Mechanisms regulating mitochondrial DNA quality control
Abstract
Maintenance of genome integrity is essential for cellular survival. There are mechanisms utilized by the cells to sense and respond to assaults on genomic DNA. These mechanisms are conserved across all domains of life and are collectively called the DNA damage response pathways. However, eukaryotic cells also have extrachromosomal DNA in mitochondria (mtDNA), which is indispensable for mitochondrial function, and hence cell survival. Indeed, impaired mitochondrial activity arising due to mutations in mtDNA has been found to be associated with many human pathologies. Despite its importance, our understanding of how cells ensure mtDNA genome integrity is limited. Since mitochondria do not encode for machinery required for the maintenance of their own genomes, they depend on the nucleus for replication, transcription, and repair processes. This adds a layer of complexity with the requirement for organelle crosstalk and coordination in response to mtDNA damage. This review summarizes recent findings that provide new insights into mechanisms involved in mtDNA quality control, acting at the level of mtDNA or organelle and also discusses a few new avenues of research towards a comprehensive understanding of the “mtDNA damage response”.
Keywords
INTRODUCTION
Preservation of genome integrity is essential for the faithful propagation of life. Sources of DNA damage include endogenous factors such as errors during DNA replication, reactive oxygen species (ROS), and metabolic intermediates (like methylglyoxal), as well as exogenous agents including UV radiation and chemicals that can directly modify and damage the DNA[1,2]. Responses to such perturbations require mechanisms that can sense damage and repair aberrant DNA modifications. In eukaryotes, this entails sensing and responding to both nuclear and mitochondrial DNA (mtDNA) damage.
All eukaryotic cells, with a few exceptions, contain mitochondria which are double membrane-bound organelles. While chiefly the sites for oxidative phosphorylation, mitochondria are also involved in other cellular processes, including amino acid biosynthesis, Fe-S cluster biogenesis, and apoptosis[3-5]. Mitochondria are highly dynamic and undergo changes in shape and size throughout the cell cycle, independent of cell division[6]. Apart from playing an important role in cellular adaptation to different metabolic environments[7], this dynamicity is important for quality control, segregation, and distribution of the organelle as well as its DNA[7,8].
Mitochondria possess their own genomes (mtDNA), which are essential for mitochondrial function. These genomes vary drastically in size as well as numbers across eukaryotes[9]. For example, yeast mitochondrial genome is ~86 Kb in size and is present in ~10-80 copies per cell[10,11], while human mtDNA is ~16.5 Kb, and present in a few hundred to thousand copies per cell[12,13]. Mitochondrial genomes code for proteins that are part of the electron transport chain as well as tRNA and rRNA genes required for mitochondrial protein translation[10,14,15]. The DNA copies are packaged in the form of nucleoids, which are bound by many proteins involved in replication, transcription, and repair[16,17]. The most abundant of these is an HMG (high mobility group)-box containing protein, TFAM (Transcription factor A, mitochondrial in metazoans) or ABF2 (in budding yeast), which functions in the regulation of mtDNA packaging, transcription, and replication[18-23].
Similar to nuclear DNA, mtDNA also experiences damage. For example, studies have demonstrated that mtDNA faces relatively more oxidative damage than nuclear DNA[24,25], likely due to the proximity of mtDNA to the OXPHOS machinery[26]. Another source of perturbation to mtDNA is the intrinsic errors from mtDNA replication, which can result in mutations or deletions[27]. mtDNA mutations are associated with several human pathologies like LHON (Leber hereditary optic neuropathy), MELAS (Mitochondrial Encephalopathy, Lactic Acidosis, and Stroke-like episodes) syndrome, Leigh syndrome, etc.[28], and have been proposed to be an underlying cause for aging as well[29]. Thus, the maintenance of mtDNA integrity is critical for cell survival. How do cells sense and respond to mtDNA damage? Nuclear DNA damage triggers dedicated DNA damage responses that include cell cycle regulation as well as expression of genes directly involved in DNA repair[30]. Such a response in case of mtDNA damage remains to be uncovered. However, recent evidence suggests that cells do respond to mtDNA perturbations at two levels: DNA (via engaging in mtDNA repair or clearance) and organelle (via selective segregation or organelle clearance)[31-36]. The presence of multiple mechanisms that work on different levels adds complexity, as each pathway could have different consequences for the organelle and cell physiology. We point readers to excellent reviews covering topics of mtDNA damage repair, mitochondrial dynamics, and mitophagy in detail[6,8,37-43]. For this review, we limit our discussion to a few key studies that allow us to synthesize our broad understanding of mtDNA damage response and repair across scales (mtDNA, mitochondrial and cellular aspects). We discuss various pathways in light of their involvement in mtDNA quality control and highlight open areas to uncover a potential mtDNA damage response and downstream regulators of pathway choice [Figure 1].
Figure 1. Sources of mtDNA perturbations include DNA damage and errors associated with replication machinery resulting in mutations or deletions. Different mechanisms involved in mtDNA quality control: a: mtDNA damage repair, b: mtDNA degradation via POLG,
mtDNA QUALITY CONTROL: mtDNA REPAIR
There are several lines of evidence in support of proficient mtDNA damage repair across eukaryotic model systems[37-39]. Interestingly, these studies suggest that only certain types of damage might be repaired in mitochondria, with some classes of base modifications being tolerated efficiently by the replicative mtDNA polymerase, POLG/MIP1[44-47]. For example, the incorporation of rNMPs on mtDNA does not affect POLG stability or fidelity[44,47]. Indeed, there are also repair pathways that appear to be absent from mitochondria. For example, there is presently no evidence for mitochondrial nucleotide excision repair (NER)[48,49]. We direct readers to these excellent recent reviews for detailed repair pathway descriptions[37-39,50], and we highlight some major mechanisms here.
Base excision repair (BER) seems to be the most active repair pathway in mitochondria. The prevalence of BER can be appreciated by the fact that in somatic cells, the mutation landscape of mtDNA is devoid of G to T mutations, which are hallmarks of oxidative damage[51,52]. In support of BER activity on mtDNA, several DNA glycosylases have been found to localize to the mitochondria[53-59]. This includes OGG1 (8-oxoguanine DNA glycosylase) required for repair of 8-oxo-G lesions and UDG or UNG (Uracil DNA glycosylase) essential for removal of misincorporated uracils on DNA[53,58]. Indeed, inhibition of UNG1 activity leads to the accumulation of mutations on mtDNA in budding yeast[53]. Other than OGG1 and UNG1, two homologs of the human NTH1 (Endonuclease III-like protein 1), NTG1, and NTG2 with broad spectrum specificities, are also found in budding yeast. NTG1 dually localizes to both the nucleus and mitochondria[59-61] and preferentially translocates to the mitochondria under elevated mitochondrial oxidative stress[61]. Apart from NTH1, human cells also contain NEIL1 and NEIL2 glycosylases which are bi-functional and also have broad-spectrum specificities[55-57]. Some other glycosylases, such as MYH, could have tissue-specific mitochondrial expression, as shown in rat brains[54]. The idea that BER is fully active in mitochondria is further supported by the presence of accessory proteins, such as endonucleases, required in this pathway, including APE1, FEN1, and DNA2[62-66]. APE1 is required for hydrolyzing the phosphodiester bond at the apurinic/apyrimidinic site to generate a gap that is then filled by a polymerase[67,68]. While the mtDNA replicative polymerase POLG is known to participate in gap-filling, there is now increasing evidence to suggest that X family polymerase, POLβ, is involved in this gap filling in mammalian mitochondria[69-72], with evidence for brain tissue-specific localization[69]. The choice of polymerase for gap-filling could depend on the type of lesion and glycosylase recruited. For example, POLβ also has ~17-20 fold higher 5′-dRP lyase activity as compared to POLG[71,72], and this activity is required for the processing of ends generated by mono-functional glycosylases such as UNG1 or MUTYH along with APE1. Alternately, in scenarios where the lesion is acted upon by bi-functional glycosylases such as OGG1, NEIL1, or NEIL2, the ends generated can be processed by either TDP1, APTX, or PNKP (polynucleotide kinase
There is some evidence to suggest that translesion synthesis may also be active in mitochondria. There are several translesion synthesis (TLS) polymerases such as POLθ, REV1, and POLζ that localize to mitochondria[76], and affect mutation rates[77-79]. Apart from TLS, it is possible that these polymerases also participate in BER as observed in in vitro assays[80,81], but in vivo evidence for the same remains to be uncovered. Indeed, TLS itself can be carried out by POLG, which has been shown in vitro to synthesize across lesions like pyrimidine dimers and acrolein-derived exocyclic DNA adducts[82,83].
Apart from BER and TLS, there is sporadic evidence for the presence of other damage repair and tolerance systems in mitochondria. This includes mismatch repair[84] and double-strand break (DSB) repair[33,85,86]. While DSB repair is known to occur in plant mitochondria[87-90], it was widely believed to be non-existent in the mitochondria of animal cells. However, studies have shown that mitochondrial extracts obtained from mammalian cells are capable of DSB repair via HR (homologous recombination) or MMEJ (Micro-homology mediated end joining)[85,86,91,92]. These in vitro assays show end-joining activity in the mitochondrial extracts, leading to the formation of repair products with deletions[86,91]. Indeed, in mouse muscle cells, expression of a mitochondrial-targeted restriction enzyme, PstI, resulted in the formation of recombination products with large deletions, albeit at low frequencies[93]. Similarly, mitochondrial targeting of restriction enzyme, ScaI, in heteroplasmic mouse cells containing mtDNA haplotypes with different numbers of Sca1 restriction sites, resulted in the formation of both inter- and intra-molecular recombination products, with low levels of inter-molecular recombination[94]. In support of this, low frequencies of inter-molecular recombination products were also observed after the fusion of two human cytoplasmic hybrids with different mtDNA mutations[95]. In a similar study, the fusion of cybrids with mtDNA bearing different deletions allowed for functional complementation, but the genetic exchange between the two mtDNA nucleoids occurred at low frequencies[96]. More recently, using heteroplasmic mtDNA variants in Drosophila, recombination was shown to occur in vivo after induction of mtDSBs using mitochondrial-targeted restriction enzymes[97], and a screen for candidates involved in mtDNA repair revealed REC/MCM8 as a candidate involved in recombination-mediated repair[33]. Thus, these pieces of evidence show that DSB repair can occur in the mitochondria of animal cells. Future studies in this direction can further elucidate the importance of this pathway in mtDNA integrity maintenance under physiological conditions.
A particularly confounding feature of mtDNA damage is the fact that there are multiple copies of mtDNA in the cell. Thus, in the case of mtDNA damage, cells can choose between either repairing the damage or degrading the damaged DNA copy, followed by replication of wild-type mtDNA to restore copy number. Indeed, in case of mutations/deletions, clearance of damaged DNA copies and/or selective replication of intact mtDNA might be the only possible way of quality control. Whether damage on mtDNA is repaired or degraded could thus depend on the type of DNA damage as well as the amount of damage accumulated.
mtDNA QUALITY CONTROL: mtDNA CLEARANCE AND SELECTIVE REPLICATION
As an alternative to repair, multiple pieces of evidence show that in cases where damage cannot be repaired or is persistent, the mtDNA is degraded[31,98,99]. For example, UV-induced dimerization of pyrimidines, or ADP ribosylation on mtDNA leads to degradation of DNA[31,100,101]. In support, there are no reports of NER in mitochondria, which would be required for the repair of such lesions[48,49]. Even in the case of DNA breaks, mtDNA copies with DSBs are degraded and copy number is replenished by replicating wild-type mtDNA[98]. Studies utilizing mitochondrially-targeted restriction enzymes, TALENS, and ZFNs, also show that DSBs on mtDNA result in degradation, and these have been utilized to reduce the levels of heteroplasmy[102-106] [Table 1].
Different tools for specifically modifying mitochondria DNA
Tool | Mode of action | Type of modification | References |
Mito-ZFN (Zinc finger nucleases) | Zinc finger nucleases are targeted to mitochondria using a mitochondrial targeting sequences (MTS). The conventional design consists of the catalytic domain of type IIS restriction enzyme Fok1 attached to a specially designed Zinc Finger Peptide (ZFP) which gives sequence specificity. | Double-strand break | [105] |
Mito-TALENs (TALE based nucleases) | Work on the same principle as ZFNs; however, sequence specificity is brought about by a TALE (transcription activator- like effectors) instead of ZFPs. | Double-strand break | [104,106,107] |
Mito-Tev1-TALE or cTALEN (c:-compact) | Utilizes a T4 phage homing endonuclease I-Tev1 instead of Fok1. I-Tev1, unlike Fok1, does not require dimerization for cutting DNA. | Double-strand break | [108,109] |
Mitochondrial targeted restriction endonucleases Mito-XmaI Mito-SmaI Mito-PstI Mito-ScaI Mito-ApaLI Mito-ARCUS | Restriction endonucleases are targeted to mitochondria using a MTS. They cut DNA at specific recognition sites on mtDNA. | Double-strand break | [102,103,110-113] |
Mito-DarT | Utilizes a bacterial toxin DarT from a bacterial toxin-antitoxin system, which is targeted to mitochondria using a MTS. | ADP ribosylation on ssDNA on a thymidine residue in a “TNTC” motif | [31] |
Triphenyl phosphonium (TPP) conjugates TPP-Doxorubicin TPP-Chlorambucil TPP-Cisplatin TPP-Paraquat | TPP localizes to mitochondria in a membrane potential sensitive manner. | Doxorubicin- Double strand breaks Chlorambucil- alkylation Cisplatin- Crosslinks Paraquat- Increase in ROS levels | [114-117] |
Mitochondria penetrating peptides (MPPs) conjugates MPP-Doxorubicin MPP-Cisplatin MPP-Chlorambucil | MPPs are cationic, but lipophilic peptides whose penetration into mitochondria has been observed and this localization can be fine-tuned based on charge and lipophilicity. | Doxorubicin- Double strand breaks Cisplatin- Crosslinks Chlorambucil- alkylation | [118-120] |
Mitochondrial targeted Topoisomerase 1 mutant: TOP1-103 (R420K) | A substitution of arginine to lysine at position 420 of TOP1 results in a toxic mutant causing persistent single-strand breaks. | Single strand break | [121] |
Mito-FAP (mitochondrially targeted fluorogen-activating peptide) | Mito-FAP-MG-2I (Mito-FAP bound to iodine-substituted malachite green analog) complex generates singlet oxygen in the presence of NIR (near-infrared light). | Oxidative damage (Leads to an overall increase in ROS levels in the cell. Could damage nuclear DNA as well) | [122] |
How is mtDNA degradation carried out? Although multiple nucleases have been detected in the mitochondria, these do not appear to play a role in mtDNA degradation[98]. Instead, recent studies have shown that the mitochondrial replicative polymerase, POLG/MIP1, is capable of degrading mtDNA under damage or even in starvation conditions[31,98,123,124]. A role for MGME1 in the degradation of mtDNA has also been suggested, where the loss of MGME1 prevents the degradation of linearized mtDNA after induction of DSBs[98]. However, an alternate set of observations suggest that loss of MGME1 results in the de novo formation of linearized DNA fragments due to incomplete mtDNA replication and does not affect the degradation of mtDNA[125,126]. These studies suggest that POLG might play the primary role in the degradation of linearized mtDNA. However, how MIP1/POLG switches from a replicative to an exonuclease function is not fully understood. There is evidence suggesting that this switching is regulated by the levels of dNTPs in the cell[123,127]. Whether this results in the selective degradation of few mtDNA copies or “global” degradation of all mtDNA is not known. For example, it is possible that polymerase stalling at a DNA lesion triggers its exonuclease function[128,129], resulting in the degradation of only the damaged mtDNA copy. Such a mechanism would nicely couple mtDNA replication with genome integrity maintenance, and prevent unregulated DNA loss in case of damage. Indeed, selective degradation of damaged mtDNA would also contribute to selective mtDNA clearance as well as purifying selection via replication of non-damaged mtDNA.
Certainly, a combination of selective degradation and replication could contribute to mitochondrial genome integrity maintenance. This becomes particularly relevant in conditions of heteroplasmy[130]. In line with this, there is evidence to support that wild-type mtDNA is selectively replicated over mutant mtDNA in Drosophila cells[131-133]. For such selective replication to occur, cells would need to distinguish wild-type mtDNA from mutant or damaged mtDNA. As stated above, this could come from the replicative polymerase stalling at lesions, followed by selective degradation of that specific mtDNA copy. In addition to mtDNA-specific mechanisms, there is strong evidence for the regulation of selective degradation/ replication occurring at the level of the mitochondria itself.
Recently, local protein translation was shown to be dependent on mitochondrial membrane potential in Drosophila germline cells[133]. PINK1 accumulation on depolarized mitochondria prevents protein translation on the outer mitochondrial membrane, thereby reducing the abundance of proteins (including replisome components) in mitochondria[133]. Similarly, mitochondria with lower membrane potentials cannot undergo fusion[134-136], and mitochondrial fusion is known to affect mitochondrial DNA replication in both mammalian and budding yeast cells[137,138]. Given that preventing mitochondrial membrane fusion by MFN1 and MFN2 or OPA1 knockout resulted in an imbalance of replisome components (like POLG, TWINKLE, and SSBP1) in mitochondria[138], a mechanism for such reduced mtDNA replication can be hypothesized. However, for this mitochondrial activity-driven effect to dictate specificity in replication, the influence of mutations or damage on protein quality would need to be localized in the close vicinity of the damaged nucleoid(s). In support of this idea, in budding yeast, mtDNA-encoded ATP6 was shown to have limited diffusion, depending on cristae morphology[139]. This would result in the compartmentalization of mutant mt-nucleoids as well as its effects on mitochondrial activity. Such compartmentalization could further contribute to the selective shutdown of replication in these regions, driving selective DNA replication of wild-type mtDNA copies instead.
It is important to note that there are examples that are counter to the mechanism of selective replication of wild-type mtDNA described above. In contrast to Drosophila, where PINK1 localization to depolarized mitochondria suppresses mutant mtDNA replication[133], studies in C. elegans have shown an expansion of mtDNA carrying deletions[140,141]. This expansion is dependent on the activation of the UPRmt (mitochondrial unfolded protein response), resulting from OXPHOS dysfunction[142]. Activation of the UPRmt leads to accumulation of ATFS-1 in mitochondria with impaired OXPHOS machinery, finally resulting in increased binding of POLG on mtDNA[143]. Furthermore, active replication is probably not the only factor regulating selectivity; even within a cell that only has wild-type mtDNA, not all DNA copies are replicated[144]. In such instances as well, mtDNA damage will affect mitochondrial function and cells must have additional mechanisms to cope with the same.
MITOCHONDRIAL QUALITY CONTROL UNDER CONDITIONS OF mtDNA DAMAGE
An important layer of mitochondrial genome integrity maintenance appears at the organelle level. As discussed in the previous section, the effect of mtDNA perturbation can adversely impact local mitochondrial activity as well[139]. This in turn can activate quality control mechanisms that function at the level of the organelle. Multiple mitochondrial quality control pathways have been shown to function in response to mitochondrial stress, including membrane depolarization, proteotoxic stress, or ROS accumulation, among others[145-147]. A key step in this process is the re-organization of the mitochondrial network to physically separate impaired and fit mitochondria[148]. Both mitochondrial fission and fusion play essential roles in re-organizing the mitochondrial network[40,145]. Indeed, mitochondrial fragmentation has been observed in response to both mtDNA damage and the presence of mtDNA with mutations[31,32,34]. This is then accompanied by either a. mitochondrial clearance and/ or b. selective mitochondrial segregation [Figure 2].
Figure 2. Fragmentation of mitochondrial network results from perturbed mitochondrial activity to compartmentalize mitochondria with impaired function. These fragmented mitochondria have different fates: (A) active clearance via pathways like mitophagy and (B) selective segregation with retention of impaired mitochondria in one cell.
1. Active clearance of mitochondria mainly occurs via a process called mitophagy (mitochondria-specific autophagy)[41]. Other recently discovered mechanisms that help in mitochondrial protein quality control are via the formation of MDVs (Mitochondria Derived Vesicles) and SPOTs (Structures Positive for Outer Membrane), which are then actively degraded in the cell[149-151]. Clearance of any impaired mitochondria via such mitochondrial quality control pathways could passively clear out mtDNA with mutations or damage as well. In support of this mechanism, in Drosophila germline cells, clearance of deleterious mutations is dependent on the fragmentation of the mitochondrial network[34]. Fragmented mitochondria are ultimately degraded via mitophagy[34]. Similarly, even in mammalian cell lines, mtDNA damage-associated fragmentation is followed by activation of autophagy[32]. In Drosophila, such mitophagy appears to be independent of the mutation load and is programmed to occur at a specific stage of development[35]. Even in somatic cells in Drosophila and C. elegans, activation of the PINK1/Parkin pathway under lower levels of Mitofusin results in purifying selection of mtDNA[152-154]. These data suggest a role for mitophagy in conjunction with mitochondrial fragmentation in mtDNA purification.
2. Additionally, separation of impaired and fit mitochondria could also allow for selective segregation of organelles during cell division, independent of mitophagy. For example, in budding yeast, it has been found that “fitter” mitochondria are segregated into the daughter cells, while “unfit” mitochondria are retained in the mother cell[155]. Fitness, in this scenario, is defined in terms of ROS levels, presence of protein aggregates, and redox states of the mitochondria[155]. During division, daughter cells receive mitochondria with lower ROS levels and higher redox states, thereby also generating mother-daughter age asymmetry[155]. Such an asymmetry in mitochondrial segregation could contribute to selective segregation of mtDNA, without the need for active degradation. In support, recent work suggests that under mtDNA damage, mitochondrial fragmentation is not followed by mitophagy, and instead, this fragmentation could facilitate asymmetric segregation of the organelle[31]. Indeed, even in adult stem cells, segregation of mitochondria during division is asymmetric, where one cell receives “old” mitochondria whereas the other cell receives “young” mitochondria[156,157]. Whether this also translates to mtDNA quality control in these mitochondria remains to be assessed.
FUTURE PERSPECTIVES
It is becoming increasingly evident that mtDNA quality control is important and that diverse mechanisms act at the level of mtDNA as well as mitochondria to regulate the same. However, key questions about the relative importance of these mechanisms, the choice of pathway employed, and the cellular impact of the pathway(s) used remain to be answered. A previous lacuna in the field was the challenge associated with specifically perturbing mtDNA integrity, avoiding confounding effects on nuclear DNA. The toolkit to generate specific types of DNA modifications on mtDNA is now expanding [Table 1] and will enable researchers to disentangle the roles played by DNA repair, degradation, and organellar regulation in the maintenance of mtDNA integrity. More importantly, these tools can address whether cells encode an mtDNA-specific damage response, such as those mounted in response to nuclear DNA damage[30]. In this direction, we highlight the following open questions:
(1) How is mtDNA damage sensed?
(2) How is the damage signal relayed from mitochondria to the nucleus?
(3) What is the machinery involved in carrying out this sensing and relaying?
In the case of nuclear DNA damage, cells sense damage via ATM/ATR proteins which are recruited at the site of damage following replication or transcription blocks[158,159]. It is unclear whether mtDNA damage also activates such a response. Indeed, some studies show localization of ATR in mitochondria where it plays an anti-apoptotic role[160]. There is also evidence that CHK2 activation can affect mtDNA synthesis, independent of mtDNA damage[161]. However, the involvement of such responses in mtDNA damage is not known. Koczor et al., did observe CHK2 activation in response to increased ROS production in HeLa cells[162]. This response was deactivated when mtDNA-associated BER proteins were overexpressed, suggesting a link between mtDNA integrity and DNA damage checkpoint activation. The mechanism of activation of this response via mitochondrially-relayed signals (if any) and what is the functional significance of the same remains to be determined. Identification of the mechanism of this activation would reveal new insights into mitochondria-nuclear signaling and crosstalk.
Recent studies suggest that mtDNA damage can also trigger other responses in the cell. For example, mtDSBs have been shown to activate the integrated stress response via the OMA1-DELE1-HRI pathway[163]. In addition, mtDNA stress can also result in the release of mtDNA and/ or mtRNA into the cytosol[36,164-168]. This has been shown to activate the type-1 interferon response via the cGas-STING pathway (in case of mtDNA) and RIG1/MDA-5-MAVS pathway (in case of mtds-RNA)[36,164-168]. In some of these cases, there appears to be a direct connection between the regulation of mitochondrial cristae morphology and signal transduction from the mitochondria to the cytosol, likely mediated by ATAD3[163,167]. ATAD3 is required for the attachment of mtDNA to the inner mitochondrial membrane and is also implicated in the regulation of cristae morphology[169-171]. The central role of ATAD3 in mitochondria-nuclear signaling under mtDNA stress would suggest that inner membrane architecture could be a potential marker for mtDNA dysfunction[172,173]. It would be insightful to assess whether other mtDNA structuring and organizing proteins[174,175] also contribute to such signaling mechanisms.
Taken together, even at the level of signaling, there appear to be contributions at the level of mtDNA and the organelle. As discussed above, it is possible that the responses to mtDNA perturbations vary dependent on the amount and type of mtDNA damage faced by the cell, as well as growth conditions and cellular contexts[31,32,163,176]. Careful delineation of these mechanisms can reveal the general principles of an mtDNA damage response as well as specific context-dependent features that could contribute to pathway choice and associated heterogeneity in purifying selections[177]. Ultimately, these insights can enable us to target mtDNA quality control mechanisms in pathological conditions.
DECLARATIONS
Acknowledgments
We thank members of the AB and Sunil Laxman labs for their helpful comments and discussions.
Authors’ contributions
Conceptualized this review and contributed to the writing of the manuscript: Dua N, Badrinarayanan A
Availability of data and materials
Not applicable.
Financial support and sponsorship
This work is supported by Human Frontier of Science Programme grant (Grant number IA/I/21/1/505630) and intramural funding via NCBS-TIFR (Grant number 03/3/2019/R&D-II/DAE/4749).
Conflicts of interest
All authors declared that there are no conflicts of interest.
Ethical approval and consent to participate
Not applicable.
Consent for publication
Not applicable.
Copyright
© The Author(s) 2023.
REFERENCES
1. Chatterjee N, Walker GC. Mechanisms of DNA damage, repair, and mutagenesis. Environ Mol Mutagen 2017;58:235-63.
2. Moretton A, Loizou JI. Interplay between cellular metabolism and the DNA damage response in cancer. Cancers 2020;12:2051.
3. Braymer JJ, Lill R. Iron-sulfur cluster biogenesis and trafficking in mitochondria. J Biol Chem 2017;292:12754-63.
5. Murphy E, Ardehali H, Balaban RS, et al. Mitochondrial function, biology, and role in disease: a scientific statement from the american heart association. Circ Res 2016;118:1960-91.
10. Foury F, Roganti T, Lecrenier N, Purnelle B. The complete sequence of the mitochondrial genome of Saccharomyces cerevisiae. FEBS Lett 1998;440:325-31.
11. Göke A, Schrott S, Mizrak A, Belyy V, Osman C, Walter P. Mrx6 regulates mitochondrial DNA copy number in Saccharomyces cerevisiae by engaging the evolutionarily conserved Lon protease Pim1. Mol Biol Cell 2020;31:527-45.
12. Anderson S, Bankier AT, Barrell BG, et al. Sequence and organization of the human mitochondrial genome. Nature 1981;290:457-65.
13. D'Erchia AM, Atlante A, Gadaleta G, et al. Tissue-specific mtDNA abundance from exome data and its correlation with mitochondrial transcription, mass and respiratory activity. Mitochondrion 2015;20:13-21.
16. Garrido N, Griparic L, Jokitalo E, Wartiovaara J, van der Bliek AM, Spelbrink JN. Composition and dynamics of human mitochondrial nucleoids. Mol Biol Cell 2003;14:1583-96.
17. Miyakawa I. Organization and dynamics of yeast mitochondrial nucleoids. Proc Jpn Acad Ser B Phys Biol Sci 2017;93:339-59.
18. Brewer LR, Friddle R, Noy A, et al. Packaging of single DNA molecules by the yeast mitochondrial protein Abf2p. Biophys J 2003;85:2519-24.
19. Chakraborty A, Lyonnais S, Battistini F, et al. DNA structure directs positioning of the mitochondrial genome packaging protein Abf2p. Nucleic Acids Res 2017;45:951-67.
20. Kaufman BA, Durisic N, Mativetsky JM, et al. The mitochondrial transcription factor TFAM coordinates the assembly of multiple DNA molecules into nucleoid-like structures. Mol Biol Cell 2007;18:3225-36.
21. Kukat C, Davies KM, Wurm CA, et al. Cross-strand binding of TFAM to a single mtDNA molecule forms the mitochondrial nucleoid. Proc Natl Acad Sci USA 2015;112:11288-93.
22. Ngo HB, Kaiser JT, Chan DC. The mitochondrial transcription and packaging factor Tfam imposes a U-turn on mitochondrial DNA. Nat Struct Mol Biol 2011;18:1290-6.
23. Parisi MA, Xu B, Clayton DA. A human mitochondrial transcriptional activator can functionally replace a yeast mitochondrial HMG-box protein both in vivo and in vitro. Mol Cell Biol 1993;13:1951-61.
24. Richter C, Park JW, Ames BN. Normal oxidative damage to mitochondrial and nuclear DNA is extensive. Proc Natl Acad Sci USA 1988;85:6465-7.
25. Yakes FM, Van Houten B. Mitochondrial DNA damage is more extensive and persists longer than nuclear DNA damage in human cells following oxidative stress. Proc Natl Acad Sci USA 1997;94:514-9.
26. Wang Y, Bogenhagen DF. Human mitochondrial DNA nucleoids are linked to protein folding machinery and metabolic enzymes at the mitochondrial inner membrane. J Biol Chem 2006;281:25791-802.
27. Falkenberg M, Gustafsson CM. Mammalian mitochondrial DNA replication and mechanisms of deletion formation. Crit Rev Biochem Mol Biol 2020;55:509-24.
28. Craven L, Alston CL, Taylor RW, Turnbull DM. Recent advances in mitochondrial disease. Annu Rev Genomics Hum Genet 2017;18:257-75.
29. Lima T, Li TY, Mottis A, Auwerx J. Pleiotropic effects of mitochondria in aging. Nat Aging 2022;2:199-213.
30. Ciccia A, Elledge SJ. The DNA damage response: making it safe to play with knives. Mol Cell 2010;40:179-204.
31. Dua N, Seshadri A, Badrinarayanan A. DarT-mediated mtDNA damage induces dynamic reorganization and selective segregation of mitochondria. J Cell Biol 2022;221:e202205104.
32. Ghosh A, Bhattacharjee S, Chowdhuri SP, et al. SCAN1-TDP1 trapping on mitochondrial DNA promotes mitochondrial dysfunction and mitophagy. Sci Adv 2019;5:eaax9778.
33. Klucnika A, Mu P, Jezek J, et al. REC drives recombination to repair double-strand breaks in animal mtDNA. J Cell Biol 2023;222:e202201137.
34. Lieber T, Jeedigunta SP, Palozzi JM, Lehmann R, Hurd TR. Mitochondrial fragmentation drives selective removal of deleterious mtDNA in the germline. Nature 2019;570:380-4.
35. Palozzi JM, Jeedigunta SP, Minenkova AV, et al. Mitochondrial DNA quality control in the female germline requires a unique programmed mitophagy. Cell Metab 2022;34:1809-23.e6.
36. Tigano M, Vargas DC, Tremblay-Belzile S, Fu Y, Sfeir A. Nuclear sensing of breaks in mitochondrial DNA enhances immune surveillance. Nature 2021;591:477-81.
37. Kazak L, Reyes A, Holt IJ. Minimizing the damage: repair pathways keep mitochondrial DNA intact. Nat Rev Mol Cell Biol 2012;13:659-71.
38. Alexeyev M, Shokolenko I, Wilson G, LeDoux S. The maintenance of mitochondrial DNA integrity-critical analysis and update. Cold Spring Harb Perspect Biol 2013;5:a012641.
39. Omar García-lepe U, Ma Bermúdez-cruz R. Mitochondrial genome maintenance: damage and repair pathways. London: IntechOpen; 2019.
42. Yu R, Lendahl U, Nistér M, Zhao J. Regulation of mammalian mitochondrial dynamics: opportunities and challenges. Front Endocrinol 2020;11:374.
43. Tilokani L, Nagashima S, Paupe V, Prudent J. Mitochondrial dynamics: overview of molecular mechanisms. Essays Biochem 2018;62:341-60.
44. Forslund JME, Pfeiffer A, Stojkovič G, Wanrooij PH, Wanrooij S. The presence of rNTPs decreases the speed of mitochondrial DNA replication. PLoS Genet 2018;14:e1007315.
45. Genga A, Bianchi L, Foury F. A nuclear mutant of Saccharomyces cerevisiae deficient in mitochondrial DNA replication and polymerase activity. J Biol Chem 1986;261:9328-32.
46. Gray H, Wong TW. Purification and identification of subunit structure of the human mitochondrial DNA polymerase. J Biol Chem 1992;267:5835-41.
47. Wanrooij PH, Tran P, Thompson LJ, et al. Elimination of rNMPs from mitochondrial DNA has no effect on its stability. Proc Natl Acad Sci USA 2020;117:14306-13.
48. Clayton DA, Doda JN, Friedberg EC. The absence of a pyrimidine dimer repair mechanism in mammalian mitochondria. Proc Natl Acad Sci USA 1974;71:2777-81.
49. Pascucci B, Versteegh A, van Hoffen A, van Zeeland AA, Mullenders LH, Dogliotti E. DNA repair of UV photoproducts and mutagenesis in human mitochondrial DNA. J Mol Biol 1997;273:417-27.
50. Scheibye-knudsen M, Fang EF, Croteau DL, Wilson DM, Bohr VA. Protecting the mitochondrial powerhouse. Trends Cell Biol 2015;25:158-70.
51. Kennedy SR, Salk JJ, Schmitt MW, Loeb LA. Ultra-sensitive sequencing reveals an age-related increase in somatic mitochondrial mutations that are inconsistent with oxidative damage. PLoS Genet 2013;9:e1003794.
52. Zheng W, Khrapko K, Coller HA, Thilly WG, Copeland WC. Origins of human mitochondrial point mutations as DNA polymerase gamma-mediated errors. Mutat Res 2006;599:11-20.
53. Chatterjee A, Singh KK, Uracil-DNA glycosylase-deficient yeast exhibit a mitochondrial mutator phenotype. Nucleic Acids Res 2001;29:4935-40.
54. Englander EW, Hu Z, Sharma A, Lee HM, Wu ZH, Greeley GH. Rat MYH, a glycosylase for repair of oxidatively damaged DNA, has brain-specific isoforms that localize to neuronal mitochondria. J Neurochem 2002;83:1471-80.
55. Han D, Schomacher L, Schüle KM, et al. NEIL1 and NEIL2 DNA glycosylases protect neural crest development against mitochondrial oxidative stress. Elife 2019;8:e49044.
56. Hu J, de Souza-Pinto NC, Haraguchi K, et al. Repair of formamidopyrimidines in DNA involves different glycosylases: role of the OGG1, NTH1, and NEIL1 enzymes. J Biol Chem 2005;280:40544-51.
57. Mandal SM, Hegde ML, Chatterjee A, et al. Role of human DNA glycosylase Nei-like 2 (NEIL2) and single strand break repair protein polynucleotide kinase 3'-phosphatase in maintenance of mitochondrial genome. J Biol Chem 2012;287:2819-29.
58. Singh KK, Sigala B, Sikder HA, Schwimmer C. Inactivation of Saccharomyces cerevisiae OGG1 DNA repair gene leads to an increased frequency of mitochondrial mutants. Nucleic Acids Res 2001;29:1381-8.
59. You HJ, Swanson RL, Harrington C, et al. Saccharomyces cerevisiae Ntg1p and Ntg2p: broad specificity N-glycosylases for the repair of oxidative DNA damage in the nucleus and mitochondria. Biochemistry 1999;38:11298-306.
60. Alseth I, Eide L, Pirovano M, Rognes T, Seeberg E, Bjørås M. The saccharomyces cerevisiae homologues of endonuclease III from escherichia coli, Ntg1 and Ntg2, are both required for efficient repair of spontaneous and induced oxidative DNA damage in yeast. Mol Cell Biol 1999;19:3779-87.
61. Griffiths LM, Swartzlander D, Meadows KL, Wilkinson KD, Corbett AH, Doetsch PW. Dynamic compartmentalization of base excision repair proteins in response to nuclear and mitochondrial oxidative stress. Mol Cell Biol 2009;29:794-807.
62. Acevedo-Torres K, Fonseca-Williams S, Ayala-Torres S, Torres-Ramos CA. Requirement of the Saccharomyces cerevisiae APN1 gene for the repair of mitochondrial DNA alkylation damage. Environ Mol Mutagen 2009;50:317-27.
63. Duxin JP, Dao B, Martinsson P, et al. Human Dna2 is a nuclear and mitochondrial DNA maintenance protein. Mol Cell Biol 2009;29:4274-82.
64. Liu P, Qian L, Sung JS, et al. Removal of oxidative DNA damage via FEN1-dependent long-patch base excision repair in human cell mitochondria. Mol Cell Biol 2008;28:4975-87.
65. Nagarajan P, Prevost CT, Stein A, Kasimer R, Kalifa L, Sia EA. Roles for the Rad27 flap endonuclease in mitochondrial mutagenesis and double-strand break repair in saccharomyces cerevisiae. Genetics 2017;206:843-57.
66. Zheng L, Zhou M, Guo Z, et al. Human DNA2 is a mitochondrial nuclease/helicase for efficient processing of DNA replication and repair intermediates. Mol Cell 2008;32:325-36.
67. Robson CN, Hickson ID. Isolation of cDNA clones encoding a human apurini/apyrimidinic endonuclease that corects DNA repair and mutagenisis defects in E. coli xth (exonuclease III) mutants. Nucleic Acids Res 1991;19:5519-23.
68. Demple B, Herman T, Chen DS. Cloning and expression of APE, the cDNA encoding the major human apurinic endonuclease: definition of a family of DNA repair enzymes. Proc Natl Acad Sci USA 1991;88:11450-4.
69. Sykora P, Kanno S, Akbari M, et al. DNA polymerase beta participates in mitochondrial DNA repair. Mol Cell Biol 2017;37:e00237-17.
70. Prasad R, Çağlayan M, Dai DP, et al. DNA polymerase β: a missing link of the base excision repair machinery in mammalian mitochondria. DNA Repair 2017;60:77-88.
71. Pinz KG, Bogenhagen DF. Characterization of a catalytically slow AP lyase activity in DNA polymerase gamma and other family a DNA polymerases. J Biol Chem 2000;275:12509-14.
72. Longley MJ, Prasad R, Srivastava DK, Wilson SH, Copeland WC. Identification of 5'-deoxyribose phosphate lyase activity in human DNA polymerase gamma and its role in mitochondrial base excision repair in vitro. Proc Natl Acad Sci USA 1998;95:12244-8.
73. Tahbaz N, Subedi S, Weinfeld M. Role of polynucleotide kinase/phosphatase in mitochondrial DNA repair. Nucleic Acids Res 2012;40:3484-95.
74. Meagher M, Lightowlers RN. The role of TDP1 and APTX in mitochondrial DNA repair. Biochimie 2014;100:121-4.
75. Simsek D, Furda A, Gao Y, et al. Crucial role for DNA ligase III in mitochondria but not in Xrcc1-dependent repair. Nature 2011;471:245-8.
76. Krasich R, Copeland WC. DNA polymerases in the mitochondria: a critical review of the evidence. Front Biosci Landmark Ed 2017;22:692-709.
77. Kalifa L, Sia EA. Analysis of Rev1p and Pol zeta in mitochondrial mutagenesis suggests an alternative pathway of damage tolerance. DNA Repair 2007;6:1732-9.
78. Wisnovsky S, Sack T, Pagliarini DJ, Laposa RR, Kelley SO. DNA polymerase θ increases mutational rates in mitochondrial DNA. ACS Chem Biol 2018;13:900-8.
79. Zhang H, Chatterjee A, Singh KK. Saccharomyces cerevisiae polymerase zeta functions in mitochondria. Genetics 2006;172:2683-8.
80. Prasad R, Longley MJ, Sharief FS, Hou EW, Copeland WC, Wilson SH. Human DNA polymerase theta possesses 5'-dRP lyase activity and functions in single-nucleotide base excision repair in vitro. Nucleic Acids Res 2009;37:1868-77.
81. Prasad R, Poltoratsky V, Hou EW, Wilson SH. Rev1 is a base excision repair enzyme with 5'-deoxyribose phosphate lyase activity. Nucleic Acids Res 2016;44:10824-33.
82. Kasiviswanathan R, Gustafson MA, Copeland WC, Meyer JN. Human mitochondrial DNA polymerase γ exhibits potential for bypass and mutagenesis at UV-induced cyclobutane thymine dimers. J Biol Chem 2012;287:9222-9.
83. Kasiviswanathan R, Minko IG, Lloyd RS, Copeland WC. Translesion synthesis past acrolein-derived DNA adducts by human mitochondrial DNA polymerase γ. J Biol Chem 2013;288:14247-55.
84. Mason PA, Matheson EC, Hall AG, Lightowlers RN. Mismatch repair activity in mammalian mitochondria. Nucleic Acids Res 2003;31:1052-8.
85. Dahal S, Dubey S, Raghavan SC. Homologous recombination-mediated repair of DNA double-strand breaks operates in mammalian mitochondria. Cell Mol Life Sci 2018;75:1641-55.
86. Tadi SK, Sebastian R, Dahal S, Babu RK, Choudhary B, Raghavan SC. Microhomology-mediated end joining is the principal mediator of double-strand break repair during mitochondrial DNA lesions. Mol Biol Cell 2016;27:223-35.
87. Davila JI, Arrieta-Montiel MP, Wamboldt Y, et al. Double-strand break repair processes drive evolution of the mitochondrial genome in Arabidopsis. BMC Biol 2011;9:64.
88. Manchekar M, Scissum-Gunn K, Song D, Khazi F, McLean SL, Nielsen BL. DNA recombination activity in soybean mitochondria. J Mol Biol 2006;356:288-99.
89. Miller-Messmer M, Kühn K, Bichara M, Le Ret M, Imbault P, Gualberto JM. RecA-dependent DNA repair results in increased heteroplasmy of the Arabidopsis mitochondrial genome. Plant Physiol 2012;159:211-26.
90. Shedge V, Arrieta-Montiel M, Christensen AC, Mackenzie SA. Plant mitochondrial recombination surveillance requires unusual RecA and MutS homologs. Plant Cell 2007;19:1251-64.
91. Lakshmipathy U, Campbell C. Double strand break rejoining by mammalian mitochondrial extracts. Nucleic Acids Res 1999;27:1198-204.
92. Thyagarajan B, Padua RA, Campbell C. Mammalian mitochondria possess homologous DNA recombination activity. J Biol Chem 1996;271:27536-43.
93. Srivastava S, Moraes CT. Double-strand breaks of mouse muscle mtDNA promote large deletions similar to multiple mtDNA deletions in humans. Hum Mol Genet 2005;14:893-902.
94. Bacman SR, Williams SL, Moraes CT. Intra- and inter-molecular recombination of mitochondrial DNA after in vivo induction of multiple double-strand breaks. Nucleic Acids Res 2009;37:4218-26.
95. D'Aurelio M, Gajewski CD, Lin MT, et al. Heterologous mitochondrial DNA recombination in human cells. Hum Mol Genet 2004;13:3171-9.
96. Gilkerson RW, Schon EA, Hernandez E, Davidson MM. Mitochondrial nucleoids maintain genetic autonomy but allow for functional complementation. J Cell Biol 2008;181:1117-28.
97. Ma H, O'Farrell PH. Selections that isolate recombinant mitochondrial genomes in animals. Elife 2015;4:e07247.
98. Peeva V, Blei D, Trombly G, et al. Linear mitochondrial DNA is rapidly degraded by components of the replication machinery. Nat Commun 2018;9:1727.
99. Shokolenko IN, Wilson GL, Alexeyev MF. Persistent damage induces mitochondrial DNA degradation. DNA Repair 2013;12:488-99.
100. Bess AS, Crocker TL, Ryde IT, Meyer JN. Mitochondrial dynamics and autophagy aid in removal of persistent mitochondrial DNA damage in Caenorhabditis elegans. Nucleic Acids Res 2012;40:7916-31.
101. Lawarée E, Jankevicius G, Cooper C, Ahel I, Uphoff S, Tang CM. DNA ADP-ribosylation stalls replication and is reversed by RecF-mediated homologous recombination and nucleotide excision repair. Cell Rep 2020;30:1373-84.e4.
102. Alexeyev MF, Venediktova N, Pastukh V, Shokolenko I, Bonilla G, Wilson GL. Selective elimination of mutant mitochondrial genomes as therapeutic strategy for the treatment of NARP and MILS syndromes. Gene Ther 2008;15:516-23.
103. Bacman SR, Williams SL, Hernandez D, Moraes CT. Modulating mtDNA heteroplasmy by mitochondria-targeted restriction endonucleases in a 'differential multiple cleavage-site' model. Gene Ther 2007;14:1309-18.
104. Bacman SR, Williams SL, Pinto M, Peralta S, Moraes CT. Specific elimination of mutant mitochondrial genomes in patient-derived cells by mitoTALENs. Nat Med 2013;19:1111-3.
105. Gammage PA, Rorbach J, Vincent AI, Rebar EJ, Minczuk M. Mitochondrially targeted ZFNs for selective degradation of pathogenic mitochondrial genomes bearing large-scale deletions or point mutations. EMBO Mol Med 2014;6:458-66.
106. Hashimoto M, Bacman SR, Peralta S, et al. MitoTALEN: a general approach to reduce mutant mtDNA loads and restore oxidative phosphorylation function in mitochondrial diseases. Mol Ther 2015;23:1592-9.
107. Kazama T, Okuno M, Watari Y, et al. Curing cytoplasmic male sterility via TALEN-mediated mitochondrial genome editing. Nat Plants 2019;5:722-30.
108. Beurdeley M, Bietz F, Li J, et al. Compact designer TALENs for efficient genome engineering. Nat Commun 2013;4:1762.
109. Pereira CV, Bacman SR, Arguello T, et al. mitoTev-TALE: a monomeric DNA editing enzyme to reduce mutant mitochondrial DNA levels. EMBO Mol Med 2018;10:e8084.
110. Bacman SR, Williams SL, Garcia S, Moraes CT. Organ-specific shifts in mtDNA heteroplasmy following systemic delivery of a mitochondria-targeted restriction endonuclease. Gene Ther 2010;17:713-20.
111. Srivastava S, Moraes CT. Manipulating mitochondrial DNA heteroplasmy by a mitochondrially targeted restriction endonuclease. Hum Mol Genet 2001;10:3093-9.
112. Tanaka M, Borgeld HJ, Zhang J, et al. Gene therapy for mitochondrial disease by delivering restriction endonuclease SmaI into mitochondria. J Biomed Sci 2002;9:534-41.
113. Zekonyte U, Bacman SR, Smith J, et al. Mitochondrial targeted meganuclease as a platform to eliminate mutant mtDNA in vivo. Nat Commun 2021;12:3210.
114. Han M, Vakili MR, Soleymani Abyaneh H, Molavi O, Lai R, Lavasanifar A. Mitochondrial delivery of doxorubicin via triphenylphosphine modification for overcoming drug resistance in MDA-MB-435/DOX cells. Mol Pharm 2014;11:2640-9.
115. Marrache S, Pathak RK, Dhar S. Detouring of cisplatin to access mitochondrial genome for overcoming resistance. Proc Natl Acad Sci USA 2014;111:10444-9.
116. Millard M, Gallagher JD, Olenyuk BZ, Neamati N. A selective mitochondrial-targeted chlorambucil with remarkable cytotoxicity in breast and pancreatic cancers. J Med Chem 2013;56:9170-9.
117. Robb EL, Gawel JM, Aksentijević D, et al. Selective superoxide generation within mitochondria by the targeted redox cycler MitoParaquat. Free Radic Biol Med 2015;89:883-94.
118. Chamberlain GR, Tulumello DV, Kelley SO. Targeted delivery of doxorubicin to mitochondria. ACS Chem Biol 2013;8:1389-95.
119. Fonseca SB, Pereira MP, Mourtada R, et al. Rerouting chlorambucil to mitochondria combats drug deactivation and resistance in cancer cells. Chem Biol 2011;18:445-53.
120. Wisnovsky SP, Wilson JJ, Radford RJ, et al. Targeting mitochondrial DNA with a platinum-based anticancer agent. Chem Biol 2013;20:1323-8.
121. la Loza MC, Wellinger RE. A novel approach for organelle-specific DNA damage targeting reveals different susceptibility of mitochondrial DNA to the anticancer drugs camptothecin and topotecan. Nucleic Acids Res 2009;37:e26.
122. Qian W, Kumar N, Roginskaya V, et al. Chemoptogenetic damage to mitochondria causes rapid telomere dysfunction. Proc Natl Acad Sci USA 2019;116:18435-44.
123. Medeiros TC, Thomas RL, Ghillebert R, Graef M. Autophagy balances mtDNA synthesis and degradation by DNA polymerase POLG during starvation. J Cell Biol 2018;217:1601-11.
124. Nissanka N, Bacman SR, Plastini MJ, Moraes CT. The mitochondrial DNA polymerase gamma degrades linear DNA fragments precluding the formation of deletions. Nat Commun 2018;9:2491.
125. Matic S, Jiang M, Nicholls TJ, et al. Mice lacking the mitochondrial exonuclease MGME1 accumulate mtDNA deletions without developing progeria. Nat Commun 2018;9:1202.
126. Milenkovic D, Sanz-Moreno A, Calzada-Wack J, et al. Mice lacking the mitochondrial exonuclease MGME1 develop inflammatory kidney disease with glomerular dysfunction. PLoS Genet 2022;18:e1010190.
127. Blázquez-Bermejo C, Carreño-Gago L, Molina-Granada D, et al. Increased dNTP pools rescue mtDNA depletion in human POLG-deficient fibroblasts. FASEB J 2019;33:7168-79.
128. Carvajal-Maldonado D, Drogalis Beckham L, Wood RD, Doublié S. When DNA polymerases multitask: functions beyond nucleotidyl transfer. Front Mol Biosci 2021;8:815845.
129. Singh A, Pandey M, Nandakumar D, Raney KD, Yin YW, Patel SS. Excessive excision of correct nucleotides during DNA synthesis explained by replication hurdles. EMBO J 2020;39:e103367.
130. Nissanka N, Moraes CT. Mitochondrial DNA heteroplasmy in disease and targeted nuclease-based therapeutic approaches. EMBO Rep 2020;21:e49612.
131. Chen Z, Wang ZH, Zhang G, et al. Mitochondrial DNA segregation and replication restrict the transmission of detrimental mutation. J Cell Biol 2020;219:e201905160.
132. Hill JH, Chen Z, Xu H. Selective propagation of functional mitochondrial DNA during oogenesis restricts the transmission of a deleterious mitochondrial variant. Nat Genet 2014;46:389-92.
133. Zhang Y, Wang ZH, Liu Y, et al. PINK1 inhibits local protein synthesis to limit transmission of deleterious mitochondrial DNA mutations. Mol Cell 2019;73:1127-37.e5.
134. Legros F, Lombès A, Frachon P, Rojo M. Mitochondrial fusion in human cells is efficient, requires the inner membrane potential, and is mediated by mitofusins. Mol Biol Cell 2002;13:4343-54.
135. Song Z, Chen H, Fiket M, Alexander C, Chan DC. OPA1 processing controls mitochondrial fusion and is regulated by mRNA splicing, membrane potential, and Yme1L. J Cell Biol 2007;178:749-55.
136. Twig G, Elorza A, Molina AJ, et al. Fission and selective fusion govern mitochondrial segregation and elimination by autophagy. EMBO J 2008;27:433-46.
137. Hori A, Yoshida M, Ling F. Mitochondrial fusion increases the mitochondrial DNA copy number in budding yeast. Genes Cells 2011;16:527-44.
138. Silva Ramos E, Motori E, Brüser C, et al. Mitochondrial fusion is required for regulation of mitochondrial DNA replication. PLoS Genet 2019;15:e1008085.
139. Jakubke C, Roussou R, Maiser A, et al. Cristae-dependent quality control of the mitochondrial genome. Sci Adv 2021;7:eabi8886.
140. Campbell G, Krishnan KJ, Deschauer M, Taylor RW, Turnbull DM. Dissecting the mechanisms underlying the accumulation of mitochondrial DNA deletions in human skeletal muscle. Hum Mol Genet 2014;23:4612-20.
141. Gitschlag BL, Kirby CS, Samuels DC, Gangula RD, Mallal SA, Patel MR. Homeostatic responses regulate selfish mitochondrial genome dynamics in C. elegans. Cell Metab 2016;24:91-103.
142. Lin YF, Schulz AM, Pellegrino MW, Lu Y, Shaham S, Haynes CM. Maintenance and propagation of a deleterious mitochondrial genome by the mitochondrial unfolded protein response. Nature 2016;533:416-9.
143. Yang Q, Liu P, Anderson NS, et al. LONP-1 and ATFS-1 sustain deleterious heteroplasmy by promoting mtDNA replication in dysfunctional mitochondria. Nat Cell Biol 2022;24:181-93.
144. Brüser C, Keller-Findeisen J, Jakobs S. The TFAM-to-mtDNA ratio defines inner-cellular nucleoid populations with distinct activity levels. Cell Rep 2021;37:110000.
146. Roca-Portoles A, Tait SWG. Mitochondrial quality control: from molecule to organelle. Cell Mol Life Sci 2021;78:3853-66.
147. Tatsuta T, Langer T. Quality control of mitochondria: protection against neurodegeneration and ageing. EMBO J 2008;27:306-14.
148. Kleele T, Rey T, Winter J, et al. Distinct fission signatures predict mitochondrial degradation or biogenesis. Nature 2021;593:435-9.
149. König T, Nolte H, Aaltonen MJ, et al. MIROs and DRP1 drive mitochondrial-derived vesicle biogenesis and promote quality control. Nat Cell Biol 2021;23:1271-86.
150. Li X, Straub J, Medeiros TC, et al. Mitochondria shed their outer membrane in response to infection-induced stress. Science 2022;375:eabi4343.
152. Ahier A, Dai CY, Kirmes I, et al. PINK1 and parkin shape the organism-wide distribution of a deleterious mitochondrial genome. Cell Rep 2021;35:109203.
153. Kandul NP, Zhang T, Hay BA, Guo M. Selective removal of deletion-bearing mitochondrial DNA in heteroplasmic drosophila. Nat Commun 2016;7:13100.
154. Meshnik L, Bar-Yaacov D, Kasztan D, et al. Mutant C. elegans mitofusin leads to selective removal of mtDNA heteroplasmic deletions across generations to maintain fitness. BMC Biol 2022;20:40.
155. Böckler S, Chelius X, Hock N, et al. Fusion, fission, and transport control asymmetric inheritance of mitochondria and protein aggregates. J Cell Biol 2017;216:2481-98.
156. Hinge A, He J, Bartram J, et al. Asymmetrically segregated mitochondria provide cellular memory of hematopoietic stem cell replicative history and drive HSC attrition. Cell Stem Cell 2020;26:420-30.e6.
157. Katajisto P, Döhla J, Chaffer CL, et al. Stem cells. Asymmetric apportioning of aged mitochondria between daughter cells is required for stemness. Science 2015;348:340-3.
158. Blackford AN, Jackson SP. ATM, ATR, and DNA-PK: the trinity at the heart of the DNA damage response. Mol Cell 2017;66:801-17.
159. Pizzul P, Casari E, Gnugnoli M, Rinaldi C, Corallo F, Longhese MP. The DNA damage checkpoint: a tale from budding yeast. Front Genet 2022;13:995163.
160. Hilton BA, Li Z, Musich PR, et al. ATR plays a direct antiapoptotic role at mitochondria, which is regulated by prolyl isomerase Pin1. Mol Cell 2015;60:35-46.
161. Taylor SD, Zhang H, Eaton JS, et al. The conserved Mec1/Rad53 nuclear checkpoint pathway regulates mitochondrial DNA copy number in Saccharomyces cerevisiae. Mol Biol Cell 2005;16:3010-8.
162. Koczor CA, Shokolenko IN, Boyd AK, Balk SP, Wilson GL, LeDoux SP. Mitochondrial DNA damage initiates a cell cycle arrest by a Chk2-associated mechanism in mammalian cells. J Biol Chem 2009;284:36191-201.
163. Fu Y, Kanshin E, Ueberheide B, Sfeir A. MtDNA breaks compromise mitochondrial membrane ultrastructure and trigger an integrated stress response. bioRxiv 2022. Available from: https://www.biorxiv.org/content/10.1101/2022.10.01.510431v1 [Last accessed on 12 Jun 2023].
164. Dhir A, Dhir S, Borowski LS, et al. Mitochondrial double-stranded RNA triggers antiviral signalling in humans. Nature 2018;560:238-42.
165. Moya GE, Rivera PD, Dittenhafer-Reed KE. Evidence for the role of mitochondrial DNA Release in the inflammatory response in neurological disorders. Int J Mol Sci 2021;22:7030.
166. Newman LE, Tadepalle N, Novak SW, et al. Endosomal removal and disposal of dysfunctional, immunostimulatory mitochondrial DNA. bioRxiv 2022:10.12.511955.
167. Sen A, Kallabis S, Gaedke F, et al. Mitochondrial membrane proteins and VPS35 orchestrate selective removal of mtDNA. Nat Commun 2022;13:6704.
168. Sprenger HG, MacVicar T, Bahat A, et al. Cellular pyrimidine imbalance triggers mitochondrial DNA-dependent innate immunity. Nat Metab 2021;3:636-50.
169. Arguello T, Peralta S, Antonicka H, et al. ATAD3A has a scaffolding role regulating mitochondria inner membrane structure and protein assembly. Cell Rep 2021;37:110139.
170. He J, Mao CC, Reyes A, et al. The AAA+ protein ATAD3 has displacement loop binding properties and is involved in mitochondrial nucleoid organization. J Cell Biol 2007;176:141-6.
171. Peralta S, Goffart S, Williams SL, et al. ATAD3 controls mitochondrial cristae structure in mouse muscle, influencing mtDNA replication and cholesterol levels. J Cell Sci 2018;131:jcs217075.
172. He B, Yu H, Liu S, et al. Mitochondrial cristae architecture protects against mtDNA release and inflammation. Cell Rep 2022;41:111774.
173. Riley JS, Quarato G, Cloix C, et al. Mitochondrial inner membrane permeabilisation enables mtDNA release during apoptosis. EMBO J 2018;37:e99238.
174. Colina-Tenorio L, Horten P, Pfanner N, Rampelt H. Shaping the mitochondrial inner membrane in health and disease. J Intern Med 2020;287:645-64.
175. Klecker T, Westermann B. Pathways shaping the mitochondrial inner membrane. Open Biol 2021;11:210238.
176. Bohr VA. Repair of oxidative DNA damage in nuclear and mitochondrial DNA, and some changes with aging in mammalian cells. Free Radic Biol Med 2002;32:804-12.
Cite This Article
Export citation file: BibTeX | RIS
OAE Style
Dua N, Badrinarayanan A. Mechanisms regulating mitochondrial DNA quality control. J Transl Genet Genom 2023;7:110-25. http://dx.doi.org/10.20517/jtgg.2023.08
AMA Style
Dua N, Badrinarayanan A. Mechanisms regulating mitochondrial DNA quality control. Journal of Translational Genetics and Genomics. 2023; 7(2): 110-25. http://dx.doi.org/10.20517/jtgg.2023.08
Chicago/Turabian Style
Dua, Nitish, Anjana Badrinarayanan. 2023. "Mechanisms regulating mitochondrial DNA quality control" Journal of Translational Genetics and Genomics. 7, no.2: 110-25. http://dx.doi.org/10.20517/jtgg.2023.08
ACS Style
Dua, N.; Badrinarayanan A. Mechanisms regulating mitochondrial DNA quality control. J. Transl. Genet. Genom. 2023, 7, 110-25. http://dx.doi.org/10.20517/jtgg.2023.08
About This Article
Copyright
Data & Comments
Data
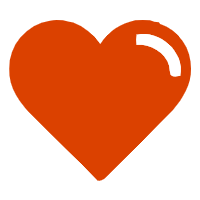

Comments
Comments must be written in English. Spam, offensive content, impersonation, and private information will not be permitted. If any comment is reported and identified as inappropriate content by OAE staff, the comment will be removed without notice. If you have any queries or need any help, please contact us at support@oaepublish.com.