Strategies of cerebral protection and neurologic dysfunctions after circulatory arrest: back to the future?
Abstract
The introduction of deep hypothermic circulatory arrest (CA) was the factor that contributed to the diffusion of aortic arch surgery in the surgical world. The progressive quest to improve the outcome of such a complex surgery included the introduction of different tools to better protect the brain, such as retrograde or antegrade cerebral perfusion. The increased experience not only resulted in a continuous improvement of the results, but also facilitated the widespread adoption of arch surgery across most of the cardiac Centers. The trend moved towards a gradual rise in the temperature (from ≤ 20 to 30 °C), coupled with a preference for selective/unilateral antegrade cerebral perfusion for brain protection. Nevertheless, results are not perfect and neurologic dysfunctions, temporary or permanent, remain a frequent complication. The spinal cord is not completely protected by cerebral perfusion and ischemia of the lower body can cause organ malfunctions with severe consequences. After decades, the field is still open for new strategies to minimize the damages intrinsic to the procedure. This review will briefly describe the energetics of the brain, the mechanisms of neurologic dysfunctions, and the advantages and disadvantages of the strategies of cerebral protection commonly used during CA for aortic arch surgery.
Keywords
INTRODUCTION
The introduction of straight hypothermic circulatory arrest (CA), at 20 °C or below, was the strategy that allowed the diffusion and the improvement of the results of the surgical repair of the aortic arch. The problem of cerebral protection was explored with attention, due to the relatively high incidence of neurologic dysfunctions (NDs), permanent neurologic dysfunctions (PNDs), or temporary neurological dysfunctions (TNDs). Slowly, cerebral perfusion (CP), retrograde cerebral perfusion (RCP) or antegrade cerebral perfusion (ACP), selective antegrade cerebral perfusion (SACP) or unilateral cerebral perfusion (UCP) was added to deep hypothermic circualtory arrest (DHCA) to increase the safe time of surgery and, at the same time, the confidence of surgeons.
Over time, the concept of increasing the temperature to reduce cardiopulmonary bypass (CPB) time was pursued and the temperature at which the circulation was stopped progressively increased, reaching 28°C [moderate hypothermic circulatory arrest (MHCA)] or even higher temperatures [mild hypothermic circulatory arrest (MiHCA)]. It was evident the necessity to add ACP as cerebral protection, because, at that temperature, the safe time for the brain is very short.
The temperature limits for CA definition have recently been set in a consensus paper[1]. The different grades of hypothermia were defined as profound (≤ 14 °C), deep (14.1-20 °C), moderate (20.1-28 °C) and mild (28.1-34 °C). Nevertheless, the definition of the intervals is not uniform and can generate misunderstandings.
Then, the strategies of cerebral protection are a mixture of temperature and CP. CA can be instituted at different temperatures (from 18-20 °C to 28 °C) using no CP, UCP (with/out direct cannulation of one or two arteries), SACP (direct cannulation of two or three arteries), or RCP. Whereas no cerebral perfusion (straight DHCA) or RCP is performed at low temperatures (18-20 °C), ACP can be performed at any temperature (from 18-20 °C to 30 °C).
The advent of CP separated the CA time into two parts, one related to the brain and the other to the lower body. The cerebral CA time can vary from 0 to the full CA time, while the lower body CA normally includes the full CA period. Recently, the possibility of perfusing the lower body through the femoral artery when a frozen elephant trunk (FET) is used, using a balloon as an occluder, has been proposed[2,3], but it is still under investigation.
A recent survey[4] showed that, in Europe, DHCA alone is rarely used (6% of the cases in acute aortic syndromes and 2% in chronic cases), bilateral SACP is used in 53% of acute and in 65% of chronic, UCP in 38% of acute and 33% of chronic, RCP in 3% of acute and 0% of chronic. In one-third of the cases, the temperature was below 22 °C (till 15°) and in the remaining two-thirds included between 22 °C and 26 °C. Rarely the temperature was kept above 30 °C.
In the US, the STS database was analyzed in two different reports. In 7,830 chronic aortic pathologies, no CP was used in 32.7%, ACP in 43.0% and RCP in 24.3% of the patients. The temperature was on the low side, the highest median being in the ACP group (22.6 °C, 19.9-25.7)[5]. In 6,387 patients with ascending aorta dissection (AAD), no CP was used in 31.2%, ACP in 46.2% and RCP in 22.6% of the patients. Again, the temperature was on the low side, the highest median being in the ACP group (22 °C, 18.4-25)[6]. The STS database, although important to give an idea of the results, limits the possibility of analysis, as many data on the temperature were missing (27.5% and 28%, respectively), the definition of stroke was not clear[7], and the caseload of many Centers was low, because more than 75% of the centers perform less than three aortic arch procedures per year[8].
The ideal cerebral protection during CA is still widely debated. Many reports compare different strategies, namely DHCA without ACP and MHCA with ACP, but in such a comparison, the increasing surgical experience can be as important as the surgical strategy[9]. It is possible that techniques now considered less appealing can actually yield superior results compared to the past. This can be attributed to improved knowledge, better materials, and enhanced surgical skills.
In this review, we will briefly describe the energetics of the brain, the mechanisms of neurologic dysfunctions, and the advantages and disadvantages of the strategies of cerebral protection commonly used during CA for aortic arch surgery.
ENERGETICS OF THE BRAIN
The brain has a high metabolic demand, accounting for 20% of the total glucose and 20% of the total oxygen consumed by the human body[10]. ATP, generated primarily by glucose metabolism, is the main energy source of the brain. Glucose enters the brain from the blood through the blood-brain barrier (BBB) by means of glucose transporters. Such capillaries are continuous and non-fenestrated vessels. Their endothelial cells (ECs), being tightly connected to each other, represent a limitation to paracellular transport across the endothelium[11]. The walls of capillaries are formed as well by mural cells, which include vascular smooth muscle cells, astrocytes and pericytes, which are embedded in the basement membrane and cover the blood-brain side of the endothelial wall with their processes[12] [Figure 1]. The membranes of ECs are then divided into two sides, with different membrane composition[13,14]. As molecules must pass two sheaths of membrane to enter or leave the brain, the role of the transporters located on each side of the cell membrane is crucial in controlling this movement. Some substances, such as glutamate, a neurotrasmitter that can be toxic ay high concentrations, can only be removed from the brain.
Figure 1. Endothelial cells and pericytes are separated by the basement membrane. Pericyte processes sheathe most of the outer side of the basement membrane. At points of contact, pericytes communicate directly with endothelial cells through the synapse-like peg-socket contacts. Astrocytic endfoot processes unsheathe the microvessel wall, which is made up of endothelial cells and pericytes. Resting microglia have a "ramified" shape. In cases of neuronal disorders that have a primary vascular origin, circulating neurotoxins may cross the BBB to reach their neuronal targets, or proinflammatory signals from the vascular cells or reduced capillary blood flow may disrupt normal synaptic transmission and trigger neuronal injury (arrow 1). Microglia recruited from the blood or within the brain and the vessel wall can sense signals from neurons (arrow 2). Activated endothelium, microglia, and astrocytes signal back to neurons, which in most cases aggravates the neuronal injury (arrow 3). In the case of a primary neuronal disorder, signals from neurons are sent to the vascular cells and microglia (arrow 2), which activate the vasculo-glial unit and contribute to the progression of the disease (arrow 3). From Zlokovic with permission[11].
Glucose is transported to neurons and astrocytes. However, the presence of astrocytes endfeet that encircle blood vessels limits the capacity of the neurons to take up glucose directly. It is then possible that most of the glucose enters the brain through astrocytes[15,16] [Figure 2].
Figure 2. Astrocyte-neuron-oligodendrocyte energy metabolism interactions. Glucose in neurons is primarily used for ATP production and is metabolized into lactate in astrocytes. The glucose transporters (GLUTs) mediate the transportation of glucose into cells. GLUT1, located in cerebral endothelial cells, transits glucose from the blood into the brain tissue. GLUT1 also mediates the entrance of glucose into astrocytes and oligodendrocytes. Glucose is transported into neurons via GLUT3. Monocarboxylate transporters (MCTs) and connexin (Cx) contribute to astrocyte-neuron-oligodendrocyte lactate transport. MCT1 and MCT4 in astrocytes release lactate, which diffuses into neurons due to the lactate gradient and is actively taken up by neurons via the MCT2. Additionally, MCT1 in the myelin cooperates with MCT2 in the axon to translate the lactate from oligodendrocytes to neurons. Therefore, glucose and lactate, together with their transporters, contribute to the astrocyte-neuron-oligodendrocyte energy metabolism interactions. TCA: Tricarboxylic acid; PPP: pentose phosphate pathway; LDH: lactate dehydrogenase. From Zhang et al., with permission[128].
Once glucose enters the neurons, it can undergo the tricarboxylic acid (TCA) cycle in the presence of oxygen to generate ATP. When it enters the astrocytes, it can be metabolized through the TCA cycle or can undergo aerobic glycolysis, which is the production of lactate from pyruvate in the presence of oxygen, present in all the brain, even if not uniformly distributed[17], reaching 25% in specific regions[10]. Aerobic glycolysis and lactate production are typical of astrocytes and oligodendrocytes but are only marginally expressed in neurons[15]. Lactate obtained from aerobic glycolysis can be shuttled to neurons, where it is subsequently converted into pyruvate again, entering the TCA cycle. This shuttle hypothesis suggests that neurons use lactate, produced by astrocytes, as an energy substrate. Oligodendrocytes can transport lactates[18], produced by themselves or by astrocytes, to neurons through the myelin, or glucose, if necessary [Figure 2].
Astrocytes can store glucose as glycogen as well, serving as a metabolic reserve when glucose uptake is reduced or in stress conditions. Neurons can synthesize glycogen, which is, however, continuously degraded[19], as glycogen accumulation in neurons leads to neuronal demise[20,21]. Glycogen is rapidly broken down to lactate if neuronal activity is increased or glucose level is low. This mechanism becomes essential to maintain axon function. Glycogen stores are actively regulated by astrocytes according to glucose levels, upregulating glycogen stores if glucose level is high and depleting glycogen stores if glucose level is low.
In summary, we can reasonably suggest that glucose metabolism provides, through the generation of ATP, the energy, with different pathways, for physiological brain function, neuronal and non-neuronal cellular maintenance, and the generation of neurotransmitters.
Glutamate, one of the most important neurotransmitters in the adult central nervous system, is stored in synaptic vesicles, used by brain cells to store neurotransmitters in the cell cytoplasm[22]. Neurons exchange signals through electrical depolarization, which induces these vesicles to fuse with the cell plasma membrane, releasing the signaling molecules into the synaptic cleft in a process called exocytosis.
Strict homeostasis is crucial to ensure the beneficial effects of glutamate, maintaining glutamate in the extracellular fluid at concentrations below their toxic range. Glutamate, after being released, is taken up by surrounding astrocytes, stimulating glucose uptake and lactate production[23]. In the astrocytes, glutamate is converted to glutamine and recycled to neuronal terminals, where it is converted again into glutamate to restore the glutamate vesicular pool[24-26]. The remaining glutamate enters the TCA cycle in astrocytes after conversion to α-ketoglutarate[24-26] [Figure 3]. Alternatively, when extracellular concentrations become elevated, it can be transported inside the ECs by means of specific transporters[27]. If glutamate concentration in the ECs becomes higher than plasma levels, it is moved into the bloodstream [Figure 3].
Figure 3. Homeostasis of glutamate in neurovascular unit. Glutamate is maintained at approximately 1 mM in the brain interstitial and cerebrospinal fluid (CSF); this concentration is more than 100 higher inside brain cells (~10 mM) and synaptic vesicles (~100 mM). This is due to the presence of Na+-dependent excitatory amino-acid transporters (EAATs) not only on neuronal (EAAT1) and astroglial cells (EAAT2 and EAAT3) but also on the brain vasculature (EAAT1, EAAT2, EAAT3). In the astrocyte, glutamate enters the tricarboxylic acid (TCA) cycle or is converted to glutamine by the enzyme glutamine synthetase. Glutamine is then released to the presynaptic neuron, where it is converted to glutamate and packaged into vesicles for further release. It seems evident that the uptake of extracellular glutamate into endothelial cells (EC) via EAATs is also an important step in glutamate homeostasis. When the endothelial glutamate concentration becomes higher than the blood glutamate concentration, glutamate is transported into the blood by XG- transporters that exist only on the luminal membrane in a position that facilitates blood excretion of glutamate from the brain. ECs may also utilize glutamate as an energy substrate. EC may catalyze the conversion of glutamate to α-ketoglutarate (α-KG) and enter the TCA cycle in the mitochondria to form pyruvate, which may then be converted to lactate in the cytosol and transported through monocarboxylate transporter 1 (MCT-1) in the luminal membrane to the blood. From Castillo et al., with permission[129].
Glutamate excitotoxicity appears when the neurotransmitter’s levels become elevated in the extracellular fluid[28]. This leads to an influx of Na+ and Cl- into the postsynaptic cell, causing intracellular hyperosmolarity, and influx of water into the cell, contributing to intracellular edema and neuronal death. Furthermore, glutamate stimulates glutaminergic receptors, but their excessive activation leads to the opening of Ca++ channels. Efflux of Ca++ into neurons, which activates plasmatic proteolytic enzymes, results in neuronal death via apoptosis or necrosis[29-31].
NEUROLOGIC DYSFUNCTION AFTER CIRCULATORY ARREST
NDs after circulatory arrest can be divided into two big groups[32]. The first one is characterized by interruption of the blood flow to a cerebral area due to embolism or thrombosis (type 1). The second one is characterized by patent cerebral vessels with damage intrinsic to neurons that die during circulatory arrest (type 2). This latter pattern can affect the whole brain (non-focal encephalopathy) or part of it (focal encephalopathy) and is directly connected to cerebral protection.
NDs can be then permanent or temporary. This division does not identify the underlying cause, but it holds significant clinical relevance. Acute occlusion of a cerebral vessel causes a stroke, a type 1 ND. If the effects disappear, it can be a type 1 TND and if persistent, a type 1 PND. Generalized seizures, for instance, can disappear after one or two episodes, leaving no residual damages, falling under the classification of type 2 TND. Focal cerebral deficits can also be caused by focal neuronal death, featuring a type 2 PND. The diagnosis and the differentiation between types are often not easy, as they require cerebral angiography, which is typically a speculative procedure.
Type 1 NDs, temporary or permanent, are related to the patients and the disease and do not depend on the cerebral protection strategy. The main cause is embolism[33] from atherosclerosis or thrombi at sites of ascending aorta, aortic arch, aortic arch branches, aortic clamping, vascular anastomosis, and aortic or cerebral perfusion cannulation[34]. Cannulation for arterial inflow is the first surgical step that can cause cerebral embolism either at the moment of vessel manipulation or because of the modality of flow, as in retrograde flow through the femoral artery that can cause atherosclerotic emboli or cerebral malperfusion in AAD. Table 1 summarizes the different cannulation sites in acute and chronic pathologies.
Arterial inflow in arch surgery
Type of cannulation | Clinical presentation | Advantages | Disadvantages | |
Antegrade flow | ||||
Before the AV | ||||
Transatrial cannulation of the LV[130,131] | A | Easy and immediate approach | Cannot be performed in case of pericardial rupture the aorta cannot cross-clamped | |
Transventricular cannulation of the AAA[132,133] | A | Easy and immediate approach | Cannot be performed in case of pericardial rupture; the aorta cannot be cross-clamped; Cannulation of the true lumen can be difficult | |
After the AV | ||||
AAA cannulation[134] | A,C | Easy and immediate approach | In AAD: Needs Seldinger technique with echocardiographic guidance; Difficulty is the dissection is circumferential | |
Direct true lumen cannulation “samurai” technique[135] | A | Impossible to cannulate the false lumen | Needs to put snares around the dissected distal AA; possible rupture of the dissected aorta; Needs a (short) period of severe hypotension; Possible bleeding around the snares | |
Epiaortic cannulation through the | ||||
(A) Axillary artery | A, C | Unfrequently dissected | Time-consuming; Often needs graft interposition; Size can be inadequate and wall can be fragile; Possible brachial plexus injury | |
(B) Innominate artery | A, C | Easy and immediate approach | Can be dissected or atherosclerotic | |
(C) Carotid artery | A | Easy approach if common CA is used | Possible atherosclerosis; Cerebral hyperperfusion? Can need a separate incision | |
Retrograde flow | ||||
Femoral artery | A, C | Easy and quick access | Can cause malperfusion or extend the dissection; Can provoke atherosclerotic emboli; Can be atherosclerotic and severely diseased |
Retrograde embolization is a well-known phenomenon strictly related to femoral artery cannulation. However, axillary arterial cannulation can also lead to atheroemboli if there is a lesion at the orifice of the brachiocephalic trunk. Nevertheless, it was shown by Kim et al. that, in patients operated on with moderate hypothermia and ACP (UCP, isolated or with left common carotid artery (LCCA) perfusion), the use of axillary cannulation, if compared with other cannulation sites, was able to reduce marginally the prevalence of focal NDs (2.6% vs. 8.6%,
ACP allows continuous cerebral perfusion throughout HCA; however, the manipulation of arch vessels (including dissection, clamping, or directly cannulation of the ostia of the arch vessels) can dislodge debris from the vessels or introduce air into the cerebral circulation, causing cerebral embolism. A study based on magnetic resonance imaging demonstrated that patients with no clinical symptoms had more embolic lesions after ACP than after RCP[36].
SACP can perfuse the brain during a period of CA, but the presence of moderate or severe atherosclerotic plaques inside the common carotid arteries can cause embolic strokes. The use of UCP needs manipulation of the origin of the brachiocephalic trunk and of the LCCA (both need to be clamped with possible fragmentation of atherosclerotic plaques, if present).
A major risk factor for non-focal encephalopathy, including generalized seizures and PND[37], is leukoaraiosis[32,38]. This term describes abnormalities of the white matter, appearing as patchy or confluent subcortical and periventricular hypodensity on computed tomography or hyperintensity on magnetic resonance imaging. These silent brain lesions are often seen in elderly patients with hypertension and diabetes, with or without a prior history of stroke or dementia.
The emerging widespread addition of FET during total arch replacement seems to increase the prevalence of postoperative NDs, mostly embolic in origin. Berger et al. reported a ND rate of 16.8% in 250 patients undergoing arch replacement for acute and chronic aortic pathologies[33]. The rate rose to 35.7% in patients undergoing arch replacement for chronic aneurysms. In all cases, moderate hypothermia and bilateral SCP were routinely used. In another clinical picture, where the procedure was performed at a rectal temperature of 20-25 °C (likely DHCA) and ACP (LSA was ligated), the stroke rate was 8.6%[39]. In three meta-analyses, the prevalence of stroke was 7.6%[40], 7.7%[41], and 7.1%[42], respectively.
Spinal cord injury (SCI) can be related to a lack of LSA perfusion or the use of FET with different mechanisms. Perfusing the LSA avoids malperfusion of the left vertebral artery (steal syndrome) but also allows to perfuse continuously the anterior spinal artery via cervical and left thoracic collaterals. There are pieces of evidence in human that ACP, including LSA perfusion, only partially perfuses the upper thoracic cord via collateral circulation from vertebral arteries, but blood flow by ACP does not reach the lower thoracic cord[43]. In an experimental setting, Etz et al. also concluded that ACP provides insufficient spinal flow below T8/T9[44]. A recent anatomical study[45] emphasized the necessity of perfusing the four suppliers of spinal cord (LSA, thoracic segmental arteries, lumbar segmental arteries, and internal iliac arteries) to avoid SCI.
SCI represents a major drawback of FET surgery. Berger et al. reported a prevalence of 1.2% after FET, but other studies reported higher prevalence rates ranging from 5.6% to 24%[33,39,43,46,47]. Three meta-analyses reported a prevalence of 4.7%[40], 3.5%[42], and 6.5%[41], respectively. Lower body CA duration represents a risk factor for SCI. In a small group of patients who underwent ACP at 28 °C and lower body CA > 60 min, paraplegia rose to 18.2% vs. 1.2% when lower body CA was 60 min or less[48]. Another important point is the length of the FET. If longer than 15 cm, extending beyond T8, it is a risk factor for SCI[40]. This was demonstrated experimentally as well[49]. In addition, proximalization of FET to zone 0 can reduce SCI, thereby reducing the occlusion of the intercostal vessels[50].
During lower body CA, it is important to recognize that cooling protects the lower spinal cord better than ACP[51].
Cognitive decline has not been studied often, but it can occur in more than 20% of the patients who had no stroke by 7 days after extubation[34].
Delirium can be present alongside ischemic strokes[34]. However, when it comes to non-focal NDs, which have a multifactorial origin, determining whether delirium is an expression of encephalopathy poses a challenge. Nevertheless, its incidence can be as high as 36% in patients without postoperative stroke[34].
NEUROPROTECTION
Before circulatory arrest: straight DHCA [Table 2]
Reducing the cerebral temperature to 18-20 °C has been, in the past, the only way to protect the brain. The institution of deep hypothermia gave the possibility to repair the aortic arch, partially or totally. First proposed by Borst et al. in 1964[52], DHCA was, for a long time, and still is, a method used by many surgeons. EEG monitoring is not necessary, as it was demonstrated that most patients achieve EEG silence after 45 min of cooling when a nasopharyngeal temperature of 15-20 °C is reached[53].
Strategies of cerebral protection
T °C | Strategy | Cannulation sitea | ACP | RCP | |||||
AAA | AA | IA | FA | RCA | LCA | LSA | SVC | ||
14.0-20.0 | Straight DHCA | F | U | U | F | N | N | N | N |
w/out delayed rewarming | |||||||||
DHCA with ACP | U | Y | U | Y | Y | Y/Nb | Y/Nb | Y/N* | |
DHCA with RCP | Y | U | U | Y | N | N | N | Y | |
20.1-28.0 | MHCA with ACP | U | Y | Y | Y | Y | Y/Nb | Y/Nb | Y/N* |
MHCP with RCP** | Y | U | U | Y | N | N | N | Y | |
28.1-34.0 | MiHCA with ACP | U | Y | Y | Y | Y | Y/Nb | Y/Nb | Y/N* |
The cerebral flow is roughly 50 mL per 100 gr[54] and the energy produced is mainly required to maintain Na+ homeostasis, which is largely governed by the Na+/K+- ATPase pump[55]. Hypothermia decreases cerebral blood flow, but also cerebral oxygen consumption, glucose utilization and brain metabolism, so this decrease is not harmful. Brain metabolism reduces by 5%-6% for every degree of decrease in temperature[56].
Hypothermia significantly reduces extracellular levels of excitatory neurotransmitters[57], the release of which is temperature dependent, as even mild levels of hypothermia exert an inhibitory effect[58,59]. Another mechanism by which hypothermia promotes the survival of neurons is through the interaction with glycine. Excitatory amino acids, through receptor activation, increase Ca++ influx into the cell. Some receptors require the presence of glycine to be activated[60]. Hypothermia significantly decreases brain glycine levels after ischemia, thus decreasing hyperexcitability by glutamate[61,62].
Straight DHCA has been shown to be a safe approach at many centers. Adding ACP or RCP to DHCA does not seem to add any advantage, at least in non-complex surgery[63]. In 490 patients, including total arch replacement and AAD, Ziganshin et al. reported a prevalence of stroke of 1.6%, 1.3% (6/478) if DHCA was
Neurocognitive function after straight DHCA was explored by Chau et al. in 33 patients who did not need DHCA and 29 who did[66]. The prevalence of patients who showed some cognitive decline was not related to DHCA (13/33 vs. 11/29, P = 1.00). In patients who needed DHCA, cognitive decline was not correlated with CA time. Nevertheless, only three patients had a CA time > 40 min and all experienced cognitive decline.
In a prospective randomized study on neurocognitive function in patients where DHCA, straight or with ACP or with RCP, was used, Svensson et al. found no neurocognitive advantage in adding ACP or RCP to DHCA[67]. On the contrary, straight DHCA patients performed better than RCP patients in 5 of 7 and better than ACP patients in 9 of 9 postoperative subscores. The same group[68], analyzing 403 patients who had undergone hemiarch or arch surgery, found that the ACP added to DHCA was a risk factor for neurocognitive decline at multivariate analysis.
DURING CIRCULATORY ARREST: CEREBRAL PERFUSION
Perfusion of the brain during CA was the answer to overcome the time limits that straight DHCA imposed to reduce the prevalence of NDs. Perfusion is established antegradely or retrogradely. In the first case, the temperature of CA progressively increased, and, in many reports, CA is performed to 26 °C or even more. In the case of RCP, the temperature is maintained as low as 18-20 °C.
Antegrade cerebral perfusion
ACP could be hemispheric (through the right axillary or subclavian arteries, or innominate artery) or
Higher pressure ACP increases cerebral blood flow, but elevates intracranial pressure, causing post bypass cerebral metabolic rate of oxygen, and poorer neurobehavioral recovery[71]. Haldenwang et al., comparing various flow rates of ACP, showed that high-flow ACP significantly increases cerebral blood flow and intracranial pressure, but can result in cerebral edema with no cerebral metabolic benefit[72].
The advantage of ACP is that it can better meet the demands of brain metabolism, flush brain metabolic waste during ischemia, and better control brain temperature. ACP can be applied to higher temperatures, eliminating the need for deep hypothermia and related complications, and reducing pump time[73].
Drawbacks of ACP are mainly related to the manipulation of arch branch vessels, such as dissection, clamping, or direct cannulation of the ostia. Other possible drawbacks include the presence of many cannulas in the operative field and the possibility of malperfusion. An MRI study from Leshnower et al. showed that all patients who had MHCA + ACP (n = 9) showed a mean of 4 ± 3.5 diffusion-weighted imaging lesions per patient vs. 1.2 ± 2.1 in patients who had DHCA + RCP (P = 0.01)[36]. Moreover, few surgeons cannulate and perfuse all three vessels (innominate, LCCA, and LSA) and few surgeons occlude the LSA to prevent retrograde steal. An EEG study in patients who had MHCA + UCP showed that some patients experienced persistent loss of electrocerebral activity, with the possibility of adverse neurocognitive outcomes[74]. It was shown as well that, in patients with AAD where an aortic branch was dissected, they had more embolic strokes on the cerebral site of the dissected arch branch vessel[75]. Another point, difficult to quantify, is that CP gradually declines with time and not uniformly within the brain, making certain regions more susceptible to ischemia[76].
Results of ACP with a variety of CA temperatures have been widely explored. The ARCH database was used by Keeling et al. to report 3,265 patients undergoing total arch replacement (55% electively) with ACP and DHCA or MHCA[77]. Results were similar in propensity-matched patients (669 in each group), with a NDs rate of 17.5% in DHCA (8% permanent, 7.1% temporary, 2.4% SCI) and 18.2% in MHCA (7.8% permanent, 7.1% temporary and 3.3% SCI). The same Authors[78] reported 2,008 cases of elective arch surgery using ACP from the same database. Most of the patients were operated on using MHCA and the length of ACP varied from < 40 min (53.4%) to ≥ 60 min (21.8%) to ≥ 90 min (6.6%). The overall NDs rate was 11.6% (5.1% permanent, 5.6% temporary and 0.9% SCI), increasing from < 40 min (9.7%) to ≥ 90 min (21.0%). Multivariate hierarchical regression for moderate hypothermia shows that ACP was safe till 80 min, longer than the 65-min CA time demonstrated safe in experiments on pigs[49].
This concept was questioned by Hughes[79], who underlined that the lack of clinically evident NDs does not reflect the safety of the procedure. The GOT ICE was a randomized study that included detailed neurocognitive testing and anatomic and functional neuroimaging. It demonstrated that, after total arch replacement using ACP and three different temperature groups (≤ 20 °C, 20.1-24.0 °C and 24.1-28.9 °C), there was no difference in clinical outcome. However, cognitive function began to decline when HCA with ACP lasted > 35 min, suggesting this to be the true safe duration of HCA with ACP. Finally, the study showed the association of short durations of HCA with significant postoperative reductions in cerebral gray matter volume, cortical thickness, and regional brain functional connectivity. These findings were significantly associated with neurocognitive deficits in verbal memory and attention/concentration. Structural verbal memory showed a significant decline in high MHCA group compared with DHCA group
Anatomy of Willis’ circle
Unilateral perfusion of the brain through the right axillary artery in combination with DHCA or MHCA is a strategy commonly used in surgical repair of the aortic arch. The contralateral brain is perfused correctly only if the circle of Willis works well. A study on 250 cadavers and 250 patients undergone brain angio-CT found that in 58.6% of the cases, there were anatomic conditions for left cerebral hemisphere hypoperfusion[80]. As part of the standard preoperative assessment, extracranial and transcranial color-coded duplex sonography was conducted on 391 patients scheduled for elective arch surgery. UCP could be defined as safe in 72% of the patients, moderately safe in 18%, and unsafe in 10%[81]. This anatomic aspect seems not to translate into a worse cerebral outcome. Two recent pooled analyses, 3,723 patients receiving bilateral CP vs. 3,065 patients receiving unilateral CP[82] and 1,894 patients receiving unilateral CP vs. 3,206 patients receiving bilateral CP[83], showed no difference in the rate of PNDs, in agreement with a recent propensity-matched analysis suggesting no neurological benefit of bilateral over unilateral CP in elective aortic arch surgery[84]. It is possible that bilateral and unilateral CP entail a similar ND rate as the former can reduce cerebral damages due to hypoperfusion, but can increase the rate of embolic ND due to epiaortic vessels manipulation[81]. On the other side, Angeloni et al. observed an increased rate of mortality in UCP during circulatory arrest times exceeding 30 min[82]. Jiang et al., in 595 patients with AAD, found that patients who received unilateral (n = 276) compared with bilateral CP (n = 319) showed higher rates of NDs (17.8% vs. 9.4%, P = 0.002), in particular permanent (8.0% vs. 2.8%, P = 0.005)[85]. These findings were not confirmed by a meta-analysis from Taosudis et al. and a report by Song et al., who found no difference in NDs, permanent or temporary, between the two strategies[86,87]. On the contrary, Piperata et al., in patients operated on for AAD, found a lower rate of permanent NEs where unilateral CP was used (4% vs. 14%,
The left subclavian artery and spinal cord injury
The progressive increase in the temperature of hypothermic CA raised the problem of spinal cord protection. The question was if CP during MHCA was enough to avoid SCI or if DHCA was the most reliable tool against spinal cord ischemic damage.
The vascularization of the spine depends on several arteries. Among the epiaortic trunks, the most important is the subclavian artery by means of the anterior spinal artery (branch of the vertebral artery), the deep cervical artery (branch of the costocervical trunk), the anterior intercostal arteries (branches of the internal thoracic artery) that fully anastomose with the posterior intercostal arteries. Not all the posterior intercostal arteries come from the descending aorta, as the first two comes from the supreme intercostal artery, the first branch of the costocervical trunk. In general, cord vascularization is assured by many arteries of different caliber that anastomose each other to maintain a sufficient nutrient flow. However, the possibility of steal exists if a low resistance pathway is opened. In the case of CA, the LSA can be not perfused and not clamped, can be clamped and not perfused, or can be perfused. In all these circumstances, steal happens. In the first option, it is evident. In the second one, there is a modest flow to the cervical cord, but the thoracic and lumbar cord remain progressively without flow (the flow from the internal thoracic artery to the posterior intercostal arteries via the anterior intercostal arteries goes into the empty descending thoracic aorta). In the third option, vascularization of the cervical cord is assured, but steal still exists for the thoracic cord. Maintaining the LCA open can not only reduce spinal cord perfusion, but also cause hypoperfusion of the left cerebral hemisphere due to the steal of blood through the left vertebral artery into the empty arch.
In 18 patients undergoing total aortic arch replacement and SACP of the three epiaortic trunks, brain and spinal cord oxygen saturation levels using near-infrared spectroscopy at the forehead and along the mid-line of the back at the T3 and T10 levels were investigated[51]. When CA started (tympanic temperature lower than 25 °C), a rapid decline of the oxygen saturation in all levels was observed. After ACP was instituted, the oxygen saturation levels increased in the forehead and remained partially elevated at the upper thoracic level (T3), but continued to decline without recovery at the lower thoracic level (T10). The authors concluded that during CA, the upper thoracic spinal cord via collateral circulation from vertebral arteries is partially perfused by ACP, but the lower thoracic cord is not. Then cooling is more protective against SCI than is ACP. Even if surgeons are aware that both the circle of Willis and the feeding arteries of the spinal cord are highly variable, detailed preoperative imaging to identify anomalies is rarely performed, although postoperative SCI remains a possible complication after complex aortic repair[89], as nowadays we have no accurate modality to monitor spinal cord perfusion both intraoperatively and postoperatively when warmer temperatures during hypothermic CA are used for long intervals.
Retrograde cerebral perfusion
RCP can provide only partial perfusion of the brain and is therefore insufficient to sustain cerebral metabolism. The presence of valves in the internal jugular vein prevents a significant amount of blood from reaching the brain[90]. Then, it is not safe to increase the temperature of CA over 20 °C. RCP can help flush solid particles and air bubbles from the arteries, thus reducing embolic phenomena. However, even during normothermia, most RCP blood is shunted away from capillaries, and this shunting is increased during deep hypothermia as arteriovenous and veno-venous shunts open[91,92]. It has been demonstrated experimentally that most of the blood was shunted to the inferior vena cava and less than 1% returned to the aortic arch. Moreover, in humans, more than 20 direct veno-venous anastomoses have been described[92].
Even with these drawbacks, clinical results are in general good[93-96]. Results of DHCA associated with RCP in hemiarch replacement (n = 500) have been recently reported by Brown et al. in chronic and acute aortic pathologies[94]. Stroke rate was 2.6% vs. 6.8% in chronic and acute settings, respectively. CA time was 15(12.0-20.0) vs. 24.5(20.0-30.0) min (P < 0.001). Tanaka et al. reported 1,443 patients (non-AAD 67.5% and AAD 32.5%) operated on DHCA and RCP. Overall stroke rate was 7.8% (4.5% vs. 14.6%), with a median CA time of 23(17-35)[95]. The Authors concluded that the safe time for RCP was 30 min. The same team, however, reported in a previous paper that the stroke rate in patients with > 40 min of RCP was very low, 1.7%[93].
Neurocognitive decline was investigated in a randomized controlled trial by Harrington et al., who found that adding RCP to DHCA is associated with a high incidence of neuropsychometric deficits despite the absence of clinical deficits[97]. Differences between DHCA with ACP or RCP (60 patients, 30 in each group) were explored by Okita et al., who found similar PNDs, but higher TNDs in patients where RCP was added. There was no difference in neurocognitive tests[98].
AFTER CIRCULATORY ARREST: DELAYED REWARMING
The rationale behind the use of delayed rewarming (DR) after straight DHCA is strictly related to brain energetics.
As previously stated, a good part of the glucose enters the brain through astrocytes[15,16] [Figure 1], from where it moves to neurons as lactate that, after being converted into pyruvate, enters the TCA cycle. Oligodendrocytes can transport lactates[18] produced by themselves or by astrocytes, or glucose, if necessary, to neurons through the myelin [Figure 1]. Astrocytes store glucose as glycogen as well, an energetic reserve for the brain metabolism when glucose uptake is reduced or in stress condition.
Glutamate, released into the synaptic cleft in a process called exocytosis, is the main neurotransmitter in the adult central nervous system. Glutamate, after being released, is taken up by surrounding astrocytes, is converted to glutamine, and then is recycled to neuronal terminals, where it is converted again into glutamate to restore the glutamate pool[24-26].
In the absence of ischemia or hypoxia, subjecting astrocyte cultures to deep hypothermia and rewarming leads to a significant elevation of extracellular glutamate concentration[99]. This in vitro observation may offer an explanation to the speculations[100,101] made in previous clinical observations that extracellular excitatory amino acid concentration could be related to the increased neuronal damage observed after large temperature changes. During deep hypothermia and after rewarming, the level of extracellular glutamate continues to increase dramatically, even in the presence of normoxia, with a progressive decrease in glycogen in astrocytes[99]. It is then possible that, in normoxic conditions, astrocytes subjected to deep hypothermia and subsequent rewarming have a reduced capacity to produce energy via their oxidative phosphorylation and must then rely more on glycolysis[99]. The reduction in glycogen content decreases glycolysis activity, which inhibits glutamate uptake via the Na+-dependent glutamate transporter in cultured astrocytes[102].
The excessive amount of glutamate in the extracellular space has been suggested to be one of the determining factors involved in postoperative neuronal dysfunction in patients after CA. Mechanisms of cold and rewarming injury involve intracellular Ca++ accumulation mediated by receptors activated by glutamate excess[103] (glutamate excitotoxicity).
A strategy to overcome this metabolic deadlock was experimentally proposed by Ehrlich et al., who suggested to start rewarming after a period of cold perfusion at 20 °C after DHCA[104]. After circulatory arrest, astrocytes cannot simultaneously supply neurons with lactate and maintain extracellular glutamate levels low, as their glycogen reserves become exhausted. If the circulation resumes at a consistently low temperature, astrocytes have the ability to replenish their glycogen stores by uptaking glucose from the blood, during a period when neuronal metabolism remains unaffected by higher temperatures. Astrocytes can then remove glutamate from the extracellular fluid, eliminating the danger of neuronal death, and send lactate to neurons for their metabolic needs. When rewarming starts, glutamate in the extracellular fluid has been removed and metabolic reserves restored. In general, all organs can benefit from a period of cold perfusion before rewarming to re-establish their nutrient stores.
In a clinical setting, Di Mauro et al. found that DR (defined as 10-min cold reperfusion before rewarming) after DHCA was able to maintain a low NEs prevalence (1.6%) if CA time was ≤ 40 min in a mixture of surgery for acute (48%) or chronic (52%) aortic disease[105]. Similar results were reported by Calafiore et al. in elective patients operated on with straight DHCA and DR[106].
COMMENT
Many surgeons abandoned DHCA (with/out ACP or RCP) in favor of MHCA with ACP, showing better results that are more related to increased experience than to better cerebral protection, but there is no doubt that any technique, in experienced hands, can provide stable and good results. Moreover, the interpretation of comparative studies between strategies used in different periods is not easy to perform, and often, the degree of hypothermia is not a risk factor for postoperative NDs.
The advent of ACP allowed the diffusion of arch surgery outside specialized Centers. However, the technique is not perfect. Surgically delivered ACP is not comparable to physiologic delivered flow to the brain in the native state. The flow can be too high and unbalanced, such as when the innominate artery alone is perfused, sometimes adding the LCCA, rarely the LSA. This non-physiologic blood flow can have consequences, such as reduced focal metabolism and localized brain edema[107].
The rate of NDs after CA is the fruit of a compromise between the strategy of cerebral protection and the manipulation of the arch or of the epiaortic vessels we must work with. However, it is clear that clinical evidence is inconclusive, as brain imaging detects more events and neurocognitive tests, repeated at 6 months after surgery, provide us the possibility to better evaluate the damages related to the surgical procedure. Svensson et al., in a prospective randomized trial including 121 patients who had total arch replacement under DHCA with ACP or RCP, found that 29 patients (24%) experienced clinical NDs, cortical infarcts or deep white or gray matter changes on brain imaging, or neurocognitive changes, without difference between groups[108].
Another point of interest, not very clear in the literature, is how long a CA has to last, even for a complex arch surgery. The STS database, which includes Centers with different volumes, showed that in 7,830 chronic cases, the median CA time was 25.8 min (16.0-30.0)[5] and in acute cases 31 min (23.0-43.0)[6]. Lau et al., in 1,043 cases where DHCA + RCP was used, reported a mean CA time of 25.8 ± 11.4 min, 50 min or longer in only 4.8% of the cases[109]. Damberg et al. reported a CA time of 29.7 ± 8.8 min using straight DHCA[65]. Pearsall et al., in 307 patients who underwent hemiarch surgery, reported a median CA time of 11 min
The literature shows that neurological outcome is not related to the temperature. Recently, two papers analyzed the STS database to evaluate the incidence of NDs after circulatory arrest. Seese et al. reported 3,898 patients who underwent elective hemiarch replacement with a median CA time of 19(14-27) min and a median temperature of 24.9 °C (22-27.5)[112]. All of them had ACP, but without specific details. They found that CA time was longer in patients where the nadir temperature was ≤ 20 °C. Overall prevalence of NDs was 4.2% (3.9% PND and 0.3% TND), 1.8% had encephalopathy, 0.3% SCI and 6.1% any ND. The adjusted analysis showed that the neurologic and hemiarch composite outcome was similar independently from the temperature, whereas early mortality was lower in the higher temperatures, reflecting possibly a lower technical difficulty. A similar study was performed from the same database by Ghoreishi et al., who reported 8,937 who underwent repair for AAD[113]. Stroke prevalence was 13%. Straight DHCA was used in 29% of the patients and was not a risk factor for postoperative stroke. Similar results were obtained by the German Registry for AAD, where stroke prevalence was 9.5% and multivariable analysis showed that additional CP techniques did not add to straight DHCA[114].
Other aspects of arch surgery are still unanswered. Bleeding is often considered a complication of deep hypothermia. However, it is often the consequence of a long operation with multiple sutures. In a multicenter study, where straight DHCA was compared with MHCA with ACP[106], the rate of re-exploration for bleeding was similar. The same outcome was reported by other Authors[115]. 24-h bleeding was also similar, as reported by many Authors[106,116]. Others analyzed patients operated on with MHCA and MiHCA and did not find any difference in re-exploration for bleeding[117]. Even if it is possible to find conflicting results in the literature[118], good outcomes can be obtained with a careful strategy, independently from the temperature reached during surgery.
The effects of HCA on the kidney are widely studied. Even if lowering the temperature seems to be protective for renal function, the prevalence of acute kidney injury (AKI) or the need for temporary dialysis does not reflect this assumption completely. In conventional cardiac surgery, the prevalence of AKI, regardless of its severity, can range from 50%[119] to 81.2%[120], even if there are possible differences due to AKI definitions. Following the STS definition (3-fold increase in creatinine level, creatinine level > 4 mg/dL, or requirement for dialysis), in 3,889 patients operated on without HCA, AKI prevalence was 2%, 71% of whom needed dialysis. Nevertheless, even mild AKI has been found to be a risk factor for all-cause mortality[121]. In patients where HCA was used, many Authors found that temperature was not a risk factor for AKI or dialysis[118,122-125]. Amano et al. reported a prevalence of AKI of 26% and a need for dialysis of 8.6% in 191 cases operated with MHCA for AAD[126]. Multivariable analysis showed that increased lower body ischemic time was a risk factor for AKI. On the other side, a meta-analysis from Cao et al. showed that MHCA reduced the incidence of AKI and dialysis if compared with DHCA[127]. Opposite results were found by our group[106], where MHCA was a risk factor for dialysis at weighed logistic regression and the incidence of any AKI was 16.9% in DHCA group and 47% in MHCA group, respectively.
CONCLUSION
Cerebral protection during arch surgery is still a work in progress. The issue is complex, both in terms of pathology (chronic aneurysms or dissections) and respective surgical strategies, which can be different according to the anatomic presentation. It is then important that surgeons have in their armamentarium many strategies of cerebral protection, choosing to perfuse or not the brain, the level of hypothermia, and so on, to fit different surgical scenarios. Lacking randomized controlled trials, rarely performed and often with a limited number of patients, the most important surgical aspect is the experience of the single team. We, after having used RCP and ACP, came back to straight DHCA with DR, as we found very attractive the concept of energy recovery before rewarming. But all the techniques described in this review are underpinned by a rationale, yielding high-quality results. Perhaps a definitive answer on the most efficient strategy will not be given, at least in the next years, but we have to recognize that the enormous progress that arch surgery made in the last decades allowed the widespread diffusion of such a complex surgery, not anymore confined in selected Centers. Nevertheless, we suggest that, at least in chronic patients, it is advisable to have a better preoperative knowledge of cerebral perfusion (CT or MRI angiography) in order to discover silent conditions predisposing to thrombosis or embolization and, if necessary, to adapt a specific cerebral protection strategy, if necessary.
DECLARATIONS
Authors’ contributions
Conceptualization; formal analysis; methodology; writing - original draft; Writing - review & editing; supervision: Calafiore AM
Conceptualization; writing - review & editing: Prapas S
Formal analysis; writing - review & editing: Guarracini S, Di Marco M
Methodology; formal analysis: Lorusso R, Paparella D
Writing - review & editing: Katsavrias K
Formal analysis; methodology: Totaro A
Methodology; supervision; writing - review & editing: Di Mauro M
Availability of data and materials
Not applicable.
Financial support and sponsorship
None.
Conflicts of interest
All authors declared that there are no conflicts of interest.
Ethical approval and consent to participate
Not applicable.
Consent for publication
Not applicable.
Copyright
© The Author(s) 2023.
REFERENCES
1. Yan TD, Bannon PG, Bavaria J, et al. Consensus on hypothermia in aortic arch surgery. Ann Cardiothorac Surg 2013;2:163-8.
2. Sun X, Guo H, Liu Y, Li Y. The aortic balloon occlusion technique in total arch replacement with frozen elephant trunk. Eur J Cardiothorac Surg 2019;55:1219-21.
3. Liu Y, Shi Y, Guo H, et al. Aortic balloon occlusion technique versus moderate hypothermic circulatory arrest with antegrade cerebral perfusion in total arch replacement and frozen elephant trunk for acute type A aortic dissection. J Thorac Cardiovasc Surg 2021;161:25-33.
4. De Paulis R, Czerny M, Weltert L, et al. Current trends in cannulation and neuroprotection during surgery of the aortic arch in Europe. Eur J Cardiothorac Surg 2015;47:917-23.
5. Itagaki S, Chikwe J, Sun E, Chu D, Toyoda N, Egorova N. Impact of cerebral perfusion on outcomes of aortic surgery: the society of thoracic surgeons adult cardiac surgery database analysis. Ann Thorac Surg 2020;109:428-35.
6. O'Hara D, McLarty A, Sun E, et al. Type-a aortic dissection and cerebral perfusion: the society of thoracic surgeons database analysis. Ann Thorac Surg 2020;110:1461-7.
7. Elefteriades JA, Ziganshin BA. To perfuse or not to perfuse: that is the question. Ann Thorac Surg 2020;110:1467-8.
8. Englum BR, He X, Gulack BC, et al. Hypothermia and cerebral protection strategies in aortic arch surgery: a comparative effectiveness analysis from the STS adult cardiac surgery database. Eur J Cardiothorac Surg 2017;52:492-8.
9. Ok YJ, Kang SR, Kim HJ, Kim JB, Choo SJ. Comparative outcomes of total arch versus hemiarch repair in acute DeBakey type I aortic dissection: the impact of 21 years of experience. Eur J Cardiothorac Surg 2021;60:967-75.
10. Magistretti PJ, Allaman I. A cellular perspective on brain energy metabolism and functional imaging. Neuron 2015;86:883-901.
11. Zlokovic BV. The blood-brain barrier in health and chronic neurodegenerative disorders. Neuron 2008;57:178-201.
15. Bélanger M, Allaman I, Magistretti PJ. Brain energy metabolism: focus on astrocyte-neuron metabolic cooperation. Cell Metab 2011;14:724-38.
16. Mergenthaler P, Lindauer U, Dienel GA, Meisel A. Sugar for the brain: the role of glucose in physiological and pathological brain function. Trends Neurosci 2013;36:587-97.
17. Vaishnavi SN, Vlassenko AG, Rundle MM, Snyder AZ, Mintun MA, Raichle ME. Regional aerobic glycolysis in the human brain. Proc Natl Acad Sci USA 2010;107:17757-62.
18. Fünfschilling U, Supplie LM, Mahad D, et al. Glycolytic oligodendrocytes maintain myelin and long-term axonal integrity. Nature 2012;485:517-21.
19. Vilchez D, Ros S, Cifuentes D, et al. Mechanism suppressing glycogen synthesis in neurons and its demise in progressive myoclonus epilepsy. Nat Neurosci 2007;10:1407-13.
21. Duran J, Gruart A, García-Rocha M, Delgado-García JM, Guinovart JJ. Glycogen accumulation underlies neurodegeneration and autophagy impairment in Lafora disease. Hum Mol Genet 2014;23:3147-56.
22. Zhou Y, Danbolt NC. Glutamate as a neurotransmitter in the healthy brain. J Neural Transm 2014;121:799-817.
23. Pellerin L, Magistretti PJ. Glutamate uptake into astrocytes stimulates aerobic glycolysis: a mechanism coupling neuronal activity to glucose utilization. Proc Natl Acad Sci USA 1994;91:10625-9.
24. Bak LK, Schousboe A, Waagepetersen HS. The glutamate/GABA-glutamine cycle: aspects of transport, neurotransmitter homeostasis and ammonia transfer. J Neurochem 2006;98:641-53.
25. McKenna MC. The glutamate-glutamine cycle is not stoichiometric: fates of glutamate in brain. J Neurosci Res 2007;85:3347-58.
26. Stobart JL, Anderson CM. Multifunctional role of astrocytes as gatekeepers of neuronal energy supply. Front Cell Neurosci 2013;7:38.
27. Cohen-Kashi-Malina K, Cooper I, Teichberg VI. Mechanisms of glutamate efflux at the blood-brain barrier: involvement of glial cells. J Cereb Blood Flow Metab 2012;32:177-89.
29. Hawkins RA, Mokashi A, Dejoseph MR, Viña JR, Fernstrom JD. Glutamate permeability at the blood-brain barrier in insulinopenic and insulin-resistant rats. Metabolism 2010;59:258-66.
30. Rossi DJ, Oshima T, Attwell D. Glutamate release in severe brain ischaemia is mainly by reversed uptake. Nature 2000;403:316-21.
31. Jensen AM, Chiu SY. Differential intracellular calcium responses to glutamate in type 1 and type 2 cultured brain astrocytes. J Neurosci 1991;11:1674-84.
32. Lin R, Svensson L, Gupta R, Lytle B, Krieger D. Chronic ischemic cerebral white matter disease is a risk factor for nonfocal neurologic injury after total aortic arch replacement. J Thorac Cardiovasc Surg 2007;133:1059-65.
33. Berger T, Kreibich M, Mueller F, et al. Risk factors for stroke after total aortic arch replacement using the frozen elephant trunk technique. Interact Cardiovasc Thorac Surg 2022;34:865-71.
34. Otomo S, Maekawa K, Baba T, Goto T, Yamamoto T. Evaluation of the risk factors for neurological and neurocognitive impairment after selective cerebral perfusion in thoracic aortic surgery. J Anesth 2020;34:527-36.
35. Kim JH, Lee SH, Lee S, Youn YN, Yoo KJ, Joo HC. Axillary artery cannulation reduces early embolic stroke and mortality after open arch repair with circulatory arrest. J Thorac Cardiovasc Surg 2020;159:772-8.e4.
36. Leshnower BG, Rangaraju S, Allen JW, Stringer AY, Gleason TG, Chen EP. Deep hypothermia with retrograde cerebral perfusion versus moderate hypothermia with antegrade cerebral perfusion for arch surgery. Ann Thorac Surg 2019;107:1104-10.
37. Ikeno Y, Sasaki K, Matsueda T, et al. Impact of white matter changes on neurologic outcomes of total arch replacement using antegrade cerebral perfusion. J Thorac Cardiovasc Surg 2019;157:1350-7.e1.
38. Okada K, Omura A, Kano H, et al. Effect of atherothrombotic aorta on outcomes of total aortic arch replacement. J Thorac Cardiovasc Surg 2013;145:984-91.e1.
39. Tsutsumi K, Ishida O, Yamanaka N, Hayashi K, Hashizume K. Total aortic arch replacement using the J-graft open stent graft for distal aortic arch aneurysm: report from two centres in Japan. Interact Cardiovasc Thorac Surg 2021;33:614-21.
40. Preventza O, Liao JL, Olive JK, et al. Neurologic complications after the frozen elephant trunk procedure: a meta-analysis of more than 3000 patients. J Thorac Cardiovasc Surg 2020;160:20-33.e4.
41. Tian DH, Ha H, Joshi Y, Yan TD. Long-term outcomes of the frozen elephant trunk procedure: a systematic review. Ann Cardiothorac Surg 2020;9:144-51.
42. Rezaei Y, Bashir M, Mousavizadeh M, et al. Frozen elephant trunk in total arch replacement: a systematic review and meta-analysis of outcomes and aortic proximalization. J Card Surg 2021;36:1922-34.
43. Tsagakis K, Osswald A, Weymann A, et al. The frozen elephant trunk technique: impact of proximalization and the four-sites perfusion technique. Eur J Cardiothorac Surg 2021;61:195-203.
44. Etz CD, Luehr M, Kari FA, et al. Selective cerebral perfusion at 28 °C - is the spinal cord safe? Eur J Cardiothorac Surg 2009;36:946-55.
45. Heber UM, Mayrhofer M, Gottardi R, et al. The intraspinal arterial collateral network: a new anatomical basis for understanding and preventing paraplegia during aortic repair. Eur J Cardiothorac Surg 2021;59:137-44.
46. Flores J, Kunihara T, Shiiya N, Yoshimoto K, Matsuzaki K, Yasuda K. Extensive deployment of the stented elephant trunk is associated with an increased risk of spinal cord injury. J Thorac Cardiovasc Surg 2006;131:336-42.
47. Jakob H, Tsagakis K, Pacini D, et al. The international E-vita open registry: data sets of 274 patients. J Cardiovasc Surg 2011;52:717-23.
48. Kamiya H, Hagl C, Kropivnitskaya I, et al. The safety of moderate hypothermic lower body circulatory arrest with selective cerebral perfusion: a propensity score analysis. J Thorac Cardiovasc Surg 2007;133:501-9.
49. Honkanen HP, Mustonen C, Tuominen H, Kiviluoma K, Anttila V, Juvonen T. Spinal cord injury during selective cerebral perfusion and segmental artery occlusion: an experimental study. Interact Cardiovasc Thorac Surg 2022;34:145-52.
50. Jiang SM, Ali Hassan SM, Nguyen G, Bisleri G. Zone 0 frozen elephant trunk for type A retrograde acute aortic dissection following endovascular stenting of the arch. J Card Surg 2021;36:2124-6.
51. Kinoshita T, Yoshida H, Hachiro K, Suzuki T, Asai T. Spinal cord collateral flow during antegrade cerebral perfusion for aortic arch surgery. J Thorac Cardiovasc Surg 2020;160:37-43.
52. Borst H, Schaudig A, Rudolph W. Arteriovenous fistula of the aortic arch: repair during deep hypothermia and circulatory arrest. J Thorac Cardiovasc Surg 1964;48:443-7.
53. Bavaria JE, Pochettino A, Brinster DR, et al. New paradigms and improved results for the surgical treatment of acute type A dissection. Ann Surg 2001;234:336-42; discussion 342-3.
54. Lassen NA. Normal average value of cerebral blood flow in younger adults is 50 ml/100 g/min. J Cereb Blood Flow Metab 1985;5:347-9.
55. Astrup J, Sørensen PM, Sørensen HR. Oxygen and glucose consumption related to Na+-K+ transport in canine brain. Stroke 1981;12:726-30.
56. Hägerdal M, Harp J, Nilsson L, Siesjö BK. The effect of induced hypothermia upon oxygen consumption in the rat brain. J Neurochem 1975;24:311-6.
57. Okuda C, Saito A, Miyazaki M, Kuriyama K. Alteration of the turnover of dopamine and 5-hydroxytryptamine in rat brain associated with hypothermia. Pharmacol Biochem Behav 1986;24:79-83.
58. Illievich UM, Zornow MH, Choi KT, Scheller MS, Strnat MA. Effects of hypothermic metabolic suppression on hippocampal glutamate concentrations after transient global cerebral ischemia. Anesth Analg 1994;78:905-11.
59. Ooboshi H, Ibayashi S, Takano K, et al. Hypothermia inhibits ischemia-induced efflux of amino acids and neuronal damage in the hippocampus of aged rats. Brain Res 2000;884:23-30.
60. Johnson JW, Ascher P. Glycine potentiates the NMDA response in cultured mouse brain neurons. Nature 1987;325:529-31.
61. Baker AJ, Zornow MH, Grafe MR, et al. Hypothermia prevents ischemia-induced increases in hippocampal glycine concentrations in rabbits. Stroke 1991;22:666-73.
62. Kvrivishvili G. Glycine and neuroprotective effect of hypothermia in hypoxic-ischemic brain damage. Neuroreport 2002;13:1995-2000.
63. Kaneko T, Aranki SF, Neely RC, et al. Is there a need for adjunct cerebral protection in conjunction with deep hypothermic circulatory arrest during noncomplex hemiarch surgery? J Thorac Cardiovasc Surg 2014;148:2911-7.
64. Ziganshin BA, Rajbanshi BG, Tranquilli M, Fang H, Rizzo JA, Elefteriades JA. Straight deep hypothermic circulatory arrest for cerebral protection during aortic arch surgery: safe and effective. J Thorac Cardiovasc Surg 2014;148:888-98; discussion 898-900.
65. Damberg A, Carino D, Charilaou P, et al. Favorable late survival after aortic surgery under straight deep hypothermic circulatory arrest. J Thorac Cardiovasc Surg 2017;154:1831-9.e1.
66. Chau KH, Friedman T, Tranquilli M, Elefteriades JA. Deep hypothermic circulatory arrest effectively preserves neurocognitive function. Ann Thorac Surg 2013;96:1553-9.
67. Svensson LG, Nadolny EM, Penney DL, et al. Prospective randomized neurocognitive and S-100 study of hypothermic circulatory arrest, retrograde brain perfusion, and antegrade brain perfusion for aortic arch operations. Ann Thorac Surg 2001;71:1905-12.
68. Svensson LG, Nadolny EM, Kimmel WA. Multimodal protocol influence on stroke and neurocognitive deficit prevention after ascending/arch aortic operations. Ann Thorac Surg 2002;74:2040-6.
69. Bachet J, Guilmet D, Goudot B, et al. Antegrade cerebral perfusion with cold blood: a 13-year experience. Ann Thorac Surg 1999;67:1874-8; discussion 1891-4.
70. Di Eusanio M, Schepens MA, Morshuis WJ, et al. Brain protection using antegrade selective cerebral perfusion: a multicenter study. Ann Thorac Surg 2003;76:1181-8; discussion 1188-9.
71. Halstead JC, Meier M, Wurm M, et al. Optimizing selective cerebral perfusion: deleterious effects of high perfusion pressures. J Thorac Cardiovasc Surg 2008;135:784-91.
72. Haldenwang PL, Strauch JT, Amann I, et al. Impact of pump flow rate during selective cerebral perfusion on cerebral hemodynamics and metabolism. Ann Thorac Surg 2010;90:1975-84.
73. Kaneda T, Saga T, Onoe M, et al. Antegrade selective cerebral perfusion with mild hypothermic systemic circulatory arrest during thoracic aortic surgery. Scand Cardiovasc J 2005;39:87-90.
74. Keenan JE, Wang H, Ganapathi AM, et al. Electroencephalography during hemiarch replacement with moderate hypothermic circulatory arrest. Ann Thorac Surg 2016;101:631-7.
75. Norton EL, Wu X, Kim KM, et al. Is hemiarch replacement adequate in acute type A aortic dissection repair in patients with arch branch vessel dissection without cerebral malperfusion? J Thorac Cardiovasc Surg 2021;161:873-84.e2.
76. Falasa MP, Arnaoutakis GJ, Janelle GM, Beaver TM. Neuromonitoring and neuroprotection advances for aortic arch surgery. JTCVS Tech 2021;7:11-9.
77. Keeling WB, Tian DH, Leshnower BG, et al. Safety of moderate hypothermia with antegrade cerebral perfusion in total aortic arch replacement. Ann Thorac Surg 2018;105:54-61.
78. Keeling WB, Tian D, Farrington W, et al. Prolonged periods of antegrade cerebral perfusion are safe during elective arch surgery. Ann Thorac Surg 2023;115:387-94.
80. Papantchev V, Stoinova V, Aleksandrov A, et al. The role of Willis circle variations during unilateral selective cerebral perfusion: a study of 500 circles. Eur J Cardiothorac Surg 2013;44:743-53.
81. Smith T, Jafrancesco G, Surace G, Morshuis WJ, Tromp SC, Heijmen RH. A functional assessment of the circle of Willis before aortic arch surgery using transcranial Doppler. J Thorac Cardiovasc Surg 2019;158:1298-304.
82. Angeloni E, Melina G, Refice SK, et al. Unilateral versus bilateral antegrade cerebral protection during aortic surgery: an updated meta-analysis. Ann Thorac Surg 2015;99:2024-31.
83. Angeloni E, Benedetto U, Takkenberg JJ, et al. Unilateral versus bilateral antegrade cerebral protection during circulatory arrest in aortic surgery: a meta-analysis of 5100 patients. J Thorac Cardiovasc Surg 2014;147:60-7.
84. Zierer A, Risteski P, El-Sayed Ahmad A, Moritz A, Diegeler A, Urbanski PP. The impact of unilateral versus bilateral antegrade cerebral perfusion on surgical outcomes after aortic arch replacement: a propensity-matched analysis. J Thorac Cardiovasc Surg 2014;147:1212-7; discussion 1217-8.
85. Jiang Q, Huang K, Wang D, Xia J, Yu T, Hu S. A comparison of bilateral and unilateral cerebral perfusion for total arch replacement surgery for non-marfan, type A aortic dissection. Perfusion 2023.
86. Tasoudis PT, Varvoglis DN, Vitkos E, Ikonomidis JS, Athanasiou T. Unilateral versus bilateral anterograde cerebral perfusion in acute type A aortic dissection repair: a systematic review and meta-analysis. Perfusion 2023;38:931-8.
87. Song SJ, Kim WK, Kim TH, Song SW. Unilateral versus bilateral antegrade cerebral perfusion during surgical repair for patients with acute type A aortic dissection. JTCVS Open 2022;11:37-48.
88. Piperata A, Watanabe M, Pernot M, et al. Unilateral versus bilateral cerebral perfusion during aortic surgery for acute type A aortic dissection: a multicentre study. Eur J Cardiothorac Surg 2022;61:828-35.
89. Coselli JS, LeMaire SA, Preventza O, et al. Outcomes of 3309 thoracoabdominal aortic aneurysm repairs. J Thorac Cardiovasc Surg 2016;151:1323-37.
90. Harmon JV Jr, Edwards WD. Venous valves in subclavian and internal jugular veins. Frequency, position, and structure in 100 autopsy cases. Am J Cardiovasc Pathol 1987;1:51-4.
91. Usui A, Oohara K, Murakami F, Ooshima H, Kawamura M, Murase M. Body temperature influences regional tissue blood flow during retrograde cerebral perfusion. J Thorac Cardiovasc Surg 1997;114:440-7.
92. Boeckxstaens CJ, Flameng WJ. Retrograde cerebral perfusion does not perfuse the brain in nonhuman primates. Ann Thorac Surg 1995;60:319-27; discussion 327-8.
93. Safi HJ, Miller CC 3rd, Lee TY, Estrera AL. Repair of ascending and transverse aortic arch. J Thorac Cardiovasc Surg 2011;142:630-3.
94. Brown JA, Navid F, Serna-Gallegos D, et al. Long-term outcomes of hemiarch replacement with hypothermic circulatory arrest and retrograde cerebral perfusion. J Thorac Cardiovasc Surg 2023;166:396-406.e2.
95. Tanaka A, Chehadi M, Smith HN, et al. Deep hypothermic circulatory arrest with retrograde cerebral perfusion: how long is safe? Ann Thorac Surg 2023;116:27-33.
96. Hu Z, Wang Z, Ren Z, et al. Similar cerebral protective effectiveness of antegrade and retrograde cerebral perfusion combined with deep hypothermia circulatory arrest in aortic arch surgery: a meta-analysis and systematic review of 5060 patients. J Thorac Cardiovasc Surg 2014;148:544-60.
97. Harrington DK, Bonser M, Moss A, Heafield MT, Riddoch MJ, Bonser RS. Neuropsychometric outcome following aortic arch surgery: a prospective randomized trial of retrograde cerebral perfusion. J Thorac Cardiovasc Surg 2003;126:638-44.
98. Okita Y, Minatoya K, Tagusari O, Ando M, Nagatsuka K, Kitamura S. Prospective comparative study of brain protection in total aortic arch replacement: deep hypothermic circulatory arrest with retrograde cerebral perfusion or selective antegrade cerebral perfusion. Ann Thorac Surg 2001;72:72-9.
99. Bissonnette B, Pellerin L, Ravussin P, Daven VB, Magistretti PJ. Deep hypothermia and rewarming alters glutamate levels and glycogen content in cultured astrocytes. Anesthesiology 1999;91:1763-9.
100. Newburger JW, Jonas RA, Wernovsky G, et al. A comparison of the perioperative neurologic effects of hypothermic circulatory arrest versus low-flow cardiopulmonary bypass in infant heart surgery. N Engl J Med 1993;329:1057-64.
101. Bellinger DC, Wernovsky G, Newburger JW, et al. Cognitive development of children following early repair of transposition of the great arteries using deep hypothermic circulatory arrest. Pediatrics 1991;87:701-7.
102. Gemba T, Oshima T, Ninomiya M. Glutamate efflux via the reversal of the sodium-dependent glutamate transporter caused by glycolytic inhibition in rat cultured astrocytes. Neuroscience 1994;63:789-95.
103. Warren DE, Bickler PE, Clark JP, et al. Hypothermia and rewarming injury in hippocampal neurons involve intracellular Ca2+ and glutamate excitotoxicity. Neuroscience 2012;207:316-25.
104. Ehrlich MP, McCullough J, Wolfe D, et al. Cerebral effects of cold reperfusion after hypothermic circulatory arrest. J Thorac Cardiovasc Surg 2001;121:923-31.
105. Di Mauro M, Iacò AL, Di Lorenzo C, et al. Cold reperfusion before rewarming reduces neurological events after deep hypothermic circulatory arrest. Eur J Cardiothorac Surg 2013;43:168-73.
106. Calafiore AM, de Paulis R, Iesu S, et al. Brain and lower body protection during aortic arch surgery. J Card Surg 2022;37:4982-90.
107. Damberg A, Ziganshin BA, Elefteriades JA. Data support continued role for straight deep hypothermic circulatory arrest. J Thorac Cardiovasc Surg 2018;155:1975-6.
108. Svensson LG, Blackstone EH, Apperson-Hansen C, et al. Implications from neurologic assessment of brain protection for total arch replacement from a randomized trial. J Thorac Cardiovasc Surg 2015;150:1140-7.e11.
109. Lau C, Gaudino M, Iannacone EM, et al. Retrograde cerebral perfusion is effective for prolonged circulatory arrest in arch aneurysm repair. Ann Thorac Surg 2018;105:491-7.
110. Pearsall C, Blitzer D, Zhao Y, et al. Long-term outcome of hemiarch replacement in a proximal aortic aneurysm repair: analysis of over 1000 patients. Eur J Cardiothorac Surg 2022;62:ezab571.
111. Nakamura T, Mikamo A, Matsuno Y, et al. Impact of acute kidney injury on prognosis of chronic kidney disease after aortic arch surgery. Interact Cardiovasc Thorac Surg 2020;30:273-9.
112. Seese L, Chen EP, Badhwar V, et al. Optimal circulatory arrest temperature for aortic hemiarch replacement with antegrade brain perfusion. J Thorac Cardiovasc Surg 2023;165:1759-70.e3.
113. Ghoreishi M, Sundt TM, Cameron DE, et al. Factors associated with acute stroke after type A aortic dissection repair: an analysis of the society of thoracic surgeons national adult cardiac surgery database. J Thorac Cardiovasc Surg 2020;159:2143-54.e3.
114. Conzelmann LO, Hoffmann I, Blettner M, et al. Analysis of risk factors for neurological dysfunction in patients with acute aortic dissection type A: data from the german registry for acute aortic dissection type A (GERAADA). Eur J Cardiothorac Surg 2012;42:557-65.
115. Keenan JE, Wang H, Gulack BC, et al. Does moderate hypothermia really carry less bleeding risk than deep hypothermia for circulatory arrest? A propensity-matched comparison in hemiarch replacement. J Thorac Cardiovasc Surg 2016;152:1559-69.e2.
116. Leshnower BG, Thourani VH, Halkos ME, et al. Moderate versus deep hypothermia with unilateral selective antegrade cerebral perfusion for acute type A dissection. Ann Thorac Surg 2015;100:1563-8; discussion 1568-9.
117. Leshnower BG, Myung RJ, Thourani VH, et al. Hemiarch replacement at 28 °C: an analysis of mild and moderate hypothermia in 500 patients. Ann Thorac Surg 2012;93:1910-5; discussion 1915-6.
118. Preventza O, Coselli JS, Akvan S, et al. The impact of temperature in aortic arch surgery patients receiving antegrade cerebral perfusion for > 30 minutes: how relevant is it really? J Thorac Cardiovasc Surg 2017;153:767-76.
119. Lagny MG, Jouret F, Koch JN, et al. Incidence and outcomes of acute kidney injury after cardiac surgery using either criteria of the RIFLE classification. BMC Nephrol 2015;16:76.
120. Priyanka P, Zarbock A, Izawa J, Gleason TG, Renfurm RW, Kellum JA. The impact of acute kidney injury by serum creatinine or urine output criteria on major adverse kidney events in cardiac surgery patients. J Thorac Cardiovasc Surg 2021;162:143-51.e7.
121. Yang Y, Ma J. Mild AKI is associated with mortality of patients who underwent cardiopulmonary bypass surgery. Exp Ther Med 2020;20:2969-74.
122. Fang Z, Wang G, Liu Q, et al. Moderate and deep hypothermic circulatory arrest has a comparable effect on acute kidney injury after total arch replacement with frozen elephant trunk procedure in type A aortic dissection. Interact Cardiovasc Thorac Surg 2019;29:130-6.
123. Arnaoutakis GJ, Vallabhajosyula P, Bavaria JE, et al. The impact of deep versus moderate hypothermia on postoperative kidney function after elective aortic hemiarch repair. Ann Thorac Surg 2016;102:1313-21.
124. Zhou H, Wang G, Yang L, et al. Acute kidney injury after total arch replacement combined with frozen elephant trunk implantation: incidence, risk factors, and outcome. J Cardiothorac Vasc Anesth 2018;32:2210-7.
125. Vekstein AM, Yerokun BA, Jawitz OK, et al. Does deeper hypothermia reduce the risk of acute kidney injury after circulatory arrest for aortic arch surgery? Eur J Cardiothorac Surg 2021;60:314-21.
126. Amano K, Takami Y, Ishikawa H, et al. Lower body ischaemic time is a risk factor for acute kidney injury after surgery for type A acute aortic dissection. Interact Cardiovasc Thorac Surg 2020;30:107-12.
127. Cao L, Guo X, Jia Y, Yang L, Wang H, Yuan S. Effect of deep hypothermic circulatory arrest versus moderate hypothermic circulatory arrest in aortic arch surgery on postoperative renal function: a systematic review and meta-analysis. J Am Heart Assoc 2020;9:e017939.
128. Zhang S, Lachance BB, Mattson MP, Jia X. Glucose metabolic crosstalk and regulation in brain function and diseases. Prog Neurobiol 2021;204:102089.
129. Castillo J, Loza MI, Mirelman D, et al. A novel mechanism of neuroprotection: Blood glutamate grabber. J Cereb Blood Flow Metab 2016;36:292-301.
130. Schoeneich F, Rahimi-Barfeh A, Grothusen C, Cremer J. Transatrial left-ventricular cannulation in acute aortic dissection type A: a novel cannulation technique. Eur J Cardiothorac Surg 2015;48:e51-2.
131. Puehler T, Friedrich C, Lutter G, et al. Midterm follow-up of the transatrial-to-left ventricle cannulation for acute type A dissection. Ann Thorac Surg 2022;116:467-73.
132. Wada S, Yamamoto S, Honda J, Hiramoto A, Wada H, Hosoda Y. Transapical aortic cannulation for cardiopulmonary bypass in type A aortic dissection operations. J Thorac Cardiovasc Surg 2006;132:369-72.
133. Shimamura J, Yamamoto S, Oshima S, et al. Surgical outcomes of aortic repair via transapical cannulation and the adventitial inversion technique for acute Type A aortic dissection. Eur J Cardiothorac Surg 2018;54:369-74.
134. Juvonen T, Jormalainen M, Mustonen C, et al. Direct aortic versus supra-aortic arterial cannulation during surgery for acute type A aortic dissection. World J Surg 2023.
Cite This Article
How to Cite
Download Citation
Export Citation File:
Type of Import
Tips on Downloading Citation
Citation Manager File Format
Type of Import
Direct Import: When the Direct Import option is selected (the default state), a dialogue box will give you the option to Save or Open the downloaded citation data. Choosing Open will either launch your citation manager or give you a choice of applications with which to use the metadata. The Save option saves the file locally for later use.
Indirect Import: When the Indirect Import option is selected, the metadata is displayed and may be copied and pasted as needed.
About This Article
Special Issue
Copyright
Data & Comments
Data
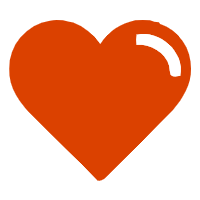
Comments
Comments must be written in English. Spam, offensive content, impersonation, and private information will not be permitted. If any comment is reported and identified as inappropriate content by OAE staff, the comment will be removed without notice. If you have any queries or need any help, please contact us at [email protected].