrawal of the fibrogenic growth factors.
Prolonged inflammation or defective suppression can have catastrophic consequences, such as loss of myocyte contractility, leading to chamber dilation, which can subsequently progress to heart failure[31]. The fibrotic response after MI can be divided into two types: replacement and reactive fibrosis. Although initial reparative fibrosis is important for preventing myocardial wall rupture, exaggerated or reactive fibrosis due to excessive deposition of extracellular matrix (ECM) can result in organ distortion and disruption of cardiac function[34]. This is defined as pathological remodeling, in which the ECM expansion leads to hypertrophy of cardiac myocytes as a trial to compensate for the increased workload by increasing in size to improve myocardial function and decrease ventricular wall tension[35].
CARDIOVASCULAR TREATMENT FOR INFLAMMATION AND FUTURE DIRECTIONS
It is currently an ongoing challenge to overcome the adverse effects of pathological remodeling post MI. Medications like angiotensin-converting enzyme inhibitors, angiotensin receptor blockers, and aldosterone antagonists are used in clinical practice for the treatment of the chronic phase of remodeling[36]. However, little is known regarding therapies targeting the acute phase of remodeling. Recently, colchicine has emerged as a potential treatment (due to its anti-inflammatory properties) to reduce cardiovascular events in patients with chronic coronary and acute coronary syndromes[37-39]. The 2023 AHA/ACC/ACCP/ASPC/NLA/PCNA Guideline for the Management of Patients with Chronic Coronary Disease suggests that in patients with chronic coronary disease, the addition of colchicine for secondary prevention may be considered to reduce recurrent cardiovascular events[40,41]. Another novel anti-inflammatory agent, Ziltivekimab, a monoclonal antibody that targets interleukin-6, is being tested in the Specifying the Anti-inflammatory Effects of Ziltivekimab (SPIDER) trial [ClinicalTrials.gov ID - NCT06263244]. Many studies propose gene therapy for the prevention and treatment of cardiac remodeling, such as B-type natriuretic peptide gene delivery, which has been used to prevent cardiac remodeling in rats[42]. Other therapies such as glucagon-like peptide and insulin-like growth factor were shown to exert anti-remodeling effects in animal models[43,44].
Recent advancements in nanomedicine research have brought to light significant progress in nanoparticle drug delivery systems, particularly in the realm of diagnosing and treating atherosclerosis. The evolution of imaging technologies has broadened the scope of research from merely identifying existing atherosclerotic plaque in symptomatic patients to characterizing asymptomatic vulnerable plaque. Nanotechnology is proving to be instrumental in the field of cardiovascular disease by facilitating the targeted accumulation of nanoparticles within atherosclerotic lesions, thereby enabling a meticulous molecular-level analysis of delicate markers and the early detection of vulnerable plaque[45].
Drug nanocarriers are commonly employed, either encapsulating drugs within their structures or on their surfaces. Various imaging nanoparticles (NPs) such as iron oxide, perfluorocarbon, gadolinium, and gold nanoparticles offer diagnostic capabilities for diverse cardiovascular conditions[46]. Beyond their diagnostic utilities, NPs hold immense potential for therapeutic applications. For instance, the successful integration of Rapamycin into leukosome nanoplatforms has demonstrated a significant reduction in proinflammatory cytokine levels and the inhibition of macrophage proliferation, thereby reshaping plaque morphology in mouse models[47,48]. Moreover, in contexts beyond atherosclerosis, certain NPs like cerium oxide have shown efficacy in mitigating oxidative stress post-myocardial infarction in murine models, thereby reducing the incidence of post-MI remodeling[49]. The constant progress in nanotechnology and nanomedicine heralds a promising future for the landscape of clinical treatments, ushering in new prospects for personalized and targeted healthcare interventions.
CONCLUSION
This review article illuminates the role of inflammation in atherosclerosis, myocardial injury, necrosis, and cardiac remodeling. The multifaceted pathophysiology of myocardial infarction underscores the intertwined roles of inflammation, plaque vulnerability, and thrombosis. Insights into the role of the immune response in atherosclerosis and inflammation highlight potential therapeutics for combating atherosclerotic disease. A meticulous understanding of post-MI cardiac remodeling processes can guide prognostic implications and the management of heart failure. Although chronic inflammatory disease indeed presents a complex and formidable challenge, it also suggests a broad and promising field for the development of innovative and efficient treatments.
DECLARATIONS
Author’s contributions
Wrote the manuscript and conducted the literature review: Botros M, Fadah K
Critically reviewed and approved the article: Botros M, Fadah K, Mukherjee D
All authors reviewed and approved of the whole manuscript.
Availability of data and materials
Not applicable
Financial support and materials
Not applicable.
Conflicts of interest
All authors declared that there are no conflicts of interest.
Ethical approval and consent to participate.
Not applicable
Consent for publication.
Not applicable
Copyright
© The Author(s) 2024
REFERENCES
1. Rodriguez T, Lehker A, Mikhailidis DP, Mukherjee D. Carotid artery pathology in inflammatory diseases. Am J Med Sci 2022;363:209-17.
2. Kong P, Cui ZY, Huang XF, Zhang DD, Guo RJ, Han M. Inflammation and atherosclerosis: signaling pathways and therapeutic intervention. Signal Transduct Target Ther 2022;7:131.
3. Pedro-Botet J, Climent E, Benaiges D. Arteriosclerosis e inflamación. Nuevos enfoques terapéuticos. Atherosclerosis and inflammation. New therapeutic approaches. Med Clin 2020;155:256-62.
4. Muller WA. How endothelial cells regulate transmigration of leukocytes in the inflammatory response. Am J Pathol 2014;184:886-96.
5. Cybulsky MI, Cheong C, Robbins CS. Macrophages and dendritic cells: partners in atherogenesis. Circ Res 2016;118:637-52.
6. Bentzon JF, Otsuka F, Virmani R, Falk E. Mechanisms of plaque formation and rupture. Circ Res 2014;114:1852-66.
7. Newby AC. Metalloproteinase production from macrophages - a perfect storm leading to atherosclerotic plaque rupture and myocardial infarction. Exp Physiol 2016;101:1327-37.
8. Franck G, Mawson T, Sausen G, et al. Flow perturbation mediates neutrophil recruitment and potentiates endothelial injury via TLR2 in mice: implications for superficial erosion. Circ Res 2017;121:31-42.
9. Quillard T, Araújo HA, Franck G, Shvartz E, Sukhova G, Libby P. TLR2 and neutrophils potentiate endothelial stress, apoptosis and detachment: implications for superficial erosion. Eur Heart J 2015;36:1394-404.
10. Mouton AJ, DeLeon-Pennell KY, Rivera Gonzalez OJ, et al. Mapping macrophage polarization over the myocardial infarction time continuum. Basic Res Cardiol 2018;113:26.
11. Frangogiannis NG. Inflammation in cardiac injury, repair and regeneration. Curr Opin Cardiol 2015;30:240-5.
12. Higashi Y. Roles of oxidative stress and inflammation in vascular endothelial dysfunction-related disease. Antioxidants 2022;11:1958.
13. Libby P, Okamoto Y, Rocha VZ, Folco E. Inflammation in atherosclerosis: transition from theory to practice. Circ J 2010;74:213-20.
14. Lavin Plaza B, Phinikaridou A, Andia ME, et al. Sustained focal vascular inflammation accelerates atherosclerosis in remote arteries. Arterioscler Thromb Vasc Biol 2020;40:2159-70.
15. Montarello NJ, Nguyen MT, Wong DTL, Nicholls SJ, Psaltis PJ. Inflammation in coronary atherosclerosis and its therapeutic implications. Cardiovasc Drugs Ther 2022;36:347-62.
16. Gimbrone MA Jr, García-Cardeña G. Endothelial cell dysfunction and the pathobiology of atherosclerosis. Circ Res 2016;118:620-36.
17. Ruparelia N, Choudhury R. Inflammation and atherosclerosis: what is on the horizon? Heart 2020;106:80-5.
18. Gusev E, Sarapultsev A. Atherosclerosis and inflammation: insights from the theory of general pathological processes. Int J Mol Sci 2023;24:7910.
19. Alonso-Herranz L, Albarrán-Juárez J, Bentzon JF. Mechanisms of fibrous cap formation in atherosclerosis. Front Cardiovasc Med 2023;10:1254114.
20. Fernández-Ruiz I. Neutrophil-driven SMC death destabilizes atherosclerotic plaques. Nat Rev Cardiol 2019;16:455.
21. Virmani R, Kolodgie FD, Burke AP, Farb A, Schwartz SM. Lessons from sudden coronary death: a comprehensive morphological classification scheme for atherosclerotic lesions. Arterioscler Thromb Vasc Biol 2000;20:1262-75.
22. Degryse B, Bonaldi T, Scaffidi P, et al. The high mobility group (HMG) boxes of the nuclear protein HMG1 induce chemotaxis and cytoskeleton reorganization in rat smooth muscle cells. J Cell Biol 2001;152:1197-206.
23. Martinet W, Schrijvers DM, De Meyer GR. Necrotic cell death in atherosclerosis. Basic Res Cardiol 2011;106:749-60.
24. Oyama J, Blais C Jr, Liu X, et al. Reduced myocardial ischemia-reperfusion injury in toll-like receptor 4-deficient mice. Circulation 2004;109:784-9.
25. Falk E, Nakano M, Bentzon JF, Finn AV, Virmani R. Update on acute coronary syndromes: the pathologists' view. Eur Heart J 2013;34:719-28.
26. Mehta LS, Beckie TM, DeVon HA, et al. Acute myocardial infarction in women: a scientific statement from the american heart association. Circulation 2016;133:916-47.
27. Swirski FK, Nahrendorf M. Leukocyte behavior in atherosclerosis, myocardial infarction, and heart failure. Science 2013;339:161-6.
28. Sager HB, Heidt T, Hulsmans M, et al. Targeting interleukin-1β reduces leukocyte production after acute myocardial infarction. Circulation 2015;132:1880-90.
29. Frantz S, Hundertmark MJ, Schulz-Menger J, Bengel FM, Bauersachs J. Left ventricular remodelling post-myocardial infarction: pathophysiology, imaging, and novel therapies. Eur Heart J 2022;43:2549-61.
30. Algoet M, Janssens S, Himmelreich U, et al. Myocardial ischemia-reperfusion injury and the influence of inflammation. Trends Cardiovasc Med 2023;33:357-66.
31. Frangogiannis NG. The inflammatory response in myocardial injury, repair, and remodelling. Nat Rev Cardiol 2014;11:255-65.
32. Arslan F, de Kleijn DP, Pasterkamp G. Innate immune signaling in cardiac ischemia. Nat Rev Cardiol 2011;8:292-300.
33. Fullerton JN, O'Brien AJ, Gilroy DW. Pathways mediating resolution of inflammation: when enough is too much. J Pathol 2013;231:8-20.
34. Maruyama K, Imanaka-Yoshida K. The pathogenesis of cardiac fibrosis: a review of recent progress. Int J Mol Sci 2022;23:2617.
35. Talman V, Ruskoaho H. Cardiac fibrosis in myocardial infarction-from repair and remodeling to regeneration. Cell Tissue Res 2016;365:563-81.
36. Wu L, Xiong X, Wu X, et al. Targeting oxidative stress and inflammation to prevent ischemia-reperfusion injury. Front Mol Neurosci 2020;13:28.
37. Paul TK, Mukherjee D. Is colchicine beneficial for the prevention of cardiovascular events after myocardial infarction? Angiology 2021;72:501-2.
38. Fujisue K, Sugamura K, Kurokawa H, et al. Colchicine improves survival, left ventricular remodeling, and chronic cardiac function after acute myocardial infarction. Circ J 2017;81:1174-82.
39. Li YW, Chen SX, Yang Y, et al. Colchicine inhibits NETs and alleviates cardiac remodeling after acute myocardial infarction. Cardiovasc Drugs Ther 2024;38:31-41.
40. Virani SS, Newby LK, Arnold SV, et al. 2023 AHA/ACC/ACCP/ASPC/NLA/PCNA guideline for the management of patients with chronic coronary disease: a report of the American heart association/American college of cardiology joint committee on clinical practice guidelines. Circulation 2023;148:e9-119.
41. Virani SS, Newby LK, Arnold SV, et al. Correction to: 2023 AHA/ACC/ACCP/ASPC/NLA/PCNA guideline for the management of patients with chronic coronary disease: a report of the American heart association/American college of cardiology joint committee on clinical practice guidelines. Circulation 2023;148:e148.
42. Cataliotti A, Tonne JM, Bellavia D, et al. Long-term cardiac pro-B-type natriuretic peptide gene delivery prevents the development of hypertensive heart disease in spontaneously hypertensive rats. Circulation 2011;123:1297-305.
43. González A, Ravassa S, Beaumont J, López B, Díez J. New targets to treat the structural remodeling of the myocardium. J Am Coll Cardiol 2011;58:1833-43.
44. Heinen A, Nederlof R, Panjwani P, et al. IGF1 treatment improves cardiac remodeling after infarction by targeting myeloid cells. Mol Ther 2019;27:46-58.
45. Lin L, Chen L, Yan J, et al. Advances of nanoparticle-mediated diagnostic and theranostic strategies for atherosclerosis. Front Bioeng Biotechnol 2023;11:1268428.
46. Wu Y, Vazquez-Prada KX, Liu Y, Whittaker AK, Zhang R, Ta HT. Recent advances in the development of theranostic nanoparticles for cardiovascular diseases. Nanotheranostics 2021;5:499-514.
47. Boada C, Zinger A, Tsao C, et al. Rapamycin-loaded biomimetic nanoparticles reverse vascular inflammation. Circ Res 2020;126:25-37.
48. Boada C, Zinger A, Tsao C, et al. Correction to: Rapamycin-loaded biomimetic nanoparticles reverse vascular inflammation. Circ Res 2020;127:e77.
Cite This Article
How to Cite
Download Citation
Export Citation File:
Type of Import
Tips on Downloading Citation
Citation Manager File Format
Type of Import
Direct Import: When the Direct Import option is selected (the default state), a dialogue box will give you the option to Save or Open the downloaded citation data. Choosing Open will either launch your citation manager or give you a choice of applications with which to use the metadata. The Save option saves the file locally for later use.
Indirect Import: When the Indirect Import option is selected, the metadata is displayed and may be copied and pasted as needed.
About This Article
Copyright
Data & Comments
Data
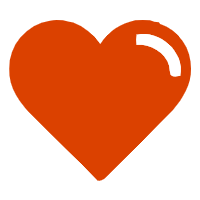
Comments
Comments must be written in English. Spam, offensive content, impersonation, and private information will not be permitted. If any comment is reported and identified as inappropriate content by OAE staff, the comment will be removed without notice. If you have any queries or need any help, please contact us at [email protected].