Platelet aerobic metabolism: new perspectives
Abstract
Although the role of platelets in hemostasis and thrombotic disorders as well as their contribution to inflammation are known, recent studies support the notion that much remains to be learned about platelet bioenergetics. Recent data suggest that platelets possess extra-mitochondrial oxidative phosphorylation (OXPHOS), which could represent one of sources of the chemical energy necessary for the prompt platelets activation. However, the extra-mitochondrial OXPHOS can play both beneficial and pathological roles, since the OXPHOS is the principal responsible of oxidative stress generation. For this reason, several authors evaluated the effects of polyphenols and other antioxidants on the modulation of the platelets oxidative stress production. In conclusion, we believe that a better understanding of platelet oxidative metabolism would allow a deeper knowledge of their physiology and the designing novel treatments targeting the role of platelets in many human diseases.
Keywords
Platelet formation and functions
Platelets are anucleated cell fragments arising from bone marrow megakaryocytes (MK), playing an important role in hemostasis, inflammation and wound repair[1]. MK develop from stem cells, differentiating to MK progenitors. Immature MK, during the third phase of development, undergo polyploidization (> 2N DNA) and cytoplasmic enlargement. A network of specialized membranes forms within the MK cytoplasm, termed demarcation membranes system, forming proplatelets, long extensions with branching ends[2,3]. The fourth developmental stage is thrombocytopoiesis: platelet release, promoted by shear forces[2,3]. Mature platelet lifetime in the blood is about 7 days.
Platelets are “first responders” promptly sensing endothelial damage initiating clotting[1], but they can also react to circulating pathogens[4]. Platelet remarkable reactivity justifies their involvement in coagulative dysfunctions and thrombotic disorders[5]. Recent evidence supports the notion that platelets can also play a pivotal role in cancer progression, promoting tumorigenesis through factor secretion and metastasis to form immune-evasive aggregates with tumour cells[6]. Platelet dysfunction appears involved in complications of type II diabetes mellitus (DM): hyperglycemia would increase platelet mitochondrial ATP content[7], but it was also shown that platelet oxidative phosphorylation (OXPHOS) was impaired in type II DM[8]. Mitochondrial complex I activity was found to be decreased in aged platelets[9].
Platelet bioenergetics
The rapid response rate of platelet activation involves dramatic shape changes, i.e., loss of discoid form, dynamic filopodia formation[6]. This phenomenon is significantly increased in stenosed arteries in vivo, consistent with the role of platelets in vascular disease[10]. Platelets, being anucleate, possess a stable metabolic program over their lifetime to meet their physiological energetic demand and expenditure during their activation. The integrated bioenergetic machinery involves both glycolysis and OXPHOS[11,12]. While at a basal state both aerobic metabolism and glycolysis play a role in energy production in platelets production, OXPHOS is required for agonist-stimulated platelet activation[12-14]. One study reported that platelets rely on OXPHOS also in basal conditions[15]. Authors studied the basal oligomycin-sensitive mitochondrial oxygen consumption rate (OCR) and glycolytic flux (ECAR), showing that human platelets from healthy donors have a low ECAR value in face of the highest basal OCR one with respect to nucleate blood cells, meaning that platelets are the most metabolically active figurative elements of the circulating blood. Accordingly, the mitochondrial platelet functioning is remarkable and it has been largely studied[13-15], since platelets are considered a valuable biomarker of mitochondrial pathologies[5,8,16].
New perspectives on oxidative metabolism in platelets
Although platelets metabolism is sustained by the OXPHOS, it seems that there is a mismatch among the platelet elevated aerobic metabolism and their mitochondria number[14,17]. We have recently shown, by TEM microscopy, that two is the average mitochondria number of platelets and several of them being devoid of mitochondria[18]. Notably, as far as the maturation process is involved, the number of platelets released per MK ranges from 2000-11,000 platelet per MK[2]. This implies that, even though the MK cytoplasm organizes itself forming dense bodies, secretory vesicles so that vital organelles are found within the nascent platelets, the mature MK should contain some 8000 mitochondria on the average, to guarantee that at least one mitochondrion resides in each platelet, which does not seem to be the case. Therefore, mitochondria may not be sufficient to produce the large amount of energy required for platelet rapid activation involving cytoskeletal rearrangements, and sudden shape changes[19], suggesting that other structures may be involved in the aerobic energy generation.
We have recently shown that platelets display a consistent oxygen consumption and ATP production in the presence of NADH, a respiring substrate not suitable for mitochondria in vitro. This NADH-stimulated oxygen consumption appeared sensitive to thrombin or collagen and insensitive to atractyloside, an inhibitor of Adenine nucleotide translocase[18], suggesting that other structures are involved in platelets respiration besides mitochondria. Moreover, in platelets, the ratio of the expression of F1Fo-ATP synthase and the translocase of the inner membrane (TIM; a mitochondrial inner membrane protein not involved in the OXPHOS) was higher than in mitochondria[18]. Since, in mitochondria, the proportion of these two proteins is conserved, this was considered a clue to the fact that ATP synthase could be also expressed in the internal platelet membranes. In other words, our study suggested the existence of an extramitochondrial energy production in platelets[18][Figure 1]. This hypothesis is confirmed by the confocal microscopy analysis, which shows a low signal of TIM in the face of a strong colocalization between COXII or β subunit of ATP synthase with calnexin, a marker of the endoplasmic reticulum (ER).
Figure 1. Scheme of metabolic flux in platelets. The scheme describes the energy production in platelets, carried out by the extramitochondrial structures. Glucose is converted in pyruvate by the glycolysis pathway with the production of 2 molecules of ATP and NADH. Since, in our model, the proton gradient is formed at the center of the membrane, the NADH produced by glycolysis could transfer its electrons and protons directly to complex I, triggering the extramitochondrial respiration and the relative ATP synthesis on both sides of the membrane. Moreover, also the hypothetical extramitochondrial Krebs cycle could represent a source of reduced coenzymes for the ectopic respiration. CI: complex I; C II: complex II; C III: complex III; C IV: complex IV; e-: electron; H+: proton; a: a subunit of Fo moiety; OAA: oxalacetic acid; αKG: α-ketoglutarate
As far as the extramitochondrial respiration is concerned, the main difference respect to mitochondrial one is the absence of a double membrane system, supposedly necessary to accumulate the proton gradient, according to Mitchell’s theory. However, in two of our previous papers, a proton delivery at the center of a membrane expressing the electron transfer chains has been proposed[20,21]. In particular, this model supposed that the proton movement inside the membranes occurs as a moving charge, according to the proton-hopping Grotthuss mechanism, with the establishment of “protonic currents” inside the membrane. These would allow the ATP synthesis on both sides of the membrane, as schematized in Figure 1. Therefore, based on this model, we can speculate that the NADH produced by glycolysis, could be used directly by the respiratory Complex I present on the platelet membranous structures, triggering the extramitochondrial OXPHOS. Moreover, considering that the expression of the cytosolic form of Krebs enzymes is well described, we can also speculate that an extramitochondrial Krebs cycle in platelets could represent another source of reduced coenzymes for the ectopic OXPHOS. On the other hand, we have demonstrated the functional expression of an extramitochondrial Krebs cycle both in disks and in myelin vesicles[22,23].
We have also reported the functional ectopic expression of the OXPHOS machinery in the rod outer segments and the myelin sheath[22,24-26]. However, a number of reports showed the expression of the five redox complexes in other systems in many membranous structures devoid of mitochondria (reviewed in Ref.[27]), some of which were found biochemically active[28-30]. On the other hand, it is reasonable believe that a mechanism as the extramitochondrial aerobic metabolism may not be exclusive of the nervous system, but exploited when a considerable amount of ATP is needed, such as in cancer cells[28,31]. Moreover, the exportability of mitochondrial proteins is suggested by the strictly interaction and membrane trafficking between mitochondria and the other cellular membranous structure, i.e., ER[32].
Potential sources of oxidative stress in platelets
Oxidative stress is the consequence of an unbalance among the intracellular overproduction of reactive oxygen species (ROS) and the intracellular antioxidant response[33]. Oxidative stress is considered a common mechanism underlying many human diseases[34]. An important source of ROS in platelets is NADPH oxidase[35], however, the main source of ROS is the electron transport chain (ETC)[36]. The existence of an extra-mitochondrial ETC in platelets is suggestive of an unsuspected source of ROS production outside the mitochondrion[37,38]. Oxidative stress plays both beneficial and pathological roles in platelets, acting both in the regulation of platelet responses and in the pathological process of athero-thrombosis[39,40] and platelet apoptosis[5]. This dual[37,38] role of ROS in platelets would justify the ectopic expression of the ETC itself. In fact, an extra-mitochondrial ROS production would avoid the damage to mitochondrial membranes consequent to ROS production, potentially dangerous, as these contain mitochondrial damage-associated molecular patterns, which can activate inflammation.
Antioxidants
Multiple lines of evidence suggest that the addition of exogenous antioxidants to the diet plays a role in the prevention of oxidative stress[41]. In particular, polyphenols, Vitamins E, A and C or n-3 PUFA appear to bear a potential interest. In the last years, several antioxidant molecules have been tested as potential inhibitors of platelets aggregation, but only some of these showed a strong positive effect [Table 1].
Effect of antioxidant molecules and food extracts on platelets aggregation
Antioxidant molecule | Concentration | % aggregation inhibition | Note | Ref. |
---|---|---|---|---|
Resveratrol | 0.075 mM | 22% | a | [43] |
0.15 mM | 55% | a | [43] | |
0.3 mM | 54% | a | [43] | |
0.6 mM | 70% | a | [43] | |
Trolox | 0.53 mM | 32% | a | [43] |
1.05 mM | 35% | a | [43] | |
2.1 mM | 52% | a | [43] | |
4.2 mM | 55% | a | [43] | |
Mixed tocopherols | 100 mg γ-, 40 mg δ-, 20 mg α-tocopherol | 14% | b | [44] |
α-tocopherol | 100 mg | no inhibition | c | [44] |
DHPE | 0.1 mM | 55% | c | [45] |
Oleuropein | 0.1 mM | 11.50% | c | [45] |
Luteolin | 0.1 mM | 23.30% | c | [45] |
Apigenin | 0.1 mM | no inhibition | c | [45] |
Quercetin | 0.1 mM | no inhibition | c | [45] |
NDGA | 0.1 mM | 52% | c | [45] |
GSH | 1 mM | 55% | d | [46] |
3 mM | 69% | d | [46] | |
10 mM | 100% | d | [46] | |
Caffein | 2 mM | 33% | e | [47] |
EGCG | 0.2 mg/mL | 100% | e | [47] |
Green tea extract | 1 mg/mL | 100% | e | [47] |
Yuzu extract | 1 mg/mL (methanol fraction) | 80% | c | [48] |
1 mg/mL (ethyl acetate fraction) | 100% | c | [48] | |
1 mg/mL (hexane fraction) | 78% | c | [48] | |
Hallabong extract | 1 mg/mL (methanol fraction) | 40% | c | [48] |
1 mg/mL (ethyl acetate fraction) | 95% | c | [48] | |
1 mg/mL (hexane fraction) | 35% | c | [48] | |
Orange extract | 1 mg/mL (methanol fraction) | 42% | c | [48] |
1 mg/mL (ethyl acetate fraction) | 93% | c | [48] | |
1 mg/mL (hexane fraction) | 18% | c | [48] | |
Walnut hull extract | 25 µg/mL | 28% | f | [49] |
50 µg/mL | 45% | f | [49] | |
100 µg/mL | 63% | f | [49] | |
200 µg/mL | 79% | f | [49] |
Notably, mixes of antioxidant molecules extracted from the food seem to be more active in the inhibition of platelets aggregation with respect the single antioxidant molecule. However, the proper choice of the antioxidant should pay attention to its ability to cross multiple membranes and to avoid an excess of reduced molecules, since an excess of reducing equivalents causes reductive stress, which displays a strong negative effect on the cellular homeostasis[42].
Conclusion
In conclusion, taking into consideration the possibility that platelets may be privileged sites of extra-mitochondrial aerobic metabolism could allow us to better understand their physio-pathology. The reliance of platelet on aerobic metabolism in face of a limited number of small mitochondria, the developed membrane system inside the platelets and the above considerations raise the hypothesis that the high oxygen consumption and the consequent free radical production can be ascribed to the ectopic OXPHOS. A deeper knowledge of the platelet bioenergetics would allow designing new treatments targeting the pivotal role of platelets in many human diseases, developing in vitro strategies to manufacture platelet products for both adult and paediatric patients, and choosing the best antioxidant therapy. We have recently shown that polyphenols have the ability to hamper the production of free radicals modulating the activity of the extra-mitochondrial respiratory chains binding the ectopic ATP synthase[38]. Future studies on the role of polyphenols supplementation on platelet aggregation in humans may benefit from this novel and challenging perspective.
Declarations
Authors’ contributionsManuscript Conceptualization: Ravera S, Panfoli I
Original draft preparation writing: Panfoli I
Writing review and editing: Ravera S, Panfoli I
Availability of data and materialsNot applicable.
Financial support and sponsorshipNone.
Conflicts of interestAll authors declared that there are no conflicts of interest.
Ethical approval and consent to participateNot applicable.
Consent for publicationNot applicable.
Copyright© The Author(s) 2019.
REFERENCES
1. Jenne CN, Urrutia R, Kubes P. Platelets: bridging hemostasis, inflammation, and immunity. Int J Lab Hematol 2013;35:254-261.
2. Reems JA, Pineault N, Sun S. In Vitro Megakaryocyte Production and Platelet Biogenesis: State of the Art. Transfus Med Rev 2010;24:33-43.
3. Italiano JE, Lecine P, Shivdasani RA, Hartwig JH. Blood platelets are assembled principally at the ends of proplatelet processes produced by differentiated megakaryocytes. J Cell Biol 1999;147:1299-312.
4. Menter DG, Kopetz S, Hawk E, Sood AK, Loree JM, et al. Platelet “first responders” in wound response, cancer, and metastasis. Cancer Metastasis Rev 2017;36:199-213.
5. Zharikov S, Shiva S. Platelet mitochondrial function: from regulation of thrombosis to biomarker of disease. Biochem Soc Trans 2013;41:118-23.
6. Kanikarla-Marie P, Lam M, Sorokin AV, Overman MJ, Kopetz S, et al. Platelet Metabolism and Other Targeted Drugs; Potential Impact on Immunotherapy. Front Oncol 2018;8:107.
7. Guo X, Wu J, Du J, Ran J, Xu J. Platelets of type 2 diabetic patients are characterized by high ATP content and low mitochondrial membrane potential. Platelets 2009;20:588-93.
8. Avila C, Huang RJ, Stevens MV, Aponte AM, Tripodi D, et al. Platelet mitochondrial dysfunction is evident in type 2 diabetes in association with modifications of mitochondrial anti-oxidant stress proteins. Exp Clin Endocrinol Diabetes 2012;120:248-51.
9. Lenaz G, Bovina C, Castelluccio C, Fato R, Formiggini G, et al. Mitochondrial Complex I defects in aging. Mol Cell Biochem 1997;174:329-33.
10. Ajzenberg N, Haghighat Talab AT, Massé JM, Drouin A, Jondeau K, et al. Platelet shape change and subsequent glycoprotein redistribution in human stenosed arteries. Platelets 2005;16:13-8.
11. Verhoeven AJ, Mommersteeg ME, Akkerman JW. Quantification of energy consumption in platelets during thrombin-induced aggregation and secretion. Tight coupling between platelet responses and the increment in energy consumption. Biochem J 1984;221:777-87.
12. Verhoeven AJ, Mommersteeg ME, Akkerman JW. Comparative studies on the energetics of platelet responses induced by different agonists. Biochem J 1986;236:879-87.
13. Leoncini G, Maresca M, Colao C. Oxidative metabolism of human platelets. Biochem Int 1991;25:647-55.
14. Sjövall F, Ehinger JKH, Marelsson SE, Morota S, Frostner EA, et al. Mitochondrial respiration in human viable platelets--methodology and influence of gender, age and storage. Mitochondrion 2013;13:7-14.
15. Baracca A, Barogi S, Carelli V, Lenaz G, Solaini G. Catalytic activities of mitochondrial ATP synthase in patients with mitochondrial DNA T8993G mutation in the ATPase 6 gene encoding subunit a. J Biol Chem 2000;275:4177-82.
16. Kramer PA, Ravi S, Chacko B, Johnson MS, Darley-Usmar VM. A review of the mitochondrial and glycolytic metabolism in human platelets and leukocytes: implications for their use as bioenergetic biomarkers. Redox Biol 2014;2:206-10.
17. Garcia-Souza LF, Oliveira MF. Mitochondria: biological roles in platelet physiology and pathology. Int J Biochem Cell Biol 2014;50:156-60.
18. Ravera S, Signorello MG, Bartolucci M, Ferrando S, Manni L, et al. Extramitochondrial energy production in platelets. Biol Cell 2018;110:97-108.
19. Li Z, Delaney MK, O’Brien KA, Du X. Signaling During Platelet Adhesion and Activation. Arterioscler Thromb Vasc Biol 2010;30:2341-9.
20. Morelli AM, Ravera S, Calzia D, Panfoli I. Hypothesis of lipid-phase-continuity proton transfer for aerobic ATP synthesis. J Cereb Blood Flow Metab 2013;33:1838-42.
21. Morelli A, Ravera S, Calzia D, Panfoli I. An update of the chemiosmotic theory as suggested by possible proton currents inside the coupling membrane. Open Biol 2019;9:180221.
22. Panfoli I, Calzia D, Ravera S, Bruschi M, Tacchetti C, et al. Extramitochondrial tricarboxylic acid cycle in retinal rod outer segments. Biochimie 2011;93:1565-75.
23. Ravera S, Bartolucci M, Calzia D, Aluigi MG, Ramoino P, et al. Tricarboxylic acid cycle-sustained oxidative phosphorylation in isolated myelin vesicles. Biochimie 2013;95:1991-8.
24. Ravera S, Panfoli I, Calzia D, Aluigi MG, Bianchini P, et al. Evidence for aerobic ATP synthesis in isolated myelin vesicles. Int J Biochem Cell Biol 2009;41:1581-91.
25. Ravera S, Aluigi MG, Calzia D, Ramoino P, Morelli A, et al. Evidence for ectopic aerobic ATP production on C6 glioma cell plasma membrane. Cell Mol Neurobiol 2011;31:313-21.
26. Ravera S, Nobbio L, Visigalli D, Bartolucci M, Calzia D, et al. Oxydative phosphorylation in sciatic nerve myelin and its impairment in a model of dysmyelinating peripheral neuropathy. J Neurochem 2013;126:82-92.
27. Panfoli I, Ravera S, Bruschi M, Candiano G, Morelli A. Proteomics unravels the exportability of mitochondrial respiratory chains. Expert Rev Proteomics 2011;8:231-9.
28. Chang HY, Huang HC, Huang TC, Yang PC, Wang YC, et al. Ectopic ATP synthase blockade suppresses lung adenocarcinoma growth by activating the unfolded protein response. Cancer Res 2012;72:4696-706.
29. Arakaki N, Kita T, Shibata H, Higuti T. Cell-surface H+-ATP synthase as a potential molecular target for anti-obesity drugs. FEBS Lett 2007;581:3405-9.
30. Mangiullo R, Gnoni A, Leone A, Gnoni GV, Papa S, et al. Structural and functional characterization of F(o)F(1)-ATP synthase on the extracellular surface of rat hepatocytes. Biochim Biophys Acta 2008;1777:1326-35.
31. Cossu V, Marini C, Piccioli P, Rocchi A, Bruno S, et al. Obligatory role of endoplasmic reticulum in brain FDG uptake. Eur J Nucl Med Mol Imaging 2019;46:1184-96.
32. Marchi S, Patergnani S, Pinton P. The endoplasmic reticulum-mitochondria connection: one touch, multiple functions. Biochim Biophys Acta 2014;1837:461-9.
33. Pham-Huy LA, He H, Pham-Huy C. Free radicals, antioxidants in disease and health. Int J Biomed Sci 2008;4:89-96.
36. Genova ML, Baracca A, Biondi A, Casalena G, Faccioli M, et al. Is supercomplex organization of the respiratory chain required for optimal electron transfer activity? Biochim Biophys Acta 2008;1777:740-6.
37. Ravera S, Bartolucci M, Cuccarolo P, Litamè E, Illarcio M, et al. Oxidative stress in myelin sheath: The other face of the extramitochondrial oxidative phosphorylation ability. Free Radic Res 2015;49:1-36.
38. Calzia D, Degan P, Caicci F, Bruschi M, Manni L, et al. Modulation of the rod outer segment aerobic metabolism diminishes the production of radicals due to light absorption. Free Radic Biol Med 2019;136:183.
39. Bartimoccia S, Nocella C, Pastori D, Pignatelli P, Carnevale R. Platelet Oxidative Stress and Antioxidant Nutrients. J Vasc Med Surg 2014;02:1-4.
41. Scalbert A, Manach C, Morand C, Rémésy C, Jiménez L. Dietary polyphenols and the prevention of diseases. Crit Rev Food Sci Nutr 2005;45:287-306.
42. Pérez-Torres I, Guarner-Lans V, Rubio-Ruiz ME. Reductive Stress in Inflammation-Associated Diseases and the Pro-Oxidant Effect of Antioxidant Agents. Int J Mol Sci 2017;18:E2098.
43. Sobotková A, Másová-Chrastinová L, Suttnar J, Stikarová J, Májek P, et al. Antioxidants change platelet responses to various stimulating events. Free Radic Biol Med 2009;47:1707-14.
44. Liu M, Wallmon A, Olsson-Mortlock C, Wallin R, Saldeen T. Mixed tocopherols inhibit platelet aggregation in humans: potential mechanisms. Am J Clin Nutr 2003;77:700-6.
45. Petroni A, Blasevich M, Salami M, Papini N, Montedoro GF, et al. Inhibition of platelet aggregation and eicosanoid production by phenolic components of olive oil. Thromb Res 1995;78:151-60.
46. Pacchiarini L, Tua A, Grignani G. In vitro effect of reduced glutathione on platelet function. Haematologica 1996;81:497-502.
47. Sagesaka-Mitane Y, Miwa M, Okada S. Platelet aggregation inhibitors in hot water extract of green tea. Chem Pharm Bull (Tokyo) 1990;38:790-3.
48. Assefa AD, Ko EY, Moon SH, Keum YS. Antioxidant and antiplatelet activities of flavonoid-rich fractions of three citrus fruits from Korea. 3 Biotech 2016;6:109.
Cite This Article
How to Cite
Download Citation
Export Citation File:
Type of Import
Tips on Downloading Citation
Citation Manager File Format
Type of Import
Direct Import: When the Direct Import option is selected (the default state), a dialogue box will give you the option to Save or Open the downloaded citation data. Choosing Open will either launch your citation manager or give you a choice of applications with which to use the metadata. The Save option saves the file locally for later use.
Indirect Import: When the Indirect Import option is selected, the metadata is displayed and may be copied and pasted as needed.
About This Article
Special Issue
Copyright
Data & Comments
Data
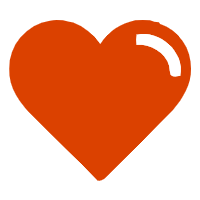
Comments
Comments must be written in English. Spam, offensive content, impersonation, and private information will not be permitted. If any comment is reported and identified as inappropriate content by OAE staff, the comment will be removed without notice. If you have any queries or need any help, please contact us at [email protected].