Bionic limb replacement: an evolving concept in lower extremity reconstruction
Abstract
Limb loss is disabling and carries significant functional and psychological repercussions to both the individual and society. The numbers of amputees are forecasted to double by 2050 from vascular disease and diabetes alone. Europe has 4.66 million amputees (431,000 amputations per year) and the United States 2 million amputees (185,000 amputations per year). Microvascular expertise is now more commonplace, increasing the likelihood of limb salvage and replantation. Further reconstructive input can take advantage of nerve and tendon grafting/transfers, free tissue transfer, and complex bone reconstruction. When this strategy does not satisfy individual needs, such as that seen with unstable soft tissues, amputation may be requested or offered. In part, the decision for salvage, replantation, or amputation in the future is likely to be guided by the sophistication of limb substitutes. This review will introduce the growing domain of bionics and where research in this area may deliver a sought clinical need.
Keywords
INTRODUCTION
Major lower extremity trauma presents itself as one of the most complex, challenging, and multi-disciplinary areas in the field of reconstructive surgery. This demands dedicated expertise to manage both the acute presentation and rehabilitative process. Outcomes are causally related to the magnitude and level of injury, patient condition, and available expertise. Depression, anxiety, substance abuse, and suicidal ideation can be significant months after injury, emphasising the importance of delivering a holistic approach[1].
Further reconstructive surgery using autologous tissues (nerve, muscle, soft tissue flaps, bone) provide a platform to replace and rebuild missing parts when available. When the desired outcome is unachievable, amputation with or without the incorporation of prostheses remains an acceptable treatment option. A healed and anatomically well-shaped residuum is crucial if not compromised by obesity, diabetes, cardiovascular disease and phantom limb pain (PLP)[2,3]. Corrective surgery at the residuum to address PLP (affects the quality of life in 65%-80% of lower limb amputees[4]), complex regional pain syndrome, bone spurs and delayed healing equally prohibit the use of prostheses[5] increasing the rate of revision[6]. A proportion of lower limb amputees with the aid of a prosthesis return to work (50%). Few are able enough to return to their premorbid occupations [3 in 9 below-knee amputees (BKA)[7]]. During ambulation, mean oxygen consumption rises (9% increase after BKA, 49% unilateral above knee and 280% bilateral above knee)[8]. This review briefly addresses the limitations that can be encountered using the conventional reconstructive armamentarium. Competing technologies continue to evolve, and bionic solutions may offer widespread functional benefit as a limb replacement strategy in the absence or presence of severely compromised tissues [Figure 1].
LOWER LIMB RECONSTRUCTION
Complex lower limb injury after open fracture results in considerable loss of function and is primarily accounted for by road traffic accidents in 65% of cases[9-11]. The last two decades have seen significant advances in surgical approach and service-led management, such that limbs previously deemed non-salvageable forego amputation. In spite of this, after salvage, infection, thrombosis, impaired bone healing, and revision surgery are not uncommon[12]. The Lower Extremity Assessment Project[11] found that the likelihood of amputation increases in the presence of complex fractures, muscle injury, vascular injury and large skin defects with mass contamination and absent plantar sensation[13]. It has been argued that limb salvage outcomes are not dissimilar from amputation, but formal comparisons do not always address the wider complexities after lower limb injury[14].
The current reconstructive armamentarium often satisfies the common goal of limb length preservation, adequate stable soft tissue cover for weight bearing and sensory recovery[15]. Recognising the zone of injury can be challenging, and the failure rate of fasciocutaneous and muscle flaps are reportedly 8%-9%[16,17]. Limb shortening after segmental tissue loss can lead to a discrepancy that, when significant, gives rise to unfavourable function[18]. Bone transport/distraction osteogenesis often require prolonged recovery and rehabilitation, and in some instances, patients request a voluntary amputation[19,20]. Isolated bone defects can be addressed by grafts (vascularised/non-vascularised)[21-24] or more advanced techniques
TRANSPLANTATION
The most achievable yet realistic strategy to replace “like with like” is transplantation. Vascularised composite allotransplantation (VCA) enables the transfer of multiple tissues (skin, muscle, bone, nerve, vessel). The enthusiasm for lower limb transplantation has yet to mirror that of the upper extremity. This is despite United States estimates suggesting the pool of lower limb amputees exceeds the upper limb by three times[27]. Though rare, lower limb transplantation has witnessed active knee flexion, extension, ankle motion and sensory recovery.
It can be argued, amongst other reasons, that there are two caveats to performing VCA on a routine basis. Firstly, cell death and necrosis after procurement and reperfusion impact donor health and the allograft. Secondly, VCA is not life-saving, and the need for life-long immunosuppression increases the risk of cancer, infection, major causes of post-transplant morbidity and mortality[28-30]. Moreover, the prospect of revision amputation of the lower limb must be carefully considered given one recipient ceased immunosuppression after being diagnosed with lymphoma[31]. Establishing which patients advance best after salvage/replantation, modern-day prostheses, or VCA is challenging[32]. Given the low number of VCA, randomised clinical trials providing a meaningful comparison between alternative strategies is yet to exist.
MODERN-DAY PROSTHESES AND STATE OF THE ART
Functional outcomes from modern-day lower limb prostheses can be excellent[15,33] but must be able to withstand considerable forces transmitted via the residuum[34]. The goal is a healed residuum with prosthetic fit that is painless and provides acceptable function[35]. Socket fit is influenced by trauma, oedema, atrophy, body habitus and changing temperature and is further complicated by pressure sores, blisters, infection and pistoning[36,37]. Bone anchored prostheses were designed to tackle poor prosthetic fit and address many complicating factors. The theoretical advantage is that bypassing soft tissues and transmitting force to skeletal components reduces energy expenditure and preserves the health of residuum. This has been met with a further improvement in gait efficiency, quality of life, range of motion and prosthetic uptake[38,39]. Movement at the knee is controlled by mechanical (passive, impedance controlled by friction/hydraulics) or microprocessor-controlled devices (impedance adjusted by kinematics and force via prosthetic sensors enabling predetermined states in various phases of gait). Foot microprocessors are often non-articulated such as the solid ankle cushion heel or energy storage and return systems (compression springs for propulsion). However, current systems still fall short in delivering embodiment and function.
Bionic prostheses are advanced active prostheses that aim to delivery subconscious, intuitive bidirectional control between an electronic device and the nervous system. Communication is via a human-machine interface, and despite advancing prostheses, the interface continues to pose numerous challenges. This technology has witnessed significant advances, primarily from the application of myoelectric systems. Electrodes in contact with innervated muscle convey electromyographical signals to actuate prosthetic movement. A clinical study of 27 patients with 238 electrodes placed in this way recorded a failure rate below 2% after 16 years[40]. However, muscle fatigue and neighbouring “noise” recorded from adjacent electrical activity limit the quality of information processed[41]. Further critique includes many months of requisite training, meaning 30% of individuals abandon their myoelectric prostheses[42]. This approach is limited when few muscle groups remain in the residuum, such as that seen in more proximal amputations.
In a bid to increase muscle targets, targeted muscle reinnervation (TMR) was introduced by Kuiken et al.[43] in 2003 to augment upper extremity function (TMR). TMR has been less investigated and applied for the lower limb. The premise is that remaining nerves in the amputated limb when surgically coapted to target nerves supplying a regional muscle, provides intuitive contraction of the recipient’s muscle when the desired function of the native nerve is required (targets are completely denervated to avoid the generation of unwanted signals). For example, the tibial nerve, when coapted to semitendinosus and the common peroneal to the biceps femoris, contractions are recorded months later upon the desired movement of the absent muscle group[44]. Moreover, a reduction in neuroma pain, opioid use, and neuromodulator medication has been observed[45]. A surgically related technique employs a regenerative peripheral nerve interface, whereby residual motor fascicles are coapted with non-vascularised muscle grafts acting as a bioamplifier once innervated. Multiple fascicles can provide more signals over that from TMR, but the signals are smaller and less easily recorded[46].
Herr has described the agonist-antagonist myoneural interface (AMI). By having muscles of opposing functions, surgically brought together as a single unit, the proprioceptive relationship is preserved. High fidelity control of a prosthetic device is seen in a transtibial amputee. Functional electrical stimulation (FES) gives feedback to the AMI of the prosthetic position[47]. Healthy tissues are required making it a more reliable option at the time of primary amputation. The approaches described are still limited in sensory and proprioceptive feedback and using muscle targets from unrelated neural inputs make myoelectric systems feel unnatural and non-intuitive[48].
THE EVOLVING ROLE OF BIONIC TECHNOLOGY AND NERVOUS SYSTEM CONTROL
A less investigated approach is to directly communicate with peripheral nerves [Electroneurography (ENG)][49], and may offer the solution to restore sensibility. This is important given grasp, and object manipulation is reliant upon afferent feedback. To enable a richer, natural feel and interaction with one’s environment require an appreciable understanding of the nervous system. Different levels within the somatosensory system are current research targets ranging from peripheral nerves to the brainstem. Peripheral nerves have both efferent (motor) and efferent (sensation via cutaneous mechanoreceptors, proprioceptors, thermoreceptors, and nociceptors) fibres, broadly divided into unmyelinated or myelinated axons (Schwann cells develop insulating myelin sheath). Large, myelinated fibres have the lowest resistance due to the increased density of voltage-dependent ion channels. Afferent fibres transmit signals from mechanical, thermal or noxious stimuli and similarly are unmyelinated or myelinated. The biomimetic approach faces two major challenges. Firstly, neural interfaces are limited in the number of channels and for individual channels to reliably stimulate distinct afferents is challenging. Aside from recording, algorithms for meaningful processing into human translation is complex. Secondly, the nature of the implants is called into question. As electrodes become more invasive, greater selectivity is achieved in addition to the quality of recorded signals. This comes at a cost, given invasive electrodes can induce injury to the nerve. The placement of the electrode can be broadly defined by its relationship with the epineurium [Figure 2].
Figure 2. Despite electrodes conveying signals from skin and muscle, more recent advances enable direct communication with the peripheral nervous system. Examples include the (1) Cuff; (2) FINE; (3) Sieve; (4) TIME; (5) USEA electrodes. FINE: Flat interface nerve electrode; TIME: transverse intra-fascicular multichannel electrode.
Extraneural electrodes
The perineurium is not penetrated with the best-known type referred to as the “cuff electrode” that encircles the nerve[50]. Comparatively, they are less selective than those breaching the perineurium but reliable for large myelinated fibres located in the periphery of the nerve[51]. Cuff electrodes are considered less traumatic, invasive and more stable than intra-fascicular electrodes at the current time. Implantation of several cuffs to reach numerous fascicles is possible and has been used to correct foot drop[52] and aid in standing after paralysis from spinal cord injury when wrapped around the femoral nerve using FES[53]. A recent variant of the cuff electrode is the flat interface nerve electrode (FINE), improving selectivity and contacts as the cuff applies pressure to the nerve enlarging its cross-section and displacing the axons from their central location[54]. In humans, FINE placement on the femoral nerve selectively and independently activates numerous muscle groups supplied by the nerve[55]. Concerns have been raised about electrode induced induced injury, and this remains unknown.
Interfascicular electrodes
Interfascicular electrodes aimed to supersede extraneural electrodes with the appreciation that motor and sensory nerve topography is heterogenous, changing course along the length of major nerves. The slowly penetrating interfascicular nerve electrode bluntly separates the epineurium on insertion to embed itself between fascicles. Selective axonal recruitment is seen in cat sciatic nerve with recruitment at different points in the nerve, suggestive of greater selectivity[56]. Given that restoration of sensation is crucial, this has driven research to investigate whether a stable direct communication with nerve fibers can be achieved.
Intraneural electrodes
These electrodes are in direct contact with nerve fibres. There is close contact with fascicles in both superficial and deep locations, meaning thresholds for axonal stimulation are lowered, improving selectivity and minimising noise[51]. One advantage is that lower stimulation currents are required for sensation[57]. Examples include the longitudinal intra-fascicular multichannel electrode (LIFE), whereby contacts are aligned along the parallel length of fibres. Their location makes them able to record and stimulate small groups of axons with high selectivity after implantation, seen in several amputees with meaningful sensory feedback[58]. The transverse intra-fascicular multichannel electrode implant is placed perpendicular to the nerve to access several fascicles[59]. In rat sciatic nerve, high selectively enabled activation of multiple downstream muscles. Less axonal injury is seen 2 months after implantation and shown to have higher selectivity than LIFE and cuff electrodes[51].
Multielectrode arrays have multiple electrode needles permitting multi-site recording and stimulation penetrating the nerve at varying depths perpendicularly along the length of the nerve[60]. The concern is intraneural damage and the potential for poor stability of recorded signals over time. A single array implanted in cat sciatic nerve for 350 days found persistent inflammation[61], and electrically stimulating a large population of afferents via a single electrode is unnatural and can evoke paraesthesia’s[62].
Regenerative electrodes
Regenerative electrodes are the most invasive yet have the greatest contact with the largest number of axons. The earliest example, devised 50 years ago, is the “sieve electrode”. Fine holes reside close to the nerve, and as fibres regenerate, they regrow through holes for selective stimulation. The prospect of growth factor delivery targeting specific neural populations electrodes will enable targeted delivery[63], and seems a plausible method considering our understanding of how nerves attempt to regenerate. Nerve regrowth takes time, and some concerns are that motor and sensory axons regrow in a disarrayed fashion than intact nerves[64]. Small unmyelinated axons have a higher growth capacity than large, myelinated axons, favouring centrally located small axons and large at the periphery of the electrode[65]. Promising results after 30 months is observed in cats[66]. One potential avenue for exploration includes the guidance of regenerating axons into small fascicles via neurite growth promotion down microchannels augmented by neurotrophic factors or scaffolds[67]. This has been seen to work well experimentally in the tibial nerve of rats[67]. Limitations of ENG decoding include overcoming the fibrotic response, minimised by increasing the degree of charge stimulated with further concerns about neural injury[68].
FUTURE CHALLENGES IN DEVELOPING LOWER LIMB BIONIC TECHNOLOGY
Commercially available prostheses do not have volitional control, especially where it is needed, the ankle. We know that sensorimotor interruption after limb loss leads amputees to have reduced confidence in maintaining balance, increasing falls risk and bearing greater cognitive burden[69]. Humans combine sensory, visual, vestibular, cognitive, and proprioceptive inputs to their surrounding environment[70]. A sensory neuroprosthesis that modulated stimulated-evoked sensation in response to interactions between the prosthesis and the environment was developed to study unilateral lower-limb amputees and their adaptation to the loss of plantar sensation. Six BKAs were compared with 14 able-bodied individuals, and they demonstrated higher foot placement accuracy. They were able to demonstrate that sensory neuroprosthesis provides modulated feedback of plantar pressure that improves lower limb performance in locomotor tasks[70].
Prostheses are heavy, need power, often noisy and require skilled repair when damaged. This is especially pertinent to overcome these barriers considering compliance declines with time for prostheses[71]. Modern bionic prostheses limit data transfer across biological-mechanical interfaces, making them slow with low bandwidth with wireless communication a priority, in addition to lead migration and fracture occurring in 24%-50% of events and wireless chips question how such systems can be appropriately powered[72,73]. Similarly, electromagnetic interference can arise from the interface as a result of tissue reactions with blood, matrix formation, inflammation, granulation tissue, foreign body reaction and fibrosis with the potential for a hypersensitivity reaction[74]. Long-term stability and reliability need further insight, and one avenue of research is tissue engineered neural interfaces that integrate encapsulated neural cells within the electrode to mitigate scar tissue formation and increase biocompatibility[75].
CONCLUSION
Outcomes after lower extremity trauma continue to improve. The growing expertise in preoperative, intraoperative, and postoperative management of complex cases, embedded within specialist centres, is now met with technological advances that supplant the sole use of autologous and allogeneic options when soft tissue availability is limited. Bionic technology is not new and has been investigated at numerous stages for clinical integration. The combined efforts of computational scientists, mechatronics, robotics and material experts working within the field, each delivering unique input, will one day potentially deliver a bespoke, intuitively controlled limb that has a human look, feel and functionality.
DECLARATIONS
Authors’ contributionsThe author contributed solely to the article.
Availability of data and materialsNot applicable.
Financial support and sponsorshipNone.
Conflicts of interestThe author declared that there are no conflicts of interest.
Ethical approval and consent to participateNot applicable.
Consent for publicationNot applicable.
Copyright© The Author(s) 2022.
REFERENCES
1. McCarthy ML, MacKenzie EJ, Edwin D, Bosse MJ, Castillo RC, Starr A. LEAP study group. Psychological distress associated with severe lower-limb injury. J Bone Joint Surg Am 2003;85:1689-97.
2. Robbins CB, Vreeman DJ, Sothmann MS, Wilson SL, Oldridge NB. A review of the long-term health outcomes associated with war-related amputation. Mil Med 2009;174:588-92.
3. Gailey R, Allen K, Castles J, Kucharik J, Roeder M. Review of secondary physical conditions associated with lower-limb amputation and long-term prosthesis use. J Rehabil Res Dev 2008;45:15-29.
4. Ephraim PL, Wegener ST, MacKenzie EJ, Dillingham TR, Pezzin LE. Phantom pain, residual limb pain, and back pain in amputees: results of a national survey. Arch Phys Med Rehabil 2005;86:1910-9.
5. Bourke HE, Yelden KC, Robinson KP, Sooriakumaran S, Ward DA. Is revision surgery following lower-limb amputation a worthwhile procedure? Injury 2011;42:660-6.
6. Higgins TF, Klatt JB, Beals TC. Lower Extremity Assessment Project (LEAP)--the best available evidence on limb-threatening lower extremity trauma. Orthop Clin North Am 2010;41:233-9.
7. Livingston DH, Keenan D, Kim D, Elcavage J, Malangoni MA. Extent of disability following traumatic extremity amputation. J Trauma 1994;37:495-9.
8. Huang CT, Jackson JR, Moore NB, et al. Amputation: energy cost of ambulation. Arch Phys Med Rehabil 1979;60:18-24.
9. Court-Brown CM, Bugler KE, Clement ND, Duckworth AD, McQueen MM. The epidemiology of open fractures in adults. A 15-year review. Injury 2012;43:891-7.
10. Court-Brown CM, McBirnie J. The epidemiology of tibial fractures. J Bone Joint Surg Br 1995;77:417-21.
11. MacKenzie EJ, Bosse MJ, Kellam JF, et al. Characterization of patients with high-energy lower extremity trauma. J Orthop Trauma 2000;14:455-66.
12. Aaron RK, Herr HM, Ciombor DM, et al. Horizons in prosthesis development for the restoration of limb function. J Am Acad Orthop Surg 2006;14:S198-204.
13. MacKenzie EJ, Bosse MJ, Kellam JF, et al. LEAP Study Group. Factors influencing the decision to amputate or reconstruct after high-energy lower extremity trauma. J Trauma 2002;52:641-9.
14. Bosse MJ, MacKenzie EJ, Kellam JF, et al. An analysis of outcomes of reconstruction or amputation after leg-threatening injuries. N Engl J Med 2002;347:1924-31.
15. Hierner R, Berger AK, Frederix PR. Lower leg replantation--decision-making, treatment, and long-term results. Microsurgery 2007;27:398-410.
16. Khouri RK, Shaw WW. Reconstruction of the lower extremity with microvascular free flaps: a 10-year experience with 304 consecutive cases. J Trauma 1989;29:1086-94.
17. Culliford AT 4th, Spector J, Blank A, Karp NS, Kasabian A, Levine JP. The fate of lower extremities with failed free flaps: a single institution’s experience over 25 years. Ann Plast Surg 2007;59:18-21; discussion 21-2.
18. Nho SJ, Helfet DL, Rozbruch SR. Temporary intentional leg shortening and deformation to facilitate wound closure using the Ilizarov/Taylor spatial frame. J Orthop Trauma 2006;20:419-24.
19. Aronson J. Limb-lengthening, skeletal reconstruction, and bone transport with the Ilizarov method. J Bone Joint Surg Am 1997;79:1243-58.
20. Papakostidis C, Bhandari M, Giannoudis PV. Distraction osteogenesis in the treatment of long bone defects of the lower limbs: effectiveness, complications and clinical results; a systematic review and meta-analysis. Bone Joint J 2013;95-B:1673-80.
21. Heller L, Phillips K, Levin LS. Pedicled osteocutaneous fibula flap for reconstruction in the lower extremity. Plast Reconstr Surg 2002;109:2037-42.
22. Dorafshar AH, Seitz IA, DeWolfe M, Agarwal JP, Gottlieb LJ. Split lateral iliac crest chimera flap: utility of the ascending branch of the lateral femoral circumflex vessels. Plast Reconstr Surg 2010;125:574-81.
23. Jeng SF, Kuo YR, Wei FC, Wang JW, Chen SH. Concomitant ipsilateral pedicled fibular transfer and free muscle flap for compound tibial defect reconstruction. Ann Plast Surg 2001;47:47-52.
24. Engel H, Lin CH, Wei FC. Role of microsurgery in lower extremity reconstruction. Plast Reconstr Surg 2011;127 Suppl 1:228S-38S.
25. Masquelet AC, Fitoussi F, Begue T, Muller GP. [Reconstruction of the long bones by the induced membrane and spongy autograft]. Ann Chir Plast Esthet 2000;45:346-53.
26. Bakri K, Stans AA, Mardini S, Moran SL. Combined massive allograft and intramedullary vascularized fibula transfer: the capanna technique for lower-limb reconstruction. Semin Plast Surg 2008;22:234-41.
27. Ziegler-Graham K, MacKenzie EJ, Ephraim PL, Travison TG, Brookmeyer R. Estimating the prevalence of limb loss in the United States: 2005 to 2050. Arch Phys Med Rehabil 2008;89:422-9.
28. Thaunat O, Badet L, Dubois V, Kanitakis J, Petruzzo P, Morelon E. Immunopathology of rejection: do the rules of solid organ apply to vascularized composite allotransplantation? Curr Opin Organ Transplant 2015;20:596-601.
29. Bermú Dez L, Santamaría A, Romero T, Calderó DF. Experimental model of facial transplant. Plast Reconstr Surg 2002;110:1374-5.
30. Gorantla VS, Barker JH, Jones JW, Prabhune K, Maldonado C, Granger DK. Immunosuppressive agents in transplantation: Mechanisms of action and current anti-rejection strategies. Microsurgery 2000;20:420-9.
31. Cavadas PC, Thione A, Carballeira A, Blanes M. Bilateral transfemoral lower extremity transplantation: result at 1 year. Am J Transplant 2013;13:1343-9.
32. Schirò GR, Sessa S, Piccioli A, Maccauro G. Primary amputation vs limb salvage in mangled extremity: a systematic review of the current scoring system. BMC Musculoskelet Disord 2015;16:372.
33. Battiston B, Tos P, Pontini I, Ferrero S. Lower limb replantations: indications and a new scoring system. Microsurgery 2002;22:187-92.
34. Koehler-McNicholas SR, Lipschutz RD, Gard SA. The biomechanical response of persons with transfemoral amputation to variations in prosthetic knee alignment during level walking. J Rehabil Res Dev 2016;53:1089-106.
35. Souza JM, Mioton LM, Harrington CJ, Potter BK, Forsberg JA. Osseointegration of extremity prostheses: a primer for the plastic surgeon. Plast Reconstr Surg 2020;146:1394-403.
36. Pezzin LE, Dillingham TR, Mackenzie EJ, Ephraim P, Rossbach P. Use and satisfaction with prosthetic limb devices and related services. Arch Phys Med Rehabil 2004;85:723-9.
37. Hagberg K, Brånemark R, Gunterberg B, Rydevik B. Osseointegrated trans-femoral amputation prostheses: prospective results of general and condition-specific quality of life in 18 patients at 2-year follow-up. Prosthet Orthot Int 2008;32:29-41.
38. Hagberg K, Hansson E, Brånemark R. Outcome of percutaneous osseointegrated prostheses for patients with unilateral transfemoral amputation at two-year follow-up. Arch Phys Med Rehabil 2014;95:2120-7.
39. Hagberg K, Ghassemi Jahani SA, Kulbacka-Ortiz K, Thomsen P, Malchau H, Reinholdt C. A 15-year follow-up of transfemoral amputees with bone-anchored transcutaneous prostheses. Bone Joint J 2020;102-B:55-63.
40. Kilgore KL, Peckham PH, Keith MW, et al. Durability of implanted electrodes and leads in an upper-limb neuroprosthesis. J Rehabil Res Dev 2003;40:457-68.
41. Solomonow M. External control of the neuromuscular system. IEEE Trans Biomed Eng 1984;31:752-63.
42. Winslow BD, Ruble M, Huber Z. Mobile, game-based training for myoelectric prosthesis control. Front Bioeng Biotechnol 2018;6:94.
43. Kuiken TA, Li G, Lock BA, et al. Targeted muscle reinnervation for real-time myoelectric control of multifunction artificial arms. JAMA 2009;301:619-28.
44. Hargrove LJ, Simon AM, Young AJ, et al. Robotic leg control with EMG decoding in an amputee with nerve transfers. N Engl J Med 2013;369:1237-42.
45. Frantz TL, Everhart JS, West JM, Ly TV, Phieffer LS, Valerio IL. Targeted muscle reinnervation at the time of major limb amputation in traumatic amputees: early experience of an effective treatment strategy to improve pain. JB JS Open Access 2020;5:e0067.
46. Frost CM, Ursu DC, Flattery SM, et al. Regenerative peripheral nerve interfaces for real-time, proportional control of a Neuroprosthetic hand. J Neuroeng Rehabil 2018;15:108.
47. Herr HM, Grabowski AM. Bionic ankle-foot prosthesis normalizes walking gait for persons with leg amputation. Proc Biol Sci 2012;279:457-64.
48. Hargrove LJ, Young AJ, Simon AM, et al. Intuitive control of a powered prosthetic leg during ambulation: a randomized clinical trial. JAMA 2015;313:2244-52.
49. Davis TS, Wark HA, Hutchinson DT, et al. Restoring motor control and sensory feedback in people with upper extremity amputations using arrays of 96 microelectrodes implanted in the median and ulnar nerves. J Neural Eng 2016;13:036001.
50. Walker CF, Lockhead GR, Markle DR, McElhaney JH. Parameters of stimulation and perception in an artificial sensory feedback system. J Bioeng 1977;1:251-6.
51. Badia J, Boretius T, Andreu D, Azevedo-Coste C, Stieglitz T, Navarro X. Comparative analysis of transverse intrafascicular multichannel, longitudinal intrafascicular and multipolar cuff electrodes for the selective stimulation of nerve fascicles. J Neural Eng 2011;8:036023.
52. Valle J, Navarro X. Interfaces with the peripheral nerve for the control of neuroprostheses. Int Rev Neurobiol 2013;109:63-83.
53. Fisher LE, Miller ME, Bailey SN, et al. Standing after spinal cord injury with four-contact nerve-cuff electrodes for quadriceps stimulation. IEEE Trans Neural Syst Rehabil Eng 2008;16:473-8.
54. Caparso AV, Durand DM, Mansour JM. A nerve cuff electrode for controlled reshaping of nerve geometry. J Biomater Appl 2009;24:247-73.
55. Schiefer MA, Polasek KH, Triolo RJ, Pinault GC, Tyler DJ. Selective stimulation of the human femoral nerve with a flat interface nerve electrode. J Neural Eng 2010;7:26006.
56. Tyler DJ, Durand DM. A slowly penetrating interfascicular nerve electrode for selective activation of peripheral nerves. IEEE Trans Rehabil Eng 1997;5:51-61.
57. Grinberg Y, Schiefer MA, Tyler DJ, Gustafson KJ. Fascicular perineurium thickness, size, and position affect model predictions of neural excitation. IEEE Trans Neural Syst Rehabil Eng 2008;16:572-81.
58. Dhillon GS, Lawrence SM, Hutchinson DT, Horch KW. Residual function in peripheral nerve stumps of amputees: implications for neural control of artificial limbs. J Hand Surg Am 2004;29:605-15; discussion 616-8.
59. Boretius T, Badia J, Pascual-Font A, et al. A transverse intrafascicular multichannel electrode (TIME) to interface with the peripheral nerve. Biosens Bioelectron 2010;26:62-9.
60. Ledbetter NM, Ethier C, Oby ER, et al. Intrafascicular stimulation of monkey arm nerves evokes coordinated grasp and sensory responses. J Neurophysiol 2013;109:580-90.
61. Clark GA, Ledbetter NM, Warren DJ, Harrison RR. Recording sensory and motor information from peripheral nerves with Utah Slanted Electrode Arrays. Annu Int Conf IEEE Eng Med Biol Soc 2011;2011:4641-4.
62. Tan DW, Schiefer MA, Keith MW, Anderson JR, Tyler J, Tyler DJ. A neural interface provides long-term stable natural touch perception. Sci Transl Med 2014;6:257ra138.
63. Lotfi P, Garde K, Chouhan AK, Bengali E, Romero-Ortega MI. Modality-specific axonal regeneration: toward selective regenerative neural interfaces. Front Neuroeng 2011;4:11.
64. Hallin RG. Microneurography in relation to intraneural topography: somatotopic organisation of median nerve fascicles in humans. J Neurol Neurosurg Psychiatry 1990;53:736-44.
65. Castro J, Negredo P, Avendaño C. Fiber composition of the rat sciatic nerve and its modification during regeneration through a sieve electrode. Brain Res 2008;1190:65-77.
66. Panetsos F, Avendaño C, Negredo P, Castro J, Bonacasa V. Neural prostheses: electrophysiological and histological evaluation of central nervous system alterations due to long-term implants of sieve electrodes to peripheral nerves in cats. IEEE Trans Neural Syst Rehabil Eng 2008;16:223-32.
67. Clements IP, Mukhatyar VJ, Srinivasan A, Bentley JT, Andreasen DS, Bellamkonda RV. Regenerative scaffold electrodes for peripheral nerve interfacing. IEEE Trans Neural Syst Rehabil Eng 2013;21:554-66.
68. la Oliva N, Mueller M, Stieglitz T, Navarro X, Del Valle J. On the use of Parylene C polymer as substrate for peripheral nerve electrodes. Sci Rep 2018;8:5965.
69. Howard CL, Wallace C, Abbas J, Stokic DS. Residual standard deviation: validation of a new measure of dual-task cost in below-knee prosthesis users. Gait Posture 2017;51:91-6.
70. Christie BP, Charkhkar H, Shell CE, Burant CJ, Tyler DJ, Triolo RJ. Ambulatory searching task reveals importance of somatosensation for lower-limb amputees. Sci Rep 2020;10:10216.
71. Biddiss EA, Chau TT. Upper limb prosthesis use and abandonment: a survey of the last 25 years. Prosthet Orthot Int 2007;31:236-57.
72. Farinetti A, Ben Gharbia D, Mancini J, Roman S, Nicollas R, Triglia JM. Cochlear implant complications in 403 patients: comparative study of adults and children and review of the literature. Eur Ann Otorhinolaryngol Head Neck Dis 2014;131:177-82.
73. Kumar K, Wilson JR, Taylor RS, Gupta S. Complications of spinal cord stimulation, suggestions to improve outcome, and financial impact. J Neurosurg Spine 2006;5:191-203.
74. Velnar T, Bunc G, Klobucar R, Gradisnik L. Biomaterials and host versus graft response: a short review. Bosn J Basic Med Sci 2016;16:82-90.
Cite This Article

How to Cite
Download Citation
Export Citation File:
Type of Import
Tips on Downloading Citation
Citation Manager File Format
Type of Import
Direct Import: When the Direct Import option is selected (the default state), a dialogue box will give you the option to Save or Open the downloaded citation data. Choosing Open will either launch your citation manager or give you a choice of applications with which to use the metadata. The Save option saves the file locally for later use.
Indirect Import: When the Indirect Import option is selected, the metadata is displayed and may be copied and pasted as needed.
About This Article
Special Issue
Copyright
Data & Comments
Data
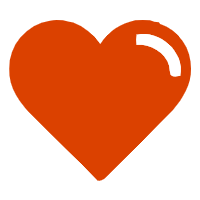
Comments
Comments must be written in English. Spam, offensive content, impersonation, and private information will not be permitted. If any comment is reported and identified as inappropriate content by OAE staff, the comment will be removed without notice. If you have any queries or need any help, please contact us at [email protected].