A multimodal approach to prevent spinal cord ischemia in patients undergoing thoracoabdominal aortic aneurism repair - from pathophysiology to anesthesiological management
Abstract
Thoraco-abdominal aortic aneurysm (TAAA) open repair is a high-risk surgery further burdened with both mortality and morbidity. Despite numerous experimental endeavors and technical advancements, spinal cord ischemia (SCI) is still the most formidable morbidity to be resolved, irrespective of the open or endovascular surgical approach. It presents a spectrum of severity, ranging from temporary or permanent paraparesis to paraplegia with or without autonomic dysfunction. The timing of SCI occurrence is a crucial factor, with approximately 15% of cases manifesting intraoperatively, 50% within 48 h post-surgery, and the remaining 35% classified as late SCI, occurring more than 48 h after the procedure. The mechanism responsible for SCI is complex and multifactorial; hence, understanding its underlying pathophysiology is essential for its effective management. Over the last decade, strategies to enhance spinal cord perfusion and minimize the risk of SCI during TAAA open repair have been implemented. These include optimization of hemodynamics, hemoglobin levels, cardiac function, and cerebrospinal fluid pressure, ensuring collateral vascular network stability and distal aortic perfusion and intrathecal administration of drugs. A multimodal approach involving anesthesiologists and surgeons can lead to improved neurological recovery and a reduced incidence and severity of SCI.
Keywords
INTRODUCTION
Spinal cord ischemia (SCI) is one of the most devastating complications in the repair of thoraco-abdominal aortic aneurysms (TAAA) in both the open and endovascular approaches. When it occurs, it carries a poor prognosis, leading to patients’ reduced quality of life, high complication and mortality rate, and prolonged duration of intensive care unit (ICU) and hospital stays[1,2]. The incidence of SCI in the repair of TAAA ranges between as low as 2%-3% and 10%, while the one-year survival of those who suffer this complication is as low as 40%[3,4]. SCI encompasses a spectrum of severity that extends from temporary or permanent paraparesis to paraplegia with or without autonomic dysfunction. SCI can manifest at various time points. Approximately 15% of cases occur intraoperatively, meaning the injury takes place during the surgical procedure. Around 50% of cases fall into the intermediate category, with symptoms manifesting within
Intermediate paraplegia, which occurs within 48 h after surgery, is more insidious in nature. Its development is intricately tied to the recruitment of the collateral network that is supplied by the hypogastric and intercostal arteries, as well as branches of the subclavian artery. The management of SCI is grounded in a deep understanding of its pathophysiology and requires a multimodal approach [Figure 1]. In fact, multiple strategies are implemented for preventing and addressing SCI, including the placement of cerebrospinal fluid (CSF) drainage, distal aortic perfusion, optimization of blood pressure, restoration of blood flow in collateral network vessels, embolization and reimplantation of segmental arteries according to the monitoring of motor (MEP) and somatosensory-evoked potentials (SSEP).
Figure 1. The multimodal approach for SCI prevention is based on MAP, CVP and CSFP modulation. MAP: Mean arterial pressure; CVP: central venous pressure; CSFP: cerebrospinal fluid pressure.
By employing these strategies, anesthesiologists and surgeons can work towards minimizing the occurrence and severity of SCI and maximizing the chances of neurological recovery[6]. The present review discusses the evolution of strategies that can be applied to maximize spinal cord perfusion and decrease the risk of SCI during thoracoabdominal aortic aneurysm repair.
VASCULAR ANATOMY OF THE SPINAL CORD
The spinal cord (SC) vasculature consists of very small vessels running in intricate, three-dimensional planes with substantial regional and inter-individual variability. SC vasculature was originally visualized with routine contrast-enhanced CT scanning back in 1994, yet information about the SC circulation has, for the most part, been gained from post-mortem studies. The first accurate anatomical descriptions were provided in 1881 by Adamkiewicz and Kady. They described the vascular system of the SC as consisting of one anterior and two posterolateral anastomotic trunks running longitudinally. Inflow vessels to the SC include: the subclavian artery (through the vertebral artery), the thyrocervical trunk, and the costocervical trunk; several segmental feeders from the intercostal and lumbar arteries; the hypogastric arteries (through the lateral sacral and iliolumbar arteries). Arteries directly nourishing the SC (intrinsic arterial system) are divided into two different systems: a central (centrifugal) system fed by the sulcal arteries; and a peripheral (centripetal) system, the pial plexus (or pial network), which gives origin to perforating branches. The pial network forms an impressive secondary anastomotic system along the entire length of the SC between the anterior and posterolateral longitudinal vessels. The anterior spinal artery (ASA) is ultimately an anastomotic channel between ascending and descending branches of neighboring anterior radicular arteries.
PATHOPHYSIOLOGY OF SPINAL CORD ISCHEMIA
Risk factors associated with spinal cord ischemia
A greater understanding of the anatomy and physiology of spinal cord perfusion has led to the recognition of several risk factors associated with spinal cord ischemia (SCI). The extent of aortic replacement is the most important predictor, with the highest rates observed for Extent II (10%-20% and Extent I TAAAs and the lowest rates for Extent IV TAAAs (1%-5%))[7-9]. In addition, occlusion of the collateral network (hypogastric and/or vertebral artery) is associated with a higher rate of immediate paraplegia and a lack of improvement in motor function following remedial maneuvers[10]. Other determinants include prior aortic replacement, chronic kidney disease, chronic obstructive pulmonary disease, and age[10,11].
Spinal cord collateral network
The artery of Adamkiewicz is a significant branch of a segmental artery (SA) found in the lower thoracic or upper lumbar region, originating from T8-L1. It possesses a distinct hairpin turn and plays a crucial role in supplying blood to the anterior spinal artery (ASA). In the treatment of extensive TAAAs, careful identification and subsequent reimplantation of the SA have been considered the most effective strategy to prevent severe neurological complications. However, the experience gained in endovascular treatment of TAAAs has shown that SCI is a potentially avoidable outcome, even when there is a significant sacrifice of the segmental arteries. This has led to a shift from the traditional anatomical paradigm, where the absence of blood flow was considered synonymous with SCI, to a pathophysiological approach. As a consequence, the need for a comprehensive understanding of spinal cord circulation is often overlooked, and the focus shifted to hemodynamic and metabolic variables as the main determinants of SCI prevention. In this approach, factors related to blood flow dynamics, such as blood pressure optimization and collateral vessel recruitment, as well as metabolic factors affecting spinal cord tissue oxygenation and energy supply, are considered crucial for preventing SCI. Lazorthes et al. detailed the multiple vessels that contributed to the spinal cord blood supply and first implemented Adamkiewicz’s original anatomical observations[12]. They described the existence of a rich collateral pathway of vessels in both the perivertebral space and alongside paraspinal muscles that anastomose outside the spinal canal, generating the spinal cord collateral network. It consists of an axial network of small arteries in the spinal canal, perivertebral tissues, and paraspinal muscles that anastomose with each other and with the nutrient arteries of the spinal cord. Inputs come from segmental, subclavian, and hypogastric arteries and their branches (originating from the internal iliac artery, namely the iliolumbar artery and lateral sacral arteries). Hence, the blood supply to the spinal cord can be either increased from one source when another is reduced or decreased when a low-resistance pathway is opened, leading to the steal phenomenon. This event is discernible and becomes apparent when utilizing an aortic cross-clamp in the thoracic district while incising the aneurysmal thoracic aorta, as noted by Christiansson and colleagues. Such action establishes a gradient between the spinal cord and the aorta, causing retrograde bleeding from the intercostal arteries into the exposed aorta. As a result, this impacts the spinal cord perfusion pressure (SCPP) and can potentially lead to anemia and hemodynamic instability[13]. Hence, there is the need to limit the back-flow from the intercostal arteries by the rapid insertion of occlusive pegs, balloons, and tourniquets in the segmental vessels within the aorta. In particular,
Mechanisms of injury
From a pathophysiological perspective, an imbalance between oxygen demand and delivery (DO2) at the spinal cord level following interruption of blood flow in the intercostal arteries during thoracic aortic surgery causes SCI. Thoracic aorta cross-clamping leads to a reduction in perfusion pressure to the vascular network supplying the central nervous system (CNS). Consequently, clamping time plays a pivotal role in the development of ischemia since its lengthening triggers cellular and molecular alterations (e.g., shift from aerobic to anaerobic metabolism), which in turn result in local and systemic alterations, ultimately leading to ischemia. Noteworthy, the combination of both a direct (interruption of blood flow) and an indirect effect which is in addition to the former (derived from the production of oxygen free radicals, lipid peroxidation, intracellular calcium accumulation, leukocyte activation, inflammatory response) ultimately results in neuronal apoptosis. More specifically, since visceral tissues in the district distal to the aortic clamp shift from an aerobic to an anaerobic metabolism, lactates are produced and cellular membranes increase their permeability, resulting in cellular swelling. Furthermore, the production of reactive oxygen species (ROS) and nitric oxide (NO) coupled with lipid peroxidation suppress adenosine triphosphate (ATP) synthesis, inactivate homeostatic metabolic enzymes, deplete antioxidant reserves, disrupt cellular and mitochondrial membranes and further boost the phenomenon of apoptosis by means of intracellular electrolytes derangement[15]. All these factors acting simultaneously lead to the destruction of the blood-spinal cord barrier, with spinal cord edema, increased leukocyte infiltration, amplification of inflammation, and oxidative stress[16]. Even reperfusion, causing inflammation, hyperemia, and edema, worsens overall cellular damage and contributes to an irreversible clinical presentation of paraplegia. In daily clinical practice, interrupting blood flow to the spinal cord does not always result in the development of SCI. Furthermore, while reimplantation of intercostal arteries during TAAA repair is desirable, it is not always feasible. Therefore, it might be plausible to replace an “anatomical paradigm” where sacrificing the intercostal arteries inevitably leads to spinal cord ischemia with a “pathophysiological” paradigm, in which spinal cord circulation is essentially ignored, and cardiac function, systemic arterial perfusion, DO2, and cellular metabolism become the therapeutic targets to prevent neurological damage [Table 1].
Physiologic determinants, target values and potential therapeutic interventions to avoid spinal cord ischemia (SCI) during thoracoabdominal aortic aneurysms (TAAA) repair
Determinant | Target value | Potential therapeutic intervention |
MAP | 85-100 mmHg | • Increase volemia • Vasoactive drugs infusion • Reduce clamping time • Gradual clamp release • Avoid abrupt pressure variations |
CSFP | 8-10 mmHg | Place external CSF drainage |
CVP | < 10 mmHg | • Implement diuretic therapy • If RV dysfunction is present: Optimize gas exchange by increasing FiO2 Moderate hyperventilation Dobutamine/adrenaline infusion |
DO2 | 1,000 mL/min | • Improve SaO2 • Keep Hb > 10 g/dL • Ensure CI > 2.5 L/min |
Body temperature | 32-34 °C | • Mild hypothermia • 300 mL cold saline infusion in each of the renal arteries • Cold saline infusion with dual-lumen catheter in epidural space |
PERIOPERATIVE MONITORING OF SPINAL CORD ISCHEMIA
Open TAAA surgery benefits from intraoperative neurophysiological monitoring (IONM) for the identification of SCI. Descending and ascending spinal pathways are evaluated by means of motor (MEPs) and somatosensory evoked potentials (SSEPs), respectively. SSEPs are elicited by stimulation of posterior tibial nerves at the ankle and/or median nerves using subdermal needle electrode pairs. Similarly, MEPs are elicited in the upper extremities with a needle electrode in the abductor pollicis brevis or the first dorsal interosseus muscle and both tibialis anterior and abductor hallucis muscles in the lower ones. Limitations of neurophysiologic monitoring include the inability to differentiate between medium and severe SCI and that it can be influenced by lower limb ischemia resulting from vascular introducers[17].
Anesthetic agents
Anesthesia shows a profound influence on evoked potentials. Volatile anesthetics reduce the amplitude of SSEP, increase their latency, and impair cortical waves; hence, their administration should not exceed 0.5 MAC[18,19]. Intravenous anesthetics (e.g., propofol and remifentanil infusions), combined with low concentrations of volatile anesthetic agents if appropriate, represent the first line of choice when the monitoring of evoked potentials is in place[20].
Neuromuscular blockers
Careful attention must also be given to the administration of neuromuscular blockers to safeguard appropriate muscle relaxation and an adequate response of MEPs. IONM also proved to be beneficial for categorizing patients based on their risk of SCI when the vascular phase of surgery is over. Bianchi et al. used a multimodal IONM approach, combining MEP, SEP, and peripheral nerve monitoring techniques in 100 consecutive patients undergoing TAAA open repair[21]. The study showed a notably higher rate of immediate postoperative motor deficits consistent with SCI, particularly in cases with irreversible MEP deteriorations compared to reversible ones. The authors concluded that implementing a multimodal IONM protocol could improve MEP interpretation and assist surgeons in making informed decisions before concluding vascular maneuvers. Although IONM has the potential to detect SCI during surgery, during the timeframe between the end of the surgical procedure and the subsequent neurological clinical evaluation, limited information about the presence of SCI is available for clinicians. In order to address this gap, von Aspern et al. have employed collateral network near-infrared spectroscopy (cnNIRS)[20]. By monitoring the perfusion of the paraspinal muscles at both thoracic and lumbar levels, cnNIRS can serve as an indirect measure of the perfusion of the spinal cord collateral network by assessing its oxygenation patterns[22]. Nevertheless, NIRS still lacks clinical validation in this setting and its routine utilization is not yet recommended[23,24].
ANESTHESIOLOGIC STRATEGIES
The main objective of anesthesia management is to avoid abrupt hemodynamic changes that can critically reduce spinal cord perfusion. The main factors that alter hemodynamics in patients undergoing TAAA are: cardiac function, the level of aortic clamping, hemoglobin levels, distal aortic perfusion with partial left heart bypass (PLHB) circulatory assistance, patient’s body temperature, intrathecal administration of medications and cerebrospinal fluid (CSF) pressure. Hence, by acting on the above-mentioned factors, a goal-directed hemodynamic strategy is mandatory for the anesthesiologist to achieve adequate spinal cord oxygenation and perfusion.
It is logical to think that mean arterial pressure (MAP) serves as the driving pressure, and higher values of systemic pressure lead to a lower risk of paraplegia. However, it is not the MAP as an “absolute value” that reduces the risk of paraplegia but the difference compared to its preoperative baseline value[5]. For instance, patients with a preoperative history of hypertension require significantly higher MAP during the perioperative period compared with normotensive patients. Within this pathophysiological approach to spinal cord perfusion, central venous pressure (CVP) also plays a quintessential role[5]. Indeed, the outflow from the spinal cord relies on the systemic venous pressure and therefore the CVP. Since the vertebral venous plexus is functionally a large single plexus with high-capacity vessels that extend along the entire spinal cord, an increase in CVP is associated with increased pressure in the vertebral venous plexus. As the spinal canal is a non-expandable space, increased pressure in the vertebral plexus directly causes an elevation of cerebrospinal fluid pressure (CSFP). The effect of elevated CVP is irrelevant when vascularization is intact but is crucial in cases of extensive sacrifice of intercostal arteries. In their experience with monitoring of MEP and SSEP during repair of TAA/A in 100 consecutive patients in whom spinal cord artery reattachment was (with 1 exception) not carried out, Etz et al. reported that spinal cord perfusion proved to be profoundly unstable under the above-mentioned conditions, with spinal cord perfusion pressure (SCPP), defined by the difference between mean arterial pressure distal to clamp (MAPd) and CSFP, being around 20 mmHg in the hours and days following surgery[25]. With such a low driving pressure value, elevated CVP can significantly increase CSFP and reduce spinal cord outflow, leading to SCI. Because spinal arteries cross the vertebral venous plexus, congestion of this plexus, secondary to high cardiac preload, exhibits a compressive effect on the segmental arteries that nourish the spinal cord.
Cardiac function
Cardiac function is the main determinant of MAP and CVP, which in turn are modulated by the right (RV) and left ventricle (LV). Transesophageal echocardiography allows for early identification of right and left heart dysfunction and its determinants (preload, contractility, or afterload).
Right ventricular dysfunction
Right ventricular (RV) dysfunction is one of the causes of CVP elevation; hence, cardiac function must be constantly monitored and supported in the event of a decline in performance. Echocardiographic criteria of RV dysfunction are summarized in Table 2.
Overall view of the main echocardiographic criteria of right and left ventricular (RV and LV, respectively) dysfunction
Criteria of ventricular dysfunction | |
Right (RV) | Left (LV) |
RVFAC < 30% Tricuspid annular plane systolic excursion < 16 mm Tissue Doppler index < 10 cm/s RV/LV > 0.6 | PAOP > 15 mmHg EF < 50% LVOT VTI < 20 cm/s with good RV function |
The RV is particularly sensitive to increased pulmonary resistance from vasoconstriction secondary to hypercapnia, hypoxia, acidosis, and polytransfusion. Therefore, the first-line treatment of RV dysfunction is the optimization of gas exchange with a high fraction of inspired oxygen (FiO2) and moderate hyperventilation.
When moderate pulmonary hypertension (Tricuspid Annular Plane Systolic Excursion [TAPSE] > 16 mm or Tissue Doppler Imaging [TDI] > 10 cm/s and Pulmonary artery systolic pressure [PAPs] > 30 mmHg) with preserved systolic function (ejection fraction [EF] > 60%) and low preload (CVP < 10 mmHg) occurs, fluid challenge is recommended. High CVP (> 15mmHg) should be treated aggressively with diuretic therapy. Poor RV contractility (TAPSE < 16 mm or TDI < 10 cm/s) may be associated with poor inotropism secondary to pre-existing coronary artery disease (CAD), and myocardial hypoperfusion due to low coronary artery gradient from left-sided dysfunction.
In cases of moderate RV dysfunction, dobutamine infusion is the first-line treatment. Adrenaline is indicated in cases of biventricular dysfunction, hypotension, or severe RV dysfunction. In patients with RV failure and low pulmonary vascular resistance but no pre-existing pulmonary hypertension, norepinephrine is effective in maintaining adequate coronary perfusion pressure (CPP). Therapeutic targets are summarized in Figure 2.
Left ventricular dysfunction
LV function is equally of paramount importance in preventing SCI. The development of low cardiac output syndrome (LCOS) with refractory and prolonged hypotension has catastrophic effects on spinal cord perfusion. Early recognition and treatment can mitigate its effects. The criteria for LV dysfunction are summarized in Table 2.
In particular, reduced ejection fraction (EF < 50%) in the postoperative period due to ischemic events or the onset of supraventricular arrhythmias is a relatively frequent cause of hypotension during the days following surgery, and may be a direct cause of the development of SCI[26].
The presence of atrial fibrillation (AF) is associated with prolonged hospitalization, higher in-hospital mortality, and reduced mid-term survival[27]. As observed by Coselli et al. in a series of more than 1,000 patients undergoing type II TAAA, the presence of preoperative CAD is one of the main predictors of SCI on multivariate analysis, increasing the risk of paraplegia in the postoperative period by 80%[26].
With regard to treatment, the evaluation of myocardial contractility and wall motion abnormalities should be conducted following preload optimization. Poor contractility is managed with adrenaline and dobutamine infusion. MAP is also critical in regard to coronary perfusion; in fact, it is not uncommon to observe MAP-dependent ST abnormalities.
If increased afterload is associated with systolic ventricular dysfunction, infusion of inodilators is indicated. Conversely, increased afterload associated with normal systolic contraction benefits from the use of short-acting vasodilators. The therapeutic targets are summarized in Figure 3.
Aortic clamping
Pressure changes can be extreme during clamping, yielding different effects on systemic blood pressure depending on the level of aortic clamping[28].
In particular, if the clamping occurs at the supraceliac level, blood is displaced from the vascular bed distal to the aortic clamping to that proximal to the clamping, due to decreased venous capacitance and the venoconstrictor effect exhibited by catecholamines[29]. Conversely, if the clamping is subceliac, the blood moves into the splanchnic circulation, causing a decrease in cardiac preload if the splanchnic venous tone is low, or an increase in preload if the venous tone is high[30]. The increase in preload and afterload as a result of aortic clamping leads to an increase in cardiac contractility and cardiac output[29].The increase in left ventricular end-systole (LV ESP) and end-diastole pressure (LV EDP) as a result of increased aortic impedance leads to transient subendocardial ischemia that stimulates increased coronary flow and contractility (ARPNER’s effect)[31]. If coronary flow reserve (CFR) is poor (e.g., due to pre-existing coronary artery disease), the patient will not be able to increase coronary flow, resulting in low flow, cardiac ischemia, and systemic hypotension, which correlate with an increased risk of SCI.
An early etiological diagnosis with intraoperative transesophageal echocardiography makes it possible to distinguish whether the hypotension is caused by hypovolemia, cardiac dysfunction, or peripheral vasodilatation[32]. Based on this, it may be indicated to administer vasodilators to reduce afterload, preload and induce coronary vasodilation, to increase cardiac contractility with inotropic drugs, or simply to expand the blood volume with fluids in the case of reduced preload.
At the time of declamping, because of volume redistribution in the lower limbs and wash-out of vasoactive and myocardial-depressor metabolites from the ischemic areas, hypotension sets in, resulting in central hypovolemia and reduced cardiac output[28]. At this stage, the risk of SCI is particularly high due to the decrease in SCPP secondary to decreased MAP and active bleeding. Hypotension following declamping can be corrected through various means, including increasing blood volume, infusing vasoactive drugs, reducing the clamp time, or employing a gradual release of the clamp. Rapid treatment of metabolic alterations may prove useful.
Partial left heart bypass
To date, partial left heart bypass (PLHB) is considered the best technique to reduce ischemia time in tissues vascularized by arteries originating downstream of the aortic clamp, to support left ventricular function and to control hypertension during TAAA correction surgery[33].
Dynamic use of PLHB makes it possible to modulate the perfusion pressure in the district proximal and distal to the clamp by keeping it between 85 mmHg and 100 mmHg for the whole duration of the surgical procedure and ensuring adequate SCPP. If the MAP falls below 60 mmHg, the perfusion pressure in the collateral medullary network is proportionally reduced. The use of PLHB has been associated with a reduced risk of paraplegia[34]. Safi et al. report that the use of PLHB in patients undergoing TAAA repair also improves the outcome in Crawford type I and II by reducing the risk of paraplegia when used in combination with CSF drainage[35]. Furthermore, although heparin is routinely administered to prevent intraoperative thrombosis, when PLHB is used, a higher degree of anticoagulation compared with the “clamp-and-go” technique is required. This may eventually worsen both the underlying TAAA-related coagulopathy and bleeding.
Cerebrospinal fluid drainage
Cerebrospinal fluid (CSF) drainage is the most effective procedure in preventing SCI after aortic repair[36]. The pathophysiological rationale behind the use of CSF drainage is the need to lower cerebrospinal fluid pressure (CSFP) by increasing SCPP. In light of this, the use of CSF drainage has entered clinical practice. CSF drainage positioning was found to significantly reduce the risk of paraplegia in a randomized controlled trial of 145 patients undergoing extent I and II TAA surgery[37]. These data are further supported by clinical practice. The use of CSF drainage as a rescue in patients who have developed neurological deficits following surgical interventions is able to reverse paraplegia and paresis by decreasing the CSFP. Hence, its use has become an essential tool in the multimodal approach for the prevention of SCI, in combination with the above-mentioned hemodynamic optimization strategies. Preventing catheter obstruction and deflecting any complications may be achieved by continuous monitoring of CSFP, even if this procedure carries its own risks and complications. The risk of developing subarachnoid hemorrhage due to excessive loss of CSF in a short time is lower with intermittent CSF drainage compared to continuous CSF drainage. Meningitis (0.1%), subdural hematoma (1.7%), and intracranial hemorrhage (1.8%) are the most severe complications[38], while subdural hematoma is associated with excessive CSF drainage and tearing of the dural veins. A CSF pressure ≥ 10 mmHg is recommended in the absence of ischemia or to perform an intermittent drainage of 10-20 mL/h with continuous systemic blood pressure monitoring. Patients with cerebral atrophy, arteriovenous malformations, brain aneurysms, and a history of previous subdural hematoma are particularly prone to developing cerebral hemorrhage[39].
Anemia
Monitoring of hemoglobin levels is particularly relevant as it directly affects DO2. In situations where cardiac output is suboptimal, maintaining maximum arterial oxygen saturation and hemoglobin levels above 10 g/dL can be beneficial[40]. The combination of bleeding, anemia, and hypotension can worsen SCI. A decrease in the oxygen content of arterial blood (CaO2) leads to a reduction in the ratio of DO2 to oxygen consumption (VO2) < 2. When DO2 falls below this threshold, VO2 becomes dependent on DO2, leading to a shift from aerobic to anaerobic metabolism, resulting in lactate production and metabolic acidosis. Thus, it is crucial to manage bleeding by ensuring meticulous surgical hemostasis and employing viscoelastic tests. Studies have shown that the use of rotational thromboelastometry significantly reduces the need for fresh frozen plasma (FFP) administration. Reduced FFP usage is associated with less hemodilution and anemia, which can help mitigate the negative effects on oxygen delivery and prevent further complications of SCI[41].
Hypothermia
Several experimental studies have demonstrated the protective effect of mild hypothermia on the prevention of SCI by reducing both nerve tissue metabolism and the release of excitatory neurotransmitters[42]. This constitutes the pathophysiological rationale for the use of regional hypothermia during aortic clamping[43]. Cambria et al. report a protective effect of regional hypothermia of the spinal cord through epidural cooling when it is used in combination with CSF drainage and intercostal artery repletion[44]. Shimizu et al. confirm these findings but propose the use of a double-lumen catheter inserted into the peridural space into which cold saline is infused[45].The risk of inserting a catheter of significant size (16 gouges in diameter and 30 cm in length) into the peridural space, together with disputed scientific evidence, has ultimately limited the use of techniques for regional cooling of the medulla to local experience.
Intrathecal drugs
Intrathecal administration of different drugs has led to inconclusive results so far. Lima et al. reported that in 330 patients undergoing TAAA surgery, the addition of 30 mg of papaverine 1% intrathecally 10 min before aortic clamping exhibited neuroprotective effects[46]. The rationale is its vasodilatory effect on the arterial circulation, which would increase blood flow to the spinal cord. However, its short duration of action and the risk of hypotension associated with possible systemic reabsorption have limited its use over time. Other authors have proposed the use of naloxone, which would have a neuroprotective effect by decreasing the level of endorphins released during SCI[47]. In particular, Acher et al. observed a significant reduction in SCI in the 49 patients in whom naloxone was used in conjunction with CSF drainage[47]. Other drugs such as calcium antagonists, N-methyl-d-aspartate, and edaravone were in animal models, but none have provided results convincing enough to be implemented in clinical trials[48,49].
CONCLUSION
TAAA repair carries substantial perioperative risks, with spinal cord ischemia being the most common complication leading to paraplegia. Therefore, each scheduled surgery case requires thorough discussions between surgeons and anesthesiologists to carefully weigh the benefits and risks specific to the patient’s situation. Several strategies (e.g., perfusion, metabolism, and oxygen delivery to the spinal cord) can be employed to mitigate the risk of spinal cord ischemia. These are based on acknowledging the most important factors in protecting the spinal cord during and after thoracic and thoracoabdominal aortic replacement, both when spinal cord blood flow is significantly reduced and after aortic replacement, while the co-axial collateral network is recruited to restore resting blood flow to near-normal levels. The anesthesiologist must ensure adequate spinal cord perfusion by elevating MAP within the range of
Figure 4. Multimodal therapeutic algorithm to treat spinal cord ischemia. CSF: Cerebrospinal fluid; CSFP: cerebrospinal fluid pressure; SpO2: peripheral capillary oxygen saturation; Hb: hemoglobin; MAP: mean arterial pressure; CVP: central venous pressure; CI: cardiac index; BSA: body surface area; SCPP; spinal cord perfusion pressure.
DECLARATIONS
Authors’ contributions
Designed the work, drafted the manuscript and revised it critically: Monaco F
Designed the work, collected and analyzed the data and drafted the manuscript: D’Andria Ursoleo J
Collected and analyzed the data and revised the manuscript critically: Barucco G, Licheri M, Faustini C, Lazzari S
Collected and analyzed the data and drafted the manuscript: Di Prima AL
All authors gave final approval of the version to be published and agree to be accountable for all aspects of the work in ensuring that questions related to the accuracy or integrity of any part of the work are appropriately investigated and resolved.
Availability of data and materials
Not applicable.
Financial support and sponsorship
None.
Conflicts of interest
All authors declared that there are no conflicts of interest.
Ethical approval and consent to participate
Not applicable.
Consent for publication
Not applicable.
Copyright
© The Author(s) 2023.
REFERENCES
1. Svensson LG, Crawford ES, Hess KR, Coselli JS, Safi HJ. Experience with 1,509 patients undergoing thoracoabdominal aortic operations. J Vasc Surg 1993;17:357-68.
2. Greenberg RK, Lu Q, Roselli EE, et al. Contemporary analysis of descending thoracic and thoracoabdominal aneurysm repair: a comparison of endovascular and open techniques. Circulation 2008;118:808-17.
3. Rocha RV, Lindsay TF, Nasir D, et al. Risk factors associated with long-term mortality and complications after thoracoabdominal aortic aneurysm repair. J Vasc Surg 2022;75:1135-41.E3.
4. Robertson CE, Brown RD Jr, Wijdicks EF, Rabinstein AA. Recovery after spinal cord infarcts: long-term outcome in 115 patients. Neurology 2012;78:114-21.
5. Etz CD, Luehr M, Kari FA, et al. Paraplegia after extensive thoracic and thoracoabdominal aortic aneurysm repair: does critical spinal cord ischemia occur postoperatively? J Thorac Cardiovasc Surg 2008;135:324-30.
6. Chung JC, Lodewyks CL, Forbes TL, et al. Prevention and management of spinal cord ischemia following aortic surgery: a survey of contemporary practice. J Thorac Cardiovasc Surg 2022;163:16-23.E7.
7. Feezor RJ, Martin TD, Hess PJ Jr, et al. Extent of aortic coverage and incidence of spinal cord ischemia after thoracic endovascular aneurysm repair. Ann Thorac Surg 2008;86:1809-14.
8. Bisdas T, Panuccio G, Sugimoto M, Torsello G, Austermann M. Risk factors for spinal cord ischemia after endovascular repair of thoracoabdominal aortic aneurysms. J Vasc Surg 2015;61:1408-16.
9. Dias NV, Sonesson B, Kristmundsson T, Holm H, Resch T. Short-term outcome of spinal cord ischemia after endovascular repair of thoracoabdominal aortic aneurysms. Eur J Vasc Endovasc Surg 2015;49:403-9.
10. Eagleton MJ, Shah S, Petkosevek D, Mastracci TM, Greenberg RK. Hypogastric and subclavian artery patency affects onset and recovery of spinal cord ischemia associated with aortic endografting. J Vasc Surg 2014;59:89-94.
11. Spanos K, Kölbel T, Kubitz JC, et al. Risk of spinal cord ischemia after fenestrated or branched endovascular repair of complex aortic aneurysms. J Vasc Surg 2019;69:357-66.
12. Lazorthes G, Gouaze A, Zadeh JO, Santini JJ, Lazorthes Y, Burdin P. Arterial vascularization of the spinal cord. Recent studies of the anastomotic substitution pathways. J Neurosurg 1971;35:253-62.
13. Christiansson L, Ulus AT, Hellberg A, Bergqvist D, Wiklund L, Karacagil S. Aspects of the spinal cord circulation as assessed by intrathecal oxygen tension monitoring during various arterial interruptions in the pig. J Thorac Cardiovasc Surg 2001;121:762-72.
14. Griepp RB, Ergin MA, Galla JD, et al. Looking for the artery of Adamkiewicz: a quest to minimize paraplegia after operations for aneurysms of the descending thoracic and thoracoabdominal aorta. J Thorac Cardiovasc Surg 1996;112:1202-13.
15. Monaco F, Gaia B, Cristina M, Monica DL. Physiopathology of intraoperative visceral ischemia and anesthesiological management of supravisceral aortic clamping. In: Tshomba Y, Baccellieri D, Chiesa R, editors. Visceral vessels and aortic repair. Cham: Springer International Publishing; 2019. pp. 147-61.
16. Deery SE, Lancaster RT, Baril DT, et al. Contemporary outcomes of open complex abdominal aortic aneurysm repair. J Vasc Surg 2016;63:1195-200.
17. Haghighi SS, Sirintrapun SJ, Johnson JC, Keller BP, Oro JJ. Suppression of spinal and cortical somatosensory evoked potentials by desflurane anesthesia. J Neurosurg Anesthesiol 1996;8:148-53.
18. Malcharek MJ, Loeffler S, Schiefer D, et al. Transcranial motor evoked potentials during anesthesia with desflurane versus propofol - a prospective randomized trial. Clin Neurophysiol 2015;126:1825-32.
19. Husain AM, Swaminathan M, McCann RL, Hughes GC. Neurophysiologic intraoperative monitoring during endovascular stent graft repair of the descending thoracic aorta. J Clin Neurophysiol 2007;24:328-35.
20. von Aspern K, Haunschild J, Hoyer A, et al. Non-invasive spinal cord oxygenation monitoring: validating collateral network near-infrared spectroscopy for thoracoabdominal aortic aneurysm repair. Eur J Cardiothorac Surg 2016;50:675-83.
21. Bianchi F, Cursi M, Caravati H, et al. Intraoperative neurophysiologic monitoring in thoracoabdominal aortic aneurysm surgery can provide real-time feedback for strategic decision making. Neurophysiol Clin 2022;52:232-41.
22. Giustiniano E, Battistini GM, Piccirillo F, et al. May near infra-red spectroscopy and rapid perfusion pressure recovering be enough to rule out post-operative spinal cord injury? Two compared case-reports. J Clin Monit Comput 2020;34:955-9.
23. Bobadilla JL, Wynn M, Tefera G, Acher CW. Low incidence of paraplegia after thoracic endovascular aneurysm repair with proactive spinal cord protective protocols. J Vasc Surg 2013;57:1537-42.
24. Luehr M, Bachet J, Mohr FW, Etz CD. Modern temperature management in aortic arch surgery: the dilemma of moderate hypothermia. Eur J Cardiothorac Surg 2014;45:27-39.
25. Etz CD, Halstead JC, Spielvogel D, et al. Thoracic and thoracoabdominal aneurysm repair: is reimplantation of spinal cord arteries a waste of time? Ann Thorac Surg 2006;82:1670-7.
26. Coselli JS, Green SY, Price MD, Zhang Q, Preventza O, de la Cruz KI. Spinal cord deficit after 1114 extent II open thoracoabdominal aortic aneurysm repairs. J Vasc Surg 2019;70:329.
27. Dolapoglu A, Volguina IV, Price MD, Green SY, Coselli JS, LeMaire SA. Cardiac arrhythmia after open thoracoabdominal aortic aneurysm repair. Ann Thorac Surg 2017;104:854-60.
28. Zammert M, Gelman S. The pathophysiology of aortic cross-clamping. Best Pract Res Clin Anaesthesiol 2016;30:257-69.
29. Gelman S. The pathophysiology of aortic cross-clamping and unclamping. Anesthesiology 1995;82:1026-57.
30. Giulini SM, Bonardelli S, Portolani N, et al. Suprarenal aortic cross-clamping in elective abdominal aortic aneurysm surgery. Eur J Vasc Endovasc Surg 2000;20:286-9.
31. Cingolani HE, Pérez NG, Cingolani OH, Ennis IL. The Anrep effect: 100 years later. Am J Physiol Heart Circ Physiol 2013;304:H175-82.
32. Kahn RA, Stone ME, Moskowitz DM. Anesthetic consideration for descending thoracic aortic aneurysm repair. Semin Cardiothorac Vasc Anesth 2007;11:205-23.
33. Coselli JS. The use of left heart bypass in the repair of thoracoabdominal aortic aneurysms: current techniques and results. Semin Thorac Cardiovasc Surg 2003;15:326-32.
34. Safi HJ, Estrera AL, Miller CC, et al. Evolution of risk for neurologic deficit after descending and thoracoabdominal aortic repair. Ann Thorac Surg 2005;80:2173-9.
35. Safi HJ, Hess KR, Randel M, et al. Cerebrospinal fluid drainage and distal aortic perfusion: reducing neurologic complications in repair of thoracoabdominal aortic aneurysm types I and II. J Vasc Surg 1996;23:223-9.
36. Hiratzka LF, Bakris GL, Beckman JA, et al. 2010 ACCF/AHA/AATS/ACR/ASA/SCA/SCAI/SIR/STS/SVM guidelines for the diagnosis and management of patients with thoracic aortic disease: executive summary. A report of the American college of cardiology foundation/American heart association task force on practice guidelines, American association for thoracic surgery, American college of radiology, American stroke association, society of cardiovascular anesthesiologists, society for cardiovascular angiography and interventions, society of interventional radiology, society of thoracic surgeons, and society for vascular medicine. Catheter Cardiovasc Interv 2010;76:E43-86.
37. Coselli JS, LeMaire SA, Köksoy C, Schmittling ZC, Curling PE. Cerebrospinal fluid drainage reduces paraplegia after thoracoabdominal aortic aneurysm repair: results of a randomized clinical trial. J Vasc Surg 2002;35:631-9.
38. Hanna JM, Andersen ND, Aziz H, Shah AA, McCann RL, Hughes GC. Results with selective preoperative lumbar drain placement for thoracic endovascular aortic repair. Ann Thorac Surg 2013;95:1968-74.
39. Weaver KD, Wiseman DB, Farber M, Ewend MG, Marston W, Keagy BA. Complications of lumbar drainage after thoracoabdominal aortic aneurysm repair. J Vasc Surg 2001;34:623-7.
40. Wu WC, Rathore SS, Wang Y, Radford MJ, Krumholz HM. Blood transfusion in elderly patients with acute myocardial infarction. N Engl J Med 2001;345:1230-6.
41. Monaco F, Barucco G, Nardelli P, et al. Editor's Choice - a rotational thromboelastometry driven transfusion strategy reduces allogenic blood transfusion during open thoraco-abdominal aortic aneurysm repair: a propensity score matched study. Eur J Vasc Endovasc Surg 2019;58:13-22.
42. Ginsberg MD, Globus MY, Dietrich WD, Busto R. Chapter 2 temperature modulation of ischemic brain injury - a synthesis of recent advances. In: Kogure K, Hossmann KA, Siesjö BK, Editors. Progress in brain research, neurobiology of ischemic brain damage. Amsterdam: Elsevier; 1993. pp. 13-22.
43. Salzano RP Jr, Ellison LH, Altonji PF, Richter J, Deckers PJ. Regional deep hypothermia of the spinal cord protects against ischemic injury during thoracic aortic cross-clamping. Ann Thorac Surg 1994;57:65-70.
44. Cambria RP, Davison JK, Carter C, et al. Epidural cooling for spinal cord protection during thoracoabdominal aneurysm repair: a five-year experience. J Vasc Surg 2000;31:1093-102.
45. Shimizu H, Mori A, Yamada T, et al. Regional spinal cord cooling using a countercurrent closed-lumen epidural catheter. Ann Thorac Surg 2010;89:1312-3.
46. Lima B, Nowicki ER, Blackstone EH, et al. Spinal cord protective strategies during descending and thoracoabdominal aortic aneurysm repair in the modern era: the role of intrathecal papaverine. J Thorac Cardiovasc Surg 2012;143:945-52.e1.
47. Acher C, Wynn M. Paraplegia after thoracoabdominal aortic surgery: not just assisted circulation, hypothermic arrest, clamp and sew, or TEVAR. Ann Cardiothorac Surg 2012;1:365-72.
48. Ehrlich M, Knolle E, Ciovica R, et al. Memantine for prevention of spinal cord injury in a rabbit model. J Thorac Cardiovasc Surg 1999;117:285-91.
Cite This Article
How to Cite
Download Citation
Export Citation File:
Type of Import
Tips on Downloading Citation
Citation Manager File Format
Type of Import
Direct Import: When the Direct Import option is selected (the default state), a dialogue box will give you the option to Save or Open the downloaded citation data. Choosing Open will either launch your citation manager or give you a choice of applications with which to use the metadata. The Save option saves the file locally for later use.
Indirect Import: When the Indirect Import option is selected, the metadata is displayed and may be copied and pasted as needed.
About This Article
Special Issue
Copyright
Data & Comments
Data
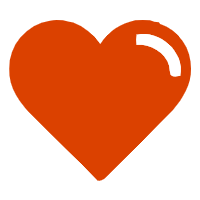
Comments
Comments must be written in English. Spam, offensive content, impersonation, and private information will not be permitted. If any comment is reported and identified as inappropriate content by OAE staff, the comment will be removed without notice. If you have any queries or need any help, please contact us at [email protected].