Omega-3 polyunsaturated fatty acids and cardiovascular health: a molecular view into structure and function
Abstract
Given the notorious impact of cardiovascular disease (CVD) as the current leading cause of mortality worldwide, the prevention, identification and management of CV risk factors represents a priority in daily clinical practice. Several studies have shown the beneficial effects of dietary omega-3 polyunsaturated fatty acids (PUFAs) on CV health. Their derivatives, eicosapentaenoic acid and docosahexaenoic acid, intervene in multiple metabolic pathways, including: regulation of the inflammatory response, by reducing the synthesis of pro-inflammatory cytokines; regulation of platelet aggregation, activation and adhesion, by modulating thromboxane A2 and plasminogen activator inhibitor-1 activity; regulation of the coagulation pathways, by reducing the carboxylation of vitamin K-dependent coagulation factors; improvement of endothelial function, given their effects on prostaglandin synthesis and endothelial nitric oxide synthase; reduction of serum lipids, through their effects on the hepatic synthesis of triacylglycerides, beta-oxidation of fatty acids and lipoprotein catabolism; and improvement of myocardial function via their membrane-stabilizing effects, and an increase in fluidity, size and distribution of membrane lipid rafts. Nevertheless, these effects appear to vary according to the type of PUFA ingested, dietary sources, daily dosing and individual factors inherent to the subject. Therefore, further studies are required to determine the ideal supplementation for each kind of patient and their particular CV profiles.
Keywords
Introduction
Cardiovascular disease (CVD) represents an ongoing global epidemic. In 2014, 27.6 million people were diagnosed with CVD worldwide,[1] and by 2030, it may be responsible for up to 23.5 million deaths yearly.[2] These trends are common in all westernized countries, including Latin America. In Venezuela, 29.47% of all mortality was attributed to CVD in 2012.[3] Given the heavy burden CVD represents for public health systems, prevention has become a key component in clinical practice and research, oriented to the identification and management of several risk factors, both modifiable and non-modifiable.[4] Regarding modifiable risk factors, westernized dietary patterns, notable for their high intake of dairy products, refined carbohydrates and saturated fats, have been strongly linked with the development of not only CVD, but also hypertension (HTN), obesity and type 2 diabetes mellitus.[5-7]
In 1980, Bang et al.[8] studied the diet of the Eskimo population of Greenland, characterized by high intake of foods rich in long chain polyunsaturated fatty acids (LC-PUFAs), and paradoxically found a low incidence of CVD in these individuals.[9] From these pioneer studies, different epidemiological and interventional investigations have backed the cardioprotective role of n-3 LC-PUFAs.[10,11]
Although many beneficial CV effects have been ascribed to PUFAs, including hypolipidemic, antithrombotic, antihypertensive and antiarrhythmic properties,[12,13] the underlying molecular mechanisms remain to be elucidated. This review aims to offer an integrated state-of-the-art vision into the structure of PUFAs and their functions in the CV system.
General overview of PUFAs
Structure and classification
Fatty acids are molecules consisting of a long linear hydrocarbon chain that generally contains a pair number of carbon atoms between 12 and 24, with a carboxyl (-COOH) group in one end and a methyl (-CH3) group in the other.[14] They are termed saturated fatty acids when only simple bonds exist between the carbon atoms, while those that have one or more double bonds are known as unsaturated fatty acids.[15] The latter include widely recognized nutritionally essential molecules for humans and other animal species, including linoleic acid (LA) and α-linoleic acid (ALA).[16]
Fatty acids with more than one double bond in their chain are called PUFAs, which are classified in 2 main subgroups: n-6 long chain PUFAs (n-6 LC-PUFAs) and n-3 long chain PUFAs (n-3 LC-PUFAs), which are commonly referred to as omega-6 and omega-3 PUFAs, respectively.[17] The former are LA derivatives with 2 double bonds, which are located 6 carbons away from the methyl end (18:2Ω6); whereas n-3 LC-PUFAs derive from ALA and have 3 double bonds, with the first one being in the third carbon of the chain (18:3Ω3)[18,19][Figure 1].
Figure 1. Metabolism of n-6 and n-3 polyunsaturated fatty acids. n-3 and n-6 polyunsaturated fatty acids are derived from linoleic acid (LA) and a-linoleic acid (ALA). Through various enzymatic reactions, LA is converted into arachidonic acid, responsible of the formation of mainly proinflammatory PG and TX. On the other hand, ALA is converted into EPA and DHA, which derive into mostly antiinflammatory PG and TX. PGE2: prostaglandin E2; PGD2: prostaglandin D2; PGF2a: prostaglandin F2a; PGI2: prostacyclin I2; PGE3: prostaglandin E3; PGI3: prostacyclin I3; TX: thromboxanes; LT: leukotrienes
Metabolism and general biologic functions of essential PUFAs
The metabolism of both types of PUFAs ends in the formation of eicosanoids, which are biologically active compounds including prostaglandins (PGs), thromboxanes (TXs) and leukotrienes (LTs).[18] As shown in Figure 1, arachidonic acid (AA) is synthetized from LA (n-6), and is converted by the action of cyclooxygenase (COX) and lipoxygenase (LOX) into 2-series PGs and TXs and 4-series LTs and lipoxines. Although these mediators intervene in both the establishment and resolution of the inflammatory response, their net effect is predominantly pro-inflammatory.[19,20] In contrast, ALA (n-3) is a precursor of eicosapentaenoic acid (EPA) and docosahexaenoic acid (DHA), from which 3-series PGs and TXs, as well as 5-series LTs, lipoxins, resolvins and neuroprotectins are derived. These compounds have chiefly anti-inflammatory effects,[17] which is why current nutritional guidelines are oriented towards an increase in n-3 PUFAs intake.[21-24] Furthermore, the products of both series mediate the regulation of other physiological processes, such as the maintenance of cell membrane architecture, especially through their arrangement in lipid rafts,[25,26] and play a role in hemostasis and vasoconstriction, which are further explained ahead.[18,27]
Role of PUFAs in cell membrane maintenance
The long hydrocarbon chains and double bonds in EPA and DHA exert modifications on the cell membrane due to their length and degree of unsaturation.[28] These molecules have been demonstrated to increase membrane fluidity and modify the size and distribution of lipid rafts in aortic endothelial cells and rat lymphocyte cultures.[29,30] Lipid rafts are dynamic membrane microdomains containing sterols, enriched sphingolipids and specific binding proteins, which attain a metastable resting state through a constellation of lipid-lipid, protein-lipid and protein-protein bonds.[31] Incorporation of n-3 PUFAs in lipid rafts results in decreased cholesterol and sphingolipids in these microdomains.[32] This has been confirmed by systematic studies in membrane models that suggest cholesterol to be incompatible with environments rich in highly unsaturated lipids, as observed in phospholipid bilayers containing DHA.[33]
Most of the research on n-3 PUFAs in membrane models has centered around DHA.[34] This molecule is deemed unique because it contains six double bonds and is very flexible, with quick rearrangements amidst multiple conformational states.[29] Spectrometry studies have revealed DHA-containing phospholipids to form their own domains with a different arrangement in presence of sphingolipids and cholesterol, excluding saturated acyl chains from their structure.[35] In addition, because n-3 PUFAs tend to reject cholesterol, DHA-containing phospholipids tend to create non-raft domains which may be physically separated on cell membranes. This allows proteins to more readily occupy a space according to its requirements in a specific domain or in amplified rafts.[36] An alternative model points out that n-3 PUFAs are probably incorporated in the rafts as nanodomains, forcing cholesterol out of rafts.[26] This model is also applicable to proteins within lipid rafts, where incorporation of n-3 PUFAs into lipid rafts forces proteins to relocate to non-raft domains.[26] Further research is required to fully understand the biologic importance and mechanisms underlying the lateral organization of lipid microdomains in cell membranes, as well as the modulatory effects of n-3 PUFAs in this context.[32]
Molecular mechanisms of PUFAs in cardiovascular health
Chronic pro-inflammatory states
The anti-inflammatory effects of n-3 PUFAs have been widely reported.[37-40] One of the central mechanisms is the down-regulation of the synthesis of pro-inflammatory cytokines such as tumor necrosis factor alpha, interleukin 6 and monocyte chemoattractant protein-1 (MCP-1)[41-44] in adipose tissue. This occurs when EPA and DHA bind to the G-protein coupled receptor (GPR120) in macrophages and adipocytes, causing its activation and internalization with β-arrestin-2, and forming the GPR120/β-arrestine-2 complex.[45] This complex is then dissociated into the transforming growth factor beta (TGF-β) activated kinase 1 binding protein 1 (TAB1) that results in the inhibition of TGF-β activated kinase 1 (TAK1), and thus the down-regulation of the nuclear factor kappa B (NF-ĸB) and the inhibition of its function.[44] Besides, the incorporation of DHA to the lipid membrane disrupts the signaling of toll-like receptor 4 (TLR-4) by impeding its translocation to the lipid raft, and inhibiting the signaling pathway of MD2/TRIAP-MyD88/IRAK-TRAF6/IKKβ[41,46,47][Figure 2]. Also, EPA and DHA cause the down-regulation of nicotinamide adenine dinucleotide phosphateoxidase, which induces the production of reactive oxygen species, a requirement for TLR-4 signaling.[41,42] These pathways converge in the inhibition of NF-ĸB, diminishing the inflammatory response.[44,45] In addition n-3 PUFAs may also prevent macrophage infiltration in adipose tissue.[41]
Figure 2. Role of polyunsaturated fatty acids in proinflammatory cytokine synthesis. EPA and DHA inhibit the production of proinflammatory cytokines through different mechanisms: (1) binding of EPA and DHA to the G protein-coupled receptor (GPR120) leads to its activation and binding to β arrestin-2, which then dissociates into TAB1 and inhibits TAK1, thus interrupting the IKKβ/NF-κB cascade; (2) the inclusion of EPA and DHA into the lipid bilayer, which modifies lipid rafts and interrupts the translocation of TLR-4 and the MD2/TRIAP-MyD88/IRAK-TRAF6/IKKβ/NF-κB pathway, thus inhibiting the production of cytokines, showing the antiinflammatory action of EPA and DHA
Thrombogenesis
The antithrombotic properties of PUFAs have been described since the 1980s, owing to the pioneer studies by Bang and Dyerberg.[48] These studies demonstrated that the Eskimo diet, characterized by high intake of seafood rich in n-3 PUFAs (mainly fish, seal and whale), was associated with a low incidence of CVD, as well as a decrease in thrombogenesis, evident by high incidence of hemorrhages.[49,50]
Even though these effects have been described in populations of different latitudes,[51-53] the inverse relation between n-3 PUFAs intake, platelet aggregation, coagulation and fibrinolysis is still not completely elucidated;[54] however, both in vitro and in vivo studies have reported that n-3 PUFAs supplementation reduces TXA2 synthesis, platelet activation and adhesion,[55] and decreases plasminogen activator inhibitor-1 (PAI-1) activity and concentration.[56]
The mechanisms by which n-3 PUFAs decrease thrombogenesis have been extensively studied, especially in platelets. High n-3 PUFA intake, especially EPA and DHA, appears to favor the replacement of AA in cell membrane phospholipids, decreasing the binding rate of AA to COX-1, resulting in reduced TXA2 synthesis, a vasoconstriction and platelet aggregation-promoting molecule.[57] On the other hand, this secondarily increases the production of TXA3, which exerts a significantly lower biological activity than TXA2.[58] Another mechanism observed with in vivo studies is the capacity of n-3 PUFAs to act as TXA2 and PG H2 antagonists, through the synthesis of protectin DX, a product of DHA dihydroxylation obtained by the action of LOX.[59] This compound also has the capacity to inhibit both COX-1 and COX-2 in platelets and neutrophils, significantly decreasing both platelet activation and aggregation [Figure 3].[60,61]
Figure 3. Role of polyunsaturated fatty acids in thrombogenesis. n-3 PUFAs exert their antithrombotic effect on platelets via two main processes: (1) replacement of arachidonic acid in the platelet membrane, which causes a decrease in the action of COX-1 on arachidonic acid, diminishing TXA2 synthesis and favoring the synthesis of TXA3; (2) activity as TXA2 antagonists through the synthesis of protectin DX, a molecule from the lipoxygenase pathway, which inhibitis COX-1 and COX-2. PUFA: polyunsaturated fatty acids; TXA2: thromboxane A2; TXA3: thromboxane A3; COX-1: cyclooxygenase 1; COX-2: cyclooxygenase 2
In contrast, views on the mechanisms underlying the anticoagulant effects of n-3 PUFAs remain controversial. Some studies suggest these molecules may interfere in the carboxylation of vitamin K-dependent coagulation factors II, VII, IX and X;[62,63] while other studies attribute more relevance to a modification in serum fibrinogen levels.[64] Similarly, the role of n-3 PUFAs in fibrinolysis remains unclear,[65] however it has been proposed that by unknown mechanisms, they alter PAI-1 synthesis through a genetic pathway.[66]
Dyslipidemia
The effects of n-3 PUFAs on serum lipids were also first ascertained by Bang and Dyerberg[67] in their emblematic study on the Eskimo population. Results of this study showed that individuals who stayed in their birthplace had lower levels of triacylglycerides (TAG), very low-density lipoproteins (VLDL-C) and low-density lipoproteins (LDL-C), whereas those who later migrated to Denmark showed a serum lipid profile similar to that of the Danish population. Thus, environmental factors - namely dietary n-3 PUFA intake - may exert a preponderant impact on serum lipids.[68]
Among these actions on lipid metabolism, the TAG-lowering effect has been found to be the most robust in large epidemiological studies;[69] however, it appears to be largely modifiable by the overall dietary composition, as elevated carbohydrate and saturated fat intake may surpass the effect of n-3 PUFAs due to increased TAG synthesis and storage.[69,70] In addition, the magnitude of the lipid-lowering effect of n-3 PUFAs depends on each subject’s basal serum lipid levels, as greater decreases are observed in subjects with higher TAG levels.[70,71]
The mechanisms by which n-3 PUFAs achieve these effects on TAG are related to the decrease of their hepatic synthesis via competitive inhibition of the enzymes involved, especially 1,2 diglyceride acyltransferase, which catalyzes the conversion of diacylglycerides into TAG.[72] In addition, PUFAs have high affinity for several peroxisome proliferator-activated receptor (PPAR) subtypes, particularly PPAR-α, a nuclear transcription factor highly expressed in adipose tissue and skeletal muscle.[73] When PPAR-α is activated by specific substrates like PUFAs, it favors the synthesis of enzymes involved in lipid catabolism.[74] Therefore, n-3 PUFA intake promotes the β-oxidation of fatty acids in peripheral tissues, which contributes to the catabolism of circulating TAG in chylomicrons and VLDL-C. This results in diminished traffic of non-esterified fatty acids to hepatocytes, causing an additional reduction in the input of substrates for TAG synthesis, further decreasing the hepatic production of VLDL-C.[72]
Additionally, PUFAs downregulate the sterol regulatory element-binding protein 1c (SREBP1c), which modulates the expression of genes involved in the synthesis of fatty acids and TAG.[75,76] PUFAs inhibit SREBP1c activity in the liver by antagonizing the liver X receptor alpha, a nuclear receptor found in hepatocytes that regulates the synthesis of SREBP and the SREBP inhibitor protein.[77,78] Another reported genetic mechanism is the ability of PUFAs to inhibit the hepatic maturation of the carbohydrate-responsive element-binding protein, a transcription factor related to the expression of enzymes involved in TAG synthesis[79][Figure 4].
Figure 4. Role of polyunsaturated fatty acids in triacylglyceride metabolism. PUFAs decrease TAG through various mechanisms: (1) competitive inhibition of DAT; (2) activating PPAR-α, which promotes the transcription of enzymes involved in lipolysis and fatty acid transport; (3) suppressing the activity of SREBP-1c which regulates the expression of genes involved in fatty acid and TAG synthesis. PUFA: polyunsaturated fatty acid; DAT: 1,2 diglyceride acyltransferase; TAG: triacylglycerides; PPAR-α: peroxisome-proliferator activated receptor alpha; RXR: retinoid X receptor; LXR-α: liver X receptor alpha; SREBP-1c: sterol regulatory element-binding protein 1c; SCAP: SREBP inhibitor protein; PPRE: PPAR response elements
These TAG-lowering mechanisms are accompanied by a secondary decrease of VLDL-C: in vitro studies have described PUFA-mediated activation of non-proteosomal degradation of apolipoprotein B (Apo B). This protein, found in the membrane of chylomicrons, LDL-C and VLDL-C, allows the transport of lipids towards peripheral tissues.[80] Activation of this pathway results in the selective degradation of the Apo B which would have been integrated into naïve VLDL, thus reducing liver secretion of this lipoprotein.[81,82]
Several randomized studies have shown that PUFAs also contribute to a slight increase of high density lipoprotein (HDL-C), ranging between 2.3-7.9% for EPA, and 2.9-18.3% for DHA.[83,84] PUFA-mediated reduction of cholesteryl ester transfer protein (CETP) activity may be accountable for this effect, as it would lead to a decrease in the net flow of cholesterol esters from HDL-C to LDL-C and VLDL-C. Recent in vitro studies also suggest PUFAs to be regulators of CETP and apolipoprotein A1 gene expression.[85,86]
On the other hand, the effects that PUFAs exert on LDL-C are yet to be defined. A study on adult women by Ooi et al.[87] where the effects of diets with high and low fish intake (1.23 g/day and 0.27 g/day of EPA and DHA, respectively) were assessed showed a non-significant decrease in serum LDL-C levels for both diets. However, significantly lower Apo-B100 concentration, greater LDL-C Apo-B100 production rate and higher conversion percentage of TAG-rich lipoproteins (TRL) into LDL-C were reported in high fish intake diets. Other studies have reported that EPA and DHA supplement intake considerably increases LDL-C levels when compared to placebo and EPA-exclusive intake.[88] However, recent evidence suggest that EPA as opposed to DHA has more prominent effects on LDL in patients with hyper-TG due to its antioxidant properties in various Apo B-containing proteins,[89] improving secondarily endothelial function and inflammatory profile.[90] Studies in animals suggest that very high intake of PUFAs of marine origin increases the union of the TRL to the endothelial LPL, prolonging the interaction period and thus resulting in a boost of LDL-C formation. Furthermore, very high intake of n-3 PUFAs has been found to boost the production of smaller TRL with less amount of Apo-E, which show a tendency to convert into LDL-C;[91] as well as increase the expression of hepatic LDL-C receptors, amplifying its catabolism.[92] Indeed, the effects of PUFAs on LDL-C levels appear to significantly vary across specific PUFA types and quantity.[88,89]
Hypertension
The role of HTN as one of the main independent risk factors for CVD has been widely recognized along with the role of n-3 PUFAs on its management.[7] However, recent findings have shown that maintaining normal blood pressure (BP) levels even in non-hypertensive individuals significantly lowers the incidence of CVD, representing important evidence for the use of n-3 PUFAs as a powerful preventive intervention.[93] A recent study by Huang et al.[94] on 1,154 Chinese adults found hypertensive subjects to have lower plasma PUFA concentrations when compared to healthy counterparts. This echoes the results of a previous study on 447 Eskimo people, where both high dietary intake and elevated plasma levels of n-3 PUFAs were associated with lower levels of diastolic blood pressure.[95]
PUFAs may regulate BP through various mechanisms, most powerfully through conversion into vasodilator PG and promotion of renin release from the kidney.[96] Moreover, it has been demonstrated that a diet rich in n3-PUFAs suppresses the activity of the angiotensin-converting enzyme, reduces the formation of angiotensin II, improves the generation of eNO (endothelial nitric oxide) and suppresses the expression of TGF-β.[97] Recently, in murine models with angiotensin II-dependent HTN, the combination of a soluble epoxide hydrolase inhibitor along with a diet rich in n3-PUFAs was tested, showing higher levels of EPA and DHA epoxides and a reduction of inflammatory markers in the kidney (PGs and MCP-1), contributing to a decrease in systolic BP and inflammation.[98]
Various cytochrome P450 (CYP) isoforms have also been identified in the physiological production of active metabolites of AA, EPA and DHA as alternative substrates.[99] In this context, Agbor et al.[100] demonstrated the contribution of isoform CYP1A1 tothe metabolism of n3-PUFAs, and the activation of endothelial nitric oxide synthase and consequent increase innitric oxide (NO) bioavailability associated with a diet rich in n3-PUFAs [Figure 5].
Figure 5. Role of polyunsaturated fatty acids in hypertension. n3-PUFAs intervene in blood regulation through the following pathways: (1) conversion into prostaglandins via the cyclooxygenase pathway, causing vasodilation of the smooth muscle in arterial walls; (2) inhibition of ACE, reducing the synthesis of AT-II, thus leading to a decrease in blood pressure; (3) promotion of cytochrome P450 isoforms such as CYP1A1, which contributes to the activation of eNOS, increasing the bioavailability of nitric oxide and thus causing vasodilation; (4) incorporation into the lipid matrix of the erythrocyte membrane, where they lead to a an increase, and a decrease in the sensitivity of arterial smooth muscle cells to vasoconstrictive effects. ACE: angiotensin-converting enzyme; AT-II: angiotensin II; eNOS: endothelial nitric oxide synthase; PUFA: polyunsaturated fatty acids
Furthermore, a study by Hoshi et al.[101] exposed the activation of large conductance Ca2+-activated K+ channels by DHA, through a fast and reversible stimulus independent of Ca2+ concentration. However, the exact bonding site remains to be identified. The activation of these channels in smooth muscle cells allows passive K+ efflux, which translates into the hyperpolarization of the cell membrane and thus its hypotensive effect.[102]
On the other hand, modifications in fatty acid composition in the lipid matrix of the cell membrane play an important role in the pathogenesis of hypertension.[103] A decrease of PUFAs in the cell membrane of erythrocytes leads to a decrease in the negative charge of the membrane, with reduced phospholipid fluidity, activation of the synthesis of proinflammatory eicosanoids, and increased sensitivity of arterial smooth muscle cells to vasoconstrictive effects.[103,104]
Myocardial function
Reports from different human and animal models have demonstrated that n-3 PUFAs improve left ventricular inotropic function, without causing hypertrophy or increase in blood pressure.[105] The underlying mechanism involves an increase in the activity of myosin ATPase and Na+/K+ ATPase, and the expression of Ca2+ ATPase in the sarcoplasmic reticulum, which are associated with positive inotropism, and maintenance of intra-sarcoplasmic reticulum calcium concentration and the sodium calcium exchanger (NCX).[106,107] Furthermore, an indirect effect is achieved through an increase in ventricular efficiency, which is defined as the production of the highest ejection volume with the lowest possible oxygen consumption, and the decrease in blood pressure.[108] This is possible due to the incorporation of DHA in the cell membrane,[109] influencing the eicosanoids mechanism and modulating cellular Ca2+ and its signaling pathways.[110] On the other hand, it has also been attributed to the shortening in the monophasic action potential due to the suppression of ATP-dependent K+ channels in the sarcolemma.[111]
Another proposed mechanism is the increase in the Na+/K+ ATPase activity, which boosts Na+ concentrations, diminishing the intracellular Ca2+ concentrations due to its effect in NCX activity in the cell membrane.[112] In addition, it has been demonstrated that the Na+/K+ ATPase activation modulates the function of L-type Ca2+ channels, which causes a greater release of calcium by the sarcoplasmic reticulum and higher intracellular Ca2+ gradients during systole, increasing contraction strength.[113]
Cardiac arrhythmia
Several studies have reported an association between n-3 PUFA intake and a lower risk of CVD-related death, specifically from ischemic events, where the myocardium is more prone to suffer irregularities in its electric activity that can lead to sudden death.[114,115]
Myocardial cells at the border of the ischemic zone have a relatively depolarized resting potential and can potentially generate ventricular fibrillation because of how easily they can be excited.[114] Because of this, an elevation in n-3 PUFAs stabilizes the high excitability of these partially depolarized cells in the ischemic myocardium. This prevents spontaneous or premature depolarization,[116] resulting in a longer refractory period and an increase in the voltage needed for the cellular depolarization.[117-120] More specifically, n-3 PUFAs can inhibit voltage-dependent Na+, K+ and Ca2+ channels, as well as Na+/Ca2+ exchangers and Ca2+-activated K+ channels.[121] Consequently, these changes lower membrane excitability,[122] translating to a net membrane-stabilizing effect.[116,123]
Finally, an antiarrhythmic mechanism has been implicated in the role that n-3 PUFAs play in autonomic control, by increasing the vagal tone.[124,125] Recent evidence highlights that the effect n-3 PUFAs exert on the electrophysiology of the ventricles and atria relies on their favorable action on cell-cell connections by modulating the expression and phosphorylation of connexin-43[125,126][Figure 6].
Figure 6. Antiarrhythmic effects of polyunsaturated fatty acids. n-3 PUFAs show several potential antiarrhythmic properties: (1) membrane potential stabilization by decreasing transmembrane io trafficn; (2) inhibition of the activity and expression of connexins, decreasing the conductivity of myocardial tissue; (3) increase in vagal tone, decreasing heart rate, conductivity and myocardial excitability. NCX: sodium-calcium exchanger; Cx43: connexin-43; PUFA: polyunsaturated fatty acids
Ischemia/reperfusion
Although the restoration of blood flow in the ischemic myocardium is essential for tissue survival during acute myocardial infarction, its reperfusion may directly accelerate the ischemic process or increase the myocardial injury in a phenomenon known as "reperfusion injury".[127-129] Important studies have reported that this event is responsible for up to 50% of the final infarction size.[130] PUFAs appear to be associated with reduced ischemic/reperfusion injury and thus with a better recovery after a coronary event.[131]
During ischemia/reperfusion, increased n-3 PUFA content in the mitochondrial membrane may contribute to stabilization and thus lower myocardial oxygen consumption (MVO2), thereby attenuating the thermodynamic inefficiency caused by hypoxia.[132,133] In addition, a lower MVO2 could diminish vulnerability to arrhythmia through the energetic maintenance of transmembrane potentials during episodes of ischemia.[133]
A study on 211 patients with ST segment elevation myocardial infarction who underwent reperfusion by percutaneous coronary intervention found patients with higher levels of n-3 PUFAs (EPA + DHA ≥ 155 mg/mL) had a lower incidence of reperfusion injury than those with lower levels of n-3 PUFAs (EPA + DHA < 155 mg/mL).[134] Although the antiarrhythmic effect may exert the most potent impact in ischemia/reperfusion injury,[135] other supplementary actions may also intervene, including antithrombotic, anti-inflammatory and vasoactive effects.[136-138]
Conclusion
As has been described in review, n-3 PUFAs boast several beneficial effects in CV physiology and pathophysiology [Figure 7]. Notwithstanding current available evidence supporting the administration of n-3 PUFAs as a therapeutic intervention in CVD, further research is required to better characterize the underlying molecular mechanisms, as well as refine recommendations for their clinical use. Indeed, one of the most pressing issues is the assessment of potential adverse effects linked to the therapeutic implementation of n3-PUFAs, along with the determination of adequate dosing and sources for these molecules in a myriad of specific clinical scenarios.
Figure 7. Role of polyunsaturated fatty acids in cardiovascular function. The actions of n3-PUFAs are diverse, including the decrease of the inflammatory response via NF-kB inhibition, as well as an increase of β-oxidation, causing the catabolism of triaclyglycerides and contributing to the decrease of lipids stored both in the liver and vessel walls. In addition, by increasing the production of TXA3 in vessel walls, PUFAs decrease vascular resistance, reducing blood pressure. On the other hand, one of the most described effects of n3-PUFAs is their action on cardiac arrhythmia, by inhibiting voltage-gated ion channels and exchangers, as well as increasing the vagal tone of the atria and ventricles, which leads to a lower heart rate. PUFA: polyunsaturated fatty acids; TXA2: thromboxane A2; TXA3: thromboxane A3; COX-1: cyclooxygenase 1; DAT: 1,2 diglyceride acyltransferase; TAG: triacylglycerides; NF-kB: nuclear factor kappa B; TLR-4: toll-like receptor 4; VLDL: very low-density lipoproteins
Authors’ contributions
Article conception and design: M.J. Calvo, M.S. Martínez
Acquisition and analysis of bibliographic information: M.J. Calvo, M.S. Martínez, W. Torres, M. Chávez-Castillo, E. Luzardo, N. Villasmil
Drafting of the manuscript: M.J. Calvo, M.S. Martínez, W. Torres, M. Chávez-Castillo, E. Luzardo, N. Villasmil, J. Salazar
Critical revision and final approval: M. Velasco, V. Bermúdez
Financial support and sponsorship
None.
Conflicts of interest
There are no conflicts of interest.
Patient consent
Not applicable.
Ethics approval
Not applicable.
REFERENCES
1. Center for Disease Control and Prevention. Summary Health Statistics: National Health Interview Survey, 2014. Avalaible from: https://ftp.cdc.gov/pub/Health_Statistics/NCHS/NHIS/SHS/2014_SHS_Table_A-1.pdf. [Last accessed on 29 Jun 2017].
2. World Health Organization. Global status report on non-communicable disease. 2010. Available from: http://whqlibdoc.who.int/publications/2011/9789240686458_eng.pdf. [Last accessed on 29 Jun 2017].
3. Anuario de Mortalidad 2013. Ministerio del Poder Popular para la Salud de la República Bolivariana de Venezuela. Available from: http://www.ovsalud.org/descargas/publicaciones/documentos-oficiales/Anuario-Mortalidad-2013.pdf. [Last accessed on 30 Jun 2017].
4. American Heart Association & American Stroke Association. Cardiovascular disease: a costly burden for America. Projections through 2035. Available from: https://www.heart.org/idc/groups/heart-public/@wcm/@adv/documents/downloadable/ucm_491543.pdf. [Last accessed on 29 Jun 2017].
5. Sweazea KL. Compounding evidence implicating Western diets in the development of metabolic syndrome. Acta Physiol 2014;211:471-3.
6. Heinonen I, Petteri R, Ruohonen ST, Ruohonen S, Ahotupa M, Savontaus E. The effects of equal caloric high fat and western diet on metabolic syndrome, oxidative stress, and vascular endothelial function in mice. Acta Physiol 2014;211:515-27.
8. Bang H, Dyerberg J, Sinclair H. The composition of the Eskimo food in north western Greenland. Am J Clin Nutri 1980;23:2657-61.
9. Bjerregaard P. Disease pattern in Greenland: studies on morbidity in Upernavik 1979-1980 and mortality in Greenland 1968-1985. Arctic Med Res 1991;50 Suppl 4:1-62.
10. Kromhout D, Bosschieter E, de Lezenne Coulander C. The inverse relation between fish consumption and 20-year mortality from coronary disease. N Engl J Med 1985;312:1205-9.
11. Kris-Etherton P, Eckel RH, Howard BV, St Jeor S, Bazzarre TL; Nutrition Committee Population Science Committee and Clinical Science Committee of the American Heart Association. AHA Science Advisory: Lyon Diet Heart Study. Benefits of a Mediterranean-style, National Cholesterol Education Program/American Heart Association Step I Dietary Pattern on Cardiovascular Disease. Circulation 2001;103:1823-5.
12. Maehre HK, Jensen IJ, Elvevoll EO, Eilertsen KE. ω-3 fatty acids and cardiovascular diseases: effects, mechanisms and dietary relevance. Int J Mol Sci 2015;16:22636-61.
13. Torres N, Guevara-Cruz M, Velazquez-Villegas LA, Tovar AR. Nutrition and atherosclerosis. Arch Med Res 2015;46:408-26.
14. Abozid MM, Ayimba E. Effect of omega 3 fatty acids family in human health. Int J Adv Res 2014;2:202-11.
16. Graham C, Burdge C, Philip C. Conversion of α-linolenic acid to longer-chain polyunsaturated fatty acids in human adults. Reprod Nutr Dev 2005;45:581-97.
17. Cicconel MM, Scicchitano P, Gesualdo M, Zito A, Carbonara S, Ricci G, Cortese F, Giordano P. The role of omega-3 polyunsatured fatty acid supplementation in childhood: a review. Recent Pat Cardiovasc Drug Discov 2013;8:42-55.
19. Patterson E, Wall R, Fitzgerald G, Ross R, Stanton C. Health implications of high dietary omega-6 polyunsaturated fatty acids. J Nutr Metab 2012;2012:539426.
20. Frolov A, Yang L, Dong H, Hammock BD, Crofford LJ. Anti-inflammatory properties of prostaglandin E2: deletion of microsomal prostaglandin E synthase-1 exacerbates non-inmune inflammatory arthritis in mice. Prostaglandins Leukot Essent Fatty Acids 2013;89:351-8.
21. Piepoli MF, Hoes AW, Agewall S, Albus C, Brotons C, Catapano AL, Cooney MT, Corrà U, Cosyns B, Deaton C, Graham I, Hall MS, Hobbs FD, Løchen ML, Löllgen H, Marques-Vidal P, Perk J, Prescott E, Redon J, Richter DJ, Sattar N, Smulders Y, Tiberi M, van der Worp HB, van Dis I, Verschuren WM; Authors/Task Force Members. 2016 European Guidelines on cardiovascular disease prevention in clinical practice: The Sixth Joint Task Force of the European Society of Cardiology and Other Societies on Cardiovascular Disease Prevention in Clinical Practice (constituted by representatives of 10 societies and by invited experts) developed with the special contribution of the European Association for Cardiovascular Prevention & Rehabilitation (EACPR). Eur Heart J 2016;37:2315-81.
22. Luis D, Zlatkis K, Comenge B, García Z, Navarro JF, Lorenzo V, Carrero JJ. Dietary quality and adherence to dietary recommendations in patients undergoing hemodialysis. J Ren Nutr 2016;26:190-5.
23. Dawson D, Branch-Mays G, Gonzalez O, Ebersole J. Dietary modulation of the inflammatory cascade. Periodontol 2014;64:161-97.
24. Panel on Dietetic Products EFSA, Nutrition, and Allergies (NDA) NDA. Scientific opinion on dietary reference values for fats, including saturated fatty acids, polyunsaturated fatty acids, monounsaturated fatty acids, trans fatty acids, and cholesterol. EFSA J 2010;8:1461.
25. Turk H, Chapkin R. Membrane lipid raft organization is uniquely modified by n-3 polyunsaturated fatty acids. Prostaglandins Leukot Essent Fatty Acids 2013;88:43-7.
26. Shaikh S. Biophysical and biochemical mechanisms by which dietary n-3 polyunsaturated fatty acids from fish oil disrupt membrane lipid rafts. J Nutr Biochem 2012;23:101-5.
27. Zanetti M, Grillo A, Losurdo P, Panizon E, Mearelli F, Cattin L, Barazzoni R, Carretta R. Omega-3 polyunsaturated fatty acids: structural and functional effects on the vascular wall. Biomed Res Int 2015;2015:791978.
28. Mozaffarian D, Wu JH. (n-3) fatty acids and cardiovascular health: are effects of EPA and DHA shared or complementary? J Nutr 2012;142:S614-25.
29. Hussein JS. Cell membrane fatty acids in health. Int J Phram Pharm Sci 2013;5:38-46.
30. Hashimoto M, Hossain MS, Yamasaki H, Yazawa K, Masumura S. Effects of eicosapentaenoic acid and docosahexaenoic acid on plasma membrane fluidity of aortic endothelial cells. Lipids 1999;34:1297-304.
31. Owen DM, Magenau A, Williamson D, Gaus K. The lipid raft hypothesis revisited -- new insights on raft composition and function from super-resolution fluorescence microscopy. Bioessays 2012;34:739-47.
32. Ma DW, Seo J, Switzer KC, Fan YY, McMurray DN, Lupton JR JR, Chapkin RS. n-3 PUFA and membrane microdomains: a new frontier in bioactive lipid research. J Nutr Biochem 2004;15:700-6.
33. Jaureguiberry MS, Tricerri MA, Sanchez SA, Finarelli GS, Montanaro MA, Prieto ED, Rimoldi OJ. Role of plasma membrane lipid composition on cellular homeostasis: learning from cell line models expressing fatty acid desaturases. Acta Biochim Biophys Sin (Shanghai) 2014;46:273-82.
34. Hou TY, McMurray DN, Chapkin RS. Omega-3 fatty acids, lipid rafts, and T cell signaling. Eur J Pharmacol 2016;785:2-9.
35. Williams JA, Batten SE, Harris M, Rockett BD, Shaikh SR, Stillwell W, Wassall SR. Docosahexaenoic and eicosapentaenoic acids segregate differently between raft and nonraft domains. Biophys J 2012;103:228-37.
36. Levental KR, Lorent JH, Lin X, Skinkle AD, Surma MA, Stockenbojer EA, Gorfe AA, Levental I. Polyunsaturated lipids regulate membrane domain stability by tuning membrane order. Biophys J 2016;110:1800-10.
37. Cicero AF, Rosticci M, Morbini M, Cagnati M, Grandi E, Parini A, Borghi C. Lipid-lowering and anti-inflammatory effects of omega 3 ethyl esters and krill oil: a randomized, cross-over, clinical trial. Arch Med Sci 2016;12:507-12.
38. Raphael W, Sordillo LM. Dietary polyunsaturated fatty acids and inflammation: the role of phospholipid byosynthesis. Int J Mol Sci 2013;14:21167-88.
39. Tortosa-Caparros E, Navas-Carrillo, Marin F, Orenes-Pi-ero E. Anti-inflammatory effects of omega 3 and omega 3 polyunsaturated fatty acids in cardiovascular disease and metabolic syndrome. Crit Rev Food Sci Nutr 2017;57:3421-9.
40. Calder PC. Omega-3 polyunsaturated fatty acids and inflammatory processes: nutrition or pharmacology? Br J Clin Pharmacol 2013;75:645-62.
41. Puglisi MJ, Hasty AH, Saraswathi V. The role of adipose tissue in mediating the beneficial effects of dietary fish oil. J Nutr Biochem 2011;22:101-8.
42. Nakahira K, Kim HP, Geng XH, Nakao A, Wang X, Murase N, Borghi C. Carbon monoxide differentially inhibits TLR signaling pathways by regulating ROS-induced trafficking of TLRs to lipid rafts. J Exp Med 2006;203:2377-89.
43. Rockett BD, Salameh M, Carraway K, Morrison K, Shaikh SR. n-3 PUFA improves fatty acid composition, prevents palmitate-induced apoptosis, and differentially modifies B cell cytokine secretion in vitro and ex vivo. J Lipid Res 2010;51:1284-97.
44. Oh DY, Talukdar S, Bae EJ, Imamura T, Morinaga H, Fan W, Li P, Lu WJ, Watkins SM, Olefsky JM. GPR120 is an omega-3 fatty acid receptor mediating potent anti-inflammatory and insulin-sensitizing effects. Cell 2010;142:687-98.
45. Oh DY, Walenta E. The role of omega-3 fatty acid receptor GPR120 in insulin resistance. Int J Obes Suppl 2014;4:S14-6.
47. Stulnig TM, Huber J, Leitinger N, Imre EM, Angelisova P, Nowotny P, Waldhausl W. Polyunsaturated eicosapentaenoic acid displaces proteins from membrane rafts by altering raft lipid composition. J Biol Chem 2001;276:37335-40.
48. Bang HO, Dyerberg J. Lipid metabolism and ischemic heart disease in Greenland Eskimos. In: Draper HH, editor. Advances in Nutritional Research. New York, NY: Plenum Press; 1980. pp. 1-22.
49. Dyerberg J, Bang HO. Haemostatic function and platelet polyunsaturated fatty acids in Eskimos. Lancet 1979;2:433-5.
51. Larson MK, Tormoen GW, Weaver LJ, Luepke KJ, Patel IA, Hjelmen CE. Exogenous modification of platelet membranes with the omega-3 fatty acids EPA and DHA reduces platelet procoagulant activity and thrombus formation. Am J Physiol Cell Physiol 2013;304:C273-9.
52. von Schacky C, Siess W, Fischer S, Weber PC. A comparative study of eicosapentaenoic acid metabolism by human platelets in vivo and in vitro. J Lipid Res 1985;26:457-64.
53. Hirai A, Hamazaki T, Terano T, Nishikawa T, Tamura Y, Kamugai A, Jajiki J. Eicosapentaenoic acid and platelet function in Japanese. Lancet 1980;2:1132-3.
54. Mohebi-Nejad A, Bikdeli B. Omega-3 supplements and cardiovascular diseases. Tanaffos 2014;13:6-14.
55. Lagarde M, Calzada C, Guichardant M, Vericel E. In vitro and in vivo bimodal effects of docosahexaenoic acid supplements on redox status and platelet function. Prostaglandins Leukot Essent Fatty Acids 2016;108:1-4.
56. Phang M, Lincz LF, Garg ML. Eicosapentaenoic and docosahexaenoic acid supplementations reduce platelet aggregation and hemostatic markers differentially in men and women. J Nutr 2013;143:457-63.
57. Walker CG, West AL, Browning LM, Madden J, Gambell JM, Jebb SA, Calder PC. The pattern of fatty acids displaced by EPA and DHA following 12 months supplementation varies between blood cell and plasma fractions. Nutrients 2015;7:6281-93.
58. Ayala J, Lopez C, Azueg H, Oberto C, Paiva A, Lares M. Effects of polyunsaturated fatty acids (omega-3) on the platelet aggregation. Rev Latinoam Hiperte 2009;4:71-8. (in Portuguese)
59. Parent CA, Lagarde M, Venton DL, Le Breton GC. Selective modulation of the human platelet thromboxane A2/prostaglandin H2 receptor by eicosapentaenoic and docosahexaenoic acids in intact platelets and solubilized platelet membranes. J Biol Chem 1992;267:6541-7.
60. Liu M, Boussetta T, Makni-Maalej K, Fay M, Driss F, El-Benna J, Lagarde M, Guichardant M. Protectin DX, a double lipoxygenase product of DHA, inhibits both ROS production in human neutrophils and cyclooxygenase activities. Lipids 2014;49:49-57.
61. Lagarde M, Calzada C, Guichardant M, Véricel E. Dose-effect and metabolism of docosahexaenoic acid. Pathophysiological relevance in blood platelets. Prostaglandins Leukot Essent Fatty Acids 2013;88:49-52.
62. Leray C, Wiesel ML, Freund M, Cazenave JP, Gachet C. Long-chain n-3 fatty acids specifically affect rat coagulation factors dependent on vitamin K. Arterioscler Thromb Vasc Biol 2001;21:459-65.
63. Vanschoonbeek K, Wouters K, van der Meijden PE, van Gorp PJ, Feijge MA, Herfs M, Schurgers LJ, Hofker MH, de Maat MP, Heemskerk JW. Anticoagulant effect of dietary fish oil in hyperlipidemia: a study of hepatic gene expression in APOE2 knock-in mice. Arterioscler Thromb Vasc Biol 2008;28:2023-9.
64. Vanschoonbeek K, Feijge MA, Paquay M, Rosing J, Saris W, Kluft C, Giesen PL, de Maat MP, Heemskerk JW. Variable hypocoagulant effect of fish oil intake in humans: modulation of fibrinogen level and thrombin generation. Arterioscler Thromb Vasc Biol 2004;24:1734-40.
65. Finnegan YE, Howarth D, Minihane AM, Kew S, Miller GJ, Calder PC, Williams CM. Plant and marine derived (n-3) polyunsaturated fatty acids do not affect blood coagulation and fibrinolytic factors in moderately hyperlipidemic humans. J Nutr 2003;133:2210-3.
66. Montegaard C, Tulk HM, Lauritzen L, Tholstrup T, Robinson LE. Acute ingestion of long-chain (n-3) polyunsaturated fatty acids decreases fibrinolysis in men with metabolic syndrome. J Nutr 2010;140:38-43.
67. Bang HO, Dyerberg J. Plasma lipids and lipoproteins in Greenlandic west coast Eskimos. Acta Med Scand 1972;192:85-94.
68. Dyerberg J, Bang HO, Hjorne N. Plasma cholesterol concentration in Caucasian Danes and Greenland west coast Eskimos. Dan Med Bull 1977;24:52-5.
69. Balk EM, Lichtenstein AH, Chung M, Kupelnick B, Chew P, Lau J. Effects of omega-3 fatty acids on serum markers of cardiovascular disease risk: a systematic review. Atherosclerosis 2006;189:19-30.
70. Balk E, Chung M, Lichtenstein A, Chew P, Kupelnick B, Lawrence A, DeVine D, Lau J. Effects of omega-3 fatty acids on cardiovascular risk factors and intermediate markers of cardiovascular disease. Evid Rep Technol Assess (Summ) 2004;(93):1-6.
71. Kelley DS, Siegel D, Vemuri M, Mackey BE. Docosahexaenoic acid supplementation improves fasting and postprandial lipid profiles in hypertriglyceridemic men. Am J Clin Nutr 2007;86:324-33.
72. Harris WS, Bulchandani D. Why do omega3 fatty acids lower serum triglycerids? Curr Opin Lipidol 2006;17:387-93.
73. Tillander V, Bjørndal B, Burri L, Bohov P, Skorve J, Berge RK, Alexson SE. Fish oil and krill oil supplementations differentially regulate lipid catabolic and synthetic pathways in mice. Nutr Metab (Lond) 2014;11:20.
74. Walczak R, Tontonoz P. PPARadigms and PPARadoxes: expanding rolesfor PPARg in the control of lipid metabolism. J Lipid Res 2002;43:177-86.
76. Landa V, Zídek V, Mlejnek P, Šimáková M, Šilhavý J, Trnovská J, Kazdová L, Pravenec M. Sterol regulatory element binding protein 2 overexpression is associated with reduced adipogenesis and ectopic fat accumulation in transgenic spontaneously hypertensive rats. Physiol Res 2014;63:587-90.
77. Yang T, Espenshade PJ, Wright ME, Yabe D, Gong Y, Aebersold R, Goldstein JL, Brown MS. Crucial step in cholesterol homeostasis: sterols promote binding of SCAP to INSIG-1, a membrane protein that facilitates retention of SREBPs in ER. Cell 2002;110:489-500.
78. Watanabe M, Uesugi M. Small-molecule inhibitors of SREBP activation - potential for new treatment of metabolic disorders. Med Chem Comm 2013;4:1422-33.
79. Dentin R, Benhamed F, Pégorier JP, Foufelle F, Viollet B, Vaulont S, Girard J, Postic C. Polyunsaturated fatty acids suppress glycolytic and lipogenic genes through the inhibition of ChREBP nuclear protein translocation. J Clin Invest 2005;115:2843-54.
80. Packard CJ, Demant T, Stewart JP, Bedford D, Caslake MJ, Schwertfeger G, Bedynek A, Shepherd J, Seidel D. Apolipoprotein B metabolism and the distribution of VLDL and LDL subfractions. J Lipid Res 2000;41:305-18.
81. Fisher EA, Pan M, Chen X, Wu X, Wang H, Jamil H, Sparks JD, Williams KJ. The triple threat to nascent apolipoprotein B. Evidence for multiple, distinct degradative pathways. J Biol Chem 2001;276:27855-63.
82. Pan M, Cederbaum AI, Zhang YL, Ginsberg HN, Williams KJ, Fisher EA. Lipid peroxidation and oxidant stress regulate hepatic apolipoprotein B degradation and VLDL production. J Clin Invest 2004;113:1277-87.
83. Jacobson TA, Glickstein SB, Rowe JD, Soni PN. Effects of eicosapentaenoic acid and docosahexaenoic acid on low-density lipoprotein cholesterol and other lipids: a review. J Clin Lipidol 2012;6:5-18.
84. Maggi F, Sirtori CR, Baldassarre D, Rise P, Galli C. Correlations of n-3 (omega-3) fatty acid levels with lipoproteins: negative correlation with HDL-C after an oral load. Nutrafods 2013;12:13-6.
85. Raposo HF, Patrício PR, Simões MC, Oliveira HC. Fibrates and fish oil, but not corn oil, up-regulate the expression of the cholesteryl ester transfer protein (CETP) gene. J Nutr Biochem 2014;25:669-74.
86. Chan D, Watts GF, Nguyen MN, Barrett PH. Factorial study of the effect of n-3 fatty acid supplementation and atorvastatin on the kinetics of HDL apolipoproteins A-I and A-II in men with abdominal obesity. Am J Clin Nutr 2006;84:37-43.
87. Ooi E, Lichtenstein A, Millar J, Diffenderfer M, Lamon-Fava S, Rasmussen H, Welty FK, Barrett PH, Schaefer EJ. Effects of therapeutic lifestyle change diets high and low in dietary fish-derived FAs on lipoprotein metabolism in middle-aged and elderly subjects. J Lipid Res 2012;53:1958-67.
88. Fialkow J. Omega-3 fatty acid formulations in cardiovascular disease: dietary supplements are not substitutes for prescription products. Am J Cardiovasc Drugs 2016;16:229-39.
89. Mason R, Sherratt S, Jacob R. Eicosapentaenoic acid inhibits oxidation of ApoB-containing lipoprotein particles of different size in vitro when administered alone or in combination with atorvastatin active metabolite compared with other triglyceride-lowering agents. J Cardiovasc Pharmacol 2016;68:33-40.
90. Borow K, Nelson J, Mason R. Biologic plausibility, cellular effects, and molecular mechanisms of eicosapentaenoic acid (EPA) in atherosclerosis. Atherosclerosis 2015;242:357-66.
91. Yamada N, Shames DM, Stoudemire JB, Havel RJ. Metabolism of lipoproteins containing apolipoprotein B-100 in blood plasma of rabbits: heterogeneity related to the presence of apolipoprotein E. Proc Natl Acad Sci U S A 1986;83:3479-83.
92. Fernandez ML, McNamara DJ. Dietary fat-mediated changes in hepatic apoprotein B/E receptor in the guinea pig: effect of polyunsaturated, monounsaturated, and saturated fat. Metabolism 1989;38:1094-102.
93. McInnes GT. Lowering blood pressure for cardiovascular risk reduction. J Hypertens Suppl 2005;23:S3-8.
94. Huang T, Shou T, Cai N, Wahlqvist ML, Li D. Associations of plasma n-3 polyunsaturated fatty acids with blood pressure and cardiovascular risk factors among Chinese. Int J Food Sci Nutr 2012;63:667-73.
95. Ebbesson SO, Risica PM, Ebbesson LO, Kennish JM, Tejero ME. Omega-3 fatty acids improve glucose tolerance and components of the metabolic syndrome in Alaskan Eskimos: the Alaska Siberia project. Int J Circumpolar Health 2005;64:396-408.
96. Rasmussen BM, Vessby B, Uusitupa M, Berglund L, Pedersen E, Riccardi G, Rivellese AA, Tapsell L, Hermansen K; KANWU Study Group. Effects of dietary saturated, monounsaturated, and n-3 fatty acids on blood pressure in healthy subjects. Am J Clin Nutr 2006;83:221-6.
98. Ulu A, Harris TR, Morisseau C, Miyabe C, Inoue H, Schuster G, Dong H, Iosif AM, Liu J, Weiss RH, Chiamvimonvat N, Imig JD, Hammock BD. Anti-inflammatory effects of omega-3 polyunsaturated fatty acids and soluble epoxide hydrolase inhibitors in angiotensin-II dependent hypertension. J Cardiovasc Pharmacol 2013;62:285.
99. Shao Z, Fu Z, Stahl A, Joyal JS, Hatton C, Juan A, Hurst C, Evans L, Cui Z, Pei D, Gong Y, Xu D, Tian K, Bogardus H, Edin ML, Lih F, Sapieha P, Chen J, Panigrahy D, Hellstrom A, Zeldin DC, Smith LE. Cytochrome P450 2C8 ω3-long-chain polyunsaturated fatty acid metabolites increase mouse retinal pathologic neovascularization--brief report. Arterioscler Thromb Vasc Biol 2014;34:581-6.
100. Agbor LN, Wiest EF, Rothe M, Schunck WH, Walker MK. Role of CYP1A1 in modulating the vascular and blood pressure benefits of omega-3 polyunsaturated fatty acids. J Pharmacol Exp Ther 2014;351:688-98.
101. Hoshi T, Wissuwa B, Tian Y, Tajima N, Xu R, Bauer M, Heinemann SH, Hou S. Omega-3 fatty acids lower blood pressure by directly activating large-conductance Ca2+-dependent K+ channels. Proc Natl Acad Sci U S A 2013;110:4816-21.
102. Wakle-Prabagaran M, Lorca RA, Ma X, Stamnes SJ, Amazu C, Hsiao JJ, Karch CM, Hyrc KL, Wright ME, England SK. BKCa channel regulates calcium oscillations induced by alpha-2-macroglobulin in human myometrial smooth muscle cells. Proc Natl Acad Sci U S A 2016;113:2335-44.
103. Novgorodtseva TP, Karaman YK, Gvozdenko TA, Antonyuk MV, Knyshova VV. Modification of fatty acids composition in erythrocytes lipids in arterial hypertension associated with dyslipidemia. Health 2013;5:73-7.
104. Klein-Platat C, Drai J, Oujaa M, Schlienger JL, Simon C. Plasma fatty acid composition is associated with the metabolic syndrome and low-grade inflammation in overweight adolescents. Am J Clin Nutr 2005;82:1178-84.
105. Nodari S, Triggiani M, Campia U, Manerba A, Milesi G, Cesana BM. Effects of n-3 polyunsaturated fatty acids on left ventricular function and functional capacity in patients with dilated cardiomyopathy. J Am Coll Cardiol 2011;57:870-9.
106. del Monte F, Harding SE, Schmidt U, Matsui T, Kang ZB, Dec GW, Gwathmey JK, Rosenzweig A, Hajjar RJ. Restoration of contractile function in isolated cardiomyocytes from failing human hearts by gene transfer of SERCA2a. Circulation 1999;100:2308-11.
107. Ribeiro Junior RF, Fernandes AA, Meira EF, Batista PR, Siman FD, Vassallo DV, Padilha AS, Stefanon I. Soybean oil increases SERCA2a expression and left ventricular contractility in rats without change in arterial blood pressure. Lipids Health Dis 2010;9:53.
108. Liu J, Conklin S, Manuck S, Yao J, Muldoon M. Long-chain omega-3 fatty acids and blood pressure. Am J Hypertens 2011;24:1121-6.
109. McLennan P. Myocardial membrane fatty acids and the antiarrhythmic actions of dietary fish oil in animal models. Lipids 2001;36:S111-4.
110. Mori T, Bao D, Burke V, Puddey I, Beilin J. Docosahexaenoic acid but not eicosapentaenoic acid lowers ambulatory blood pressure and heart rate in humans. Hypertension 1999;34:253-60.
111. Tsuburaya R, Yasuda S, Ito Y, Shiroto T, Gao J, Ito K, Shimokawa H. Eicosapentaenoic acid reduces ischemic ventricular fibrillation via altering monophasic action potential in pigs. J Mol Cell Cardiol 2011;51:329-36.
112. Schwinger RH, Bundgaard H, Muller-Ehmsen J, Kjeldsen K. The Na+, K+- ATPase in the failing human heart. Cardiovasc Res 2003;57:913-20.
113. Lee DI, Klein MG, Zhu W, Xiao RP, Gerzanich V, Xu KY. Activation of (Na+- K+)-ATPase modulates cardiac L-type Ca2+ channel function. Mol Pharmacol 2009;75:774-81.
114. Leaf A, Kang J, Xiao Y, Billman G. Clinical prevention of sudden cardiac death by n-3 polyunsaturated fatty acids and mechanism of prevention of arrhythmias by n-3 fish oils. Circulation 2003;107:2646-52.
115. Kromhout D, Yasuda S, Geleijnse J, Shimokawa H. Fish oil and omega 3 fatty acids in cardiovascular disease: do they really work? Eur Heart J 2012;33:436-43.
116. Xiao Y, Sigg D, Ujhelyi M, Wilhelm J, Richardson E, Iaizzo P. Pericardial delivery of omega-3 fatty acid: a novel approach to reducing myocardial infarct sizes and arrhythmias. Am J Physiol Heart Circ Physiol 2008;294:2212-8.
117. Xiao YF, Sigg DC, Leaf A. The antiarrhythmic effect of n-3 polyunsaturated fatty acids: modulation of cardiac ion channels as a potential mechanism. J Membr Biol 2005;206:141-54.
118. Endo J, Arita M. Cardioprotective mechanism of omega-3 polyunsaturated fatty acids. J Cardiol 2016;67:22-7.
119. Xiao Y, Ma L, Wang S, Josephson M, Wang G, Morgan J, Leaf A. Potent block of inactivation deficient Na+ channels by n-3 polyunsaturated fatty acids. Am J Physiol Cell Physiol 2006;290:362-70.
120. Li G, Sun H, Zhang X, Cheng L, Chiu S, Tse H, Lau CP. Omega-3 polyunsaturated fatty acids inhibit transient outward and ultra-rapid delayed rectifier K+ currents and Na+ current in human atrial myocytes. Cardiovasc Res 2009;81:286-93.
121. Moreno C, á Macías, á Prieto, de la Cruz A, González T, Valenzuela C. Effects of n-3 polyunsaturated fatty acids on cardiac ion channels. Front Physiol 2012;3:245.
122. Kang JX, Xiao YF, Leaf A. Free, long-chain, polyunsaturated fatty acids reduce membrane electrical excitability in neonatal rat cardiac myocytes. Proc Natl Acad Sci U S A 1995;92:3997-4001.
123. Pi-eiro-Corrales G, Rivero N, Culebras F. Role of omega-3 fatty acids in cardiovascular disease prevention. Nutr Hosp 2013;28:1-5.
124. Marai I, Massalha S. Effect of omega-3 polyunsaturated fatty acids and vitamin d on cardiovascular diseases. Isr Med Assoc J 2014;16:117-21.
125. Christensen J, Schmidt E. Autonomic nervous system, heart rate variability and n-3 fatty acids. J Cardiovasc Med (Hagerstown) 2007;8:S19-22.
126. Dlugosova K, Okruhlicova L, Mitasikova M, Sotnikova R, Bernatova I, Weismann P, Slezak J, Tribulova N. Modulation of connexin-43 by omega-3 fatty acids in the aorta of old spontaneously hypertensive rats. J Physiol Pharmacol 2009;60:63-9.
127. Fischer R, Dechend R, Qadri F, Markovic M, Feldt S. Dietary n-3 polyunsaturated fatty acids and direct renin inhibition improve electrical remodeling in a model of high human renin hypertension. Hypertension 2008;51:540-6.
129. Hausenloy DJ, Duchen MR, Yellon DM. Inhibiting mitochondrial permeability transition pore opening at reperfusion protects against ischemia-reperfusion injury. Cardiovasc Res 2003;60:617-25.
130. Bei R, Frigiola A, Masuelli L, Marzocchella L, Tresoldi I, Modesti A, Galvano F. Effects of omega-3 polyunsaturated fatty acids on cardiac myocyte protection. Front Biosci (Landmark Ed) 2011;16:1833-43.
131. Pepe S, Tsuchiya N, Lakatta EG, Hansford RG. PUFA and aging modulate cardiac mitochondrial membrane lipid composition and Ca2_ activation of PDH. Am J Physiol 1999;276:149-58.
132. Pepe S, McLennan P. Cardiac membrane fatty acid composition modulates myocardial oxygen consumption and postischemic recovery of contractile function. Circulation 2002;105:2303-8.
133. Abdukeyum G, Owen A, Larkin T, McLennan P. Up-regulation of mitochondrial antioxidant superoxide dismutase underpins persistent cardiac nutritional-preconditioning by long chain n-3 polyunsaturated fatty acdis in the rat. J Clin Med 2016;5:E32.
134. Arakawa K, Himeno H, Kirigaya J, Otomo F, Matsushita K, Nakahashi H, Shimizu S, Nitta M, Yano H, Endo M, Kimura K, Umemura S. Impact of n-3 polyunsaturated fatty acids in predicting ischemia/reperfusion injury and progression of myocardial damage after reperfusion in patients with ST-segment elevation acute myocardial infarction. J Cardiol 2015;66:101-7.
135. Hausenloy D, Yellon D. Myocardial ischemia-reperfusion injury: a neglected therapeutic target. J Clin Invest 2013;123:92-100.
136. Iba-ez B, Heusch G, Ovize M, Van de Werf F. Evolving therapies for myocardial ischemia/reperfusion injury. J Am Coll Cardiol 2015;65:1454-71.
137. Neri M, Riezzo I, Pascale N, Pomara C, Turillazzi E. Ischemia/reperfusion injury following acute myocardial infarction: a critical issue for clinicians and forensic pathologists. Mediators Inflamm 2017;2017:7018393.
138. Farías JG, Carrasco-Pozo C, Carrasco Loza R, Sepúlveda N, álvarez P, Quezada M, Qui-ones J, Molina V, Castillo RL. Polyunsaturated fatty acid induces cardioprotection against ischemia-reperfusion through the inhibition of NF-kappaB and induction of Nrf2. Exp Biol Med (Maywood) 2017;242:1104-14.
Cite This Article

How to Cite
Download Citation
Export Citation File:
Type of Import
Tips on Downloading Citation
Citation Manager File Format
Type of Import
Direct Import: When the Direct Import option is selected (the default state), a dialogue box will give you the option to Save or Open the downloaded citation data. Choosing Open will either launch your citation manager or give you a choice of applications with which to use the metadata. The Save option saves the file locally for later use.
Indirect Import: When the Indirect Import option is selected, the metadata is displayed and may be copied and pasted as needed.
About This Article
Copyright
Author Biographies
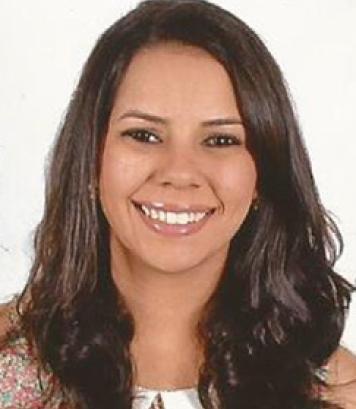
Comments
Comments must be written in English. Spam, offensive content, impersonation, and private information will not be permitted. If any comment is reported and identified as inappropriate content by OAE staff, the comment will be removed without notice. If you have any queries or need any help, please contact us at [email protected].