Stable ultrathin lithium metal anode enabled by self-adapting electrochemical regulating strategy
Abstract
Ultrathin lithium (Li) metal foils with controllable capacity could realize high-specific-energy batteries; however, the pulverization of Li metal foils due to its extreme volume change results in rapid active Li loss and capacity fading. Here, we report a strategy to stabilize ultrathin Li metal anode via in-situ transferring Li from ultrathin Li foil into a well-designed three-dimensional gradient host during a cycling process. A three-dimensional carbon fiber with gradient distribution of Ag nanoparticles is placed on the ultrathin Li foil in advance and acts as a Li reservoir, guiding Li deposition into its interior and thus alleviating the volume change of ultrathin Li foil anodes. Hence, a high reversibility of Li metal is achieved and Li pulverization is suppressed, which can be witnessed by a long cyclic life in the symmetric cells. The proposed method offers a versatile and facile approach for protecting ultrathin Li metal anodes, which will boost their commercial application process.
Keywords
INTRODUCTION
Metallic lithium (Li) is a potential anode material for rechargeable batteries due to its high theoretical specific capacity (3,860 mAh·g-1) and low redox potential (-3.04 V vs. the standard hydrogen electrode)[1-4]. Ultrathin Li metal foils matching with commercial lithium transition metal oxide cathodes are expected to achieve high-specific-energy rechargeable batteries[5-8]. However, the high chemical reactivity of Li metal makes metallic Li spontaneously react with non-aqueous electrolytes to form solid electrolyte interphase (SEI) layers, contributing to low Li plating/stripping efficiency and low Li utilization rate[9-11]. In addition, because of the conversion reaction and the hostless Li plating/stripping characteristic, the Li metal anode suffers from infinite volume changes during cycling, which are highly responsible for the pulverization of Li metal, active Li loss, and the blocking of ionic transport[12,13].
Constructing a well-designed SEI layer on ultrathin Li foil has proved effective in suppressing Li/electrolyte interfacial parasitic reaction and alleviating Li dendrite penetration[14-18]. Thus, numerous artificial SEI layers, including organic layers[19-21], inorganic layers[22-24], and organic-inorganic hybrid layers[25,26], are designed and adopted to protect the ultrathin Li metal anodes. However, the constant volume fluctuation from the intrinsic hostless characteristic of Li metal anodes challenges the structure stability in the long running. Studies have shown that encapsulating active Li metal into a host is efficacious in addressing the volume change issue[27-29]. Therefore, various micro/nanostructured hosts have been widely studied, including three-dimensional (3D) Cu current collectors[30,31], reduced graphene oxide[32-35], carbon fibers[36-39], porous carbon granules[40-43], and so on. Combined with the high lithiophilic sites, the plating/stripping behaviors and the cycling performances of the composite metallic Li anode have seen great improvement. The common method that loads Li into 3D hosts can be clarified into electrochemical plating and melting strategy. The electrochemical pre-plating strategy can strictly control the amount of Li; however, this method is usually needed to assemble, disassemble and re-assemble the batteries, which is highly time-consuming and high-cost[44]. The melting strategy can avoid the complex processes, but the as-prepared composite anodes generally demonstrate a low Li utilization[45]. Therefore, it is imperative to explore a facile, highly efficient and inexpensive method to protect ultrathin Li metal anodes.
Herein, a self-adapting electrochemical regulating strategy, that is, in-situ self-migrating of Li metal from ultrathin Li foil into a well-designed 3D host by electrochemical cycling, is used to extend the cycling life of ultrathin Li anode. The key-enabling technique is to design a lithiophilic host with gradient distribution of Ag nanoparticles. Then, this well-designed host is directly placed on a commercial ultrathin Li metal foil. During the cycling process, Li metal gradually migrates into the prestaging host with the aid of Ag nanoparticles, nucleates on the Ag nanoparticles, and grows from bottom to up in the host, which helps stabilize the ultrathin Li anode volume and avoid internal short-circuit arising from the electrical connection of Li dendrite between Li anodes and cathodes [Scheme 1]. Hence, the hybrid Li anode featuring dendrite-free morphology demonstrates high reversibility and superior cycling performance. The hybrid Li anode also demonstrates its application potential by presenting a long lifespan of 180 cycles at a very low
Scheme 1. Schematics of Li/CC-Ag hybrid anode before and after cycling. (A) CC-Ag stacked with ultrathin lithium before Li/CC-Ag cycle; (B) After a few turns of Li/CC-Ag cycles, ultrathin lithium is gradually transferred to CC-Ag; (C) Complete transfer of ultrathin lithium to CC-Ag after Li/CC-Ag cycles. CC: Carbon cotton.
EXPERIMENTAL
The absorbent cotton (AC) was pressed into thin sheets with a hot press under a pressure of 15 MPa at
Materials characterizations
Field emission scanning electron microscopy (Sigma 500) was carried out to characterize the microscopic morphology of the electrode materials, while an Energy dispersive spectrometer (EDS) was combined to qualitatively analyze the local elemental distribution of the samples. To observe the electrode morphology clearly, the cycled electrode was washed with 1,2-dimethoxyethane (DME) solvent repeatedly. The component of the CC and CC-Ag electrode was characterized by X-ray diffraction (XRD), which was conducted by using a Bruker D8 Advance with Cu Kα-rays (λ = 1.54 Å) as the target material at a current
Electrochemical tests
Electrochemical tests were performed by assembling CR2032 type coin cells in an argon-filled glove box and were tested on a NEWARE system. Celgard 2400 served as the separator, and 1 M LiTFSI/DOL-DME
RESULTS AND DISCUSSION
A simple method, involving carbonization of natural cotton followed by thermal evaporation of Ag nanoparticles, was developed to prepare a 3D carbon host with gradient lithiophilicity (CC-Ag) [Figure 1A]. The CC derived from the carbonized cotton exhibits a 3D interconnected network distributed of C and N elements [Supplementary Figure 1]. After a thermal evaporation process, abundant nanoparticles with an average diameter of 50-60 nm are homogeneously distributed on the CC
Figure 1. (A) Optical images of cotton, AC, CC, and CC-Ag; (B) XRD results of CC and CC-Ag; (C) SEM cross-sectional image of CC-Ag and corresponding elemental mappings; (D) Linear elemental mapping of Ag element along the CC-Ag. AC: Absorbent cotton; CC: carbon cotton; XRD: X-ray diffraction; SEM: scanning electron microscopy.
Due to the inevitable parasitic reaction and large electrode volume change, ultrathin Li foil usually suffers from the obsession of pulverization and inactive Li gathering during long-term cycling. Putting a 3D host with lithiophilic gradient structure on the ultrathin Li is expected to realize the self-migration of Li metal from ultrathin Li foil into the host upon cycling and form 3D composite Li metal anodes and, therefore, address the above issues. The self-adapting regulating ability of the CC-Ag is elucidated by the schematic diagrams [Figure 2A and B] and the ex-situ scanning electron microscopy (SEM) images [Figure 2C-F]. Prior to the morphological characterization, Li||Li symmetric cells were prepared by putting the CC-Ag electrode containing Ag side on the ultrathin Li foil while a Li foil serves as the counter electrode. Due to the good affinity of Ag to Li and the lithiophilic difference between the bottom and top of the CC, Li tends to deposit on the bottom of the CC-Ag [Figure 2C and D]. Upon the cycling, more Li can be observed on the bottom of the CC-Ag, indicating ultrathin Li gradually self-migrates into the interconnected network of CC-Ag from bottom to up [Figure 2E and F, Supplementary Figure 5]. Due to the high porosity of the
Figure 2. Schematic diagram of (A) Li/CC-Ag and (B) Li/CC during cycling; (C) Top and (D) bottom SEM images of Li/CC-Ag anode after the first cycle; (E) Top and (F) bottom SEM images of Li/CC-Ag anode after 50 cycles; (G) Top and (H) bottom SEM images of Li/CC anode after the first cycle; (I) Top and (J) bottom SEM images of Li/CC anode after 50 cycles. These electrodes were cycled at 0.5 mA·cm-2 with a capacity of 2 mAh·cm-2. CC: Carbon cotton; SEM: scanning electron microscopy.
The reversibility and electrochemical activity of Li from the ultrathin Li foil are verified by the electrochemical performances. The Li/CC-Ag delivers a high Li Coulombic efficiency of 99.5% at
Figure 3. (A) Voltage vs. time of Li/CC-Ag, Li/CC and Li foil-based symmetric cells and (B) the enlarged polarization voltage comparison for the three electrodes; (C) Comparison of the EIS of the Li/CC-Ag and Li/CC symmetric cells; (D) EIS of the Li/CC-Ag symmetric cells after 240 cycles; (E) Voltage vs. time of Li/CC-Ag and Li/CC symmetric cells at 1 mA·cm-2 with a capacity of 8 mAh·cm-2; (F) Voltage vs. time of Li/CC-Ag and Li/CC symmetric cells performed at increasing current densities and capacities. CC: Carbon cotton; EIS: electrochemical impedance spectrum.
Mossy Li or dendritic Li is easy to detach from the bulk Li foil and form electrically isolated dead Li at high areal capacity or current density. Hence, the symmetric cells were cycled at 1 mA·cm-2 with a capacity of
Full cells in combination with a high areal capacity LFP cathode (> 1.7 mAh·cm-2) and Li/CC-Ag or Li/CC anode were assembled to verify the application possibility of the self-transferring of Li metal from ultrathin Li foil into a 3D host. The Li/CC-Ag|LFP full cell anode delivers the highest reversible capacity of
Figure 4. (A) Voltage vs. capacity of Li/CC-Ag||LFP, Li/CC||LFP, and Li foil||LFP full cells at 0.5 C after 50 cycles and (B) their long-time cycling of these three full cells; (C) The 50th Nyquist impedance plots of Li/CC-Ag||LFP and Li/CC||LFP full cells at 0.5 C; (D) Capacity vs. cycle number of LFP full cell with the Li/CC-Ag at 1.0 C; (E) Capacity vs. cycle number of the high capacity LFP full cell with the Li/CC-Ag at 0.5 C; (F) Capacity vs. cycle number of Li/CC-Ag||LFP full cell at a low N/P ratio at 0.5 C. CC: Carbon cotton; LFP: LiFePO4.
CONCLUSIONS
In conclusion, we reported a practical method to utilize ultrathin Li foil by directly placing Ag-modified 3D carbon fiber on its surface. The well-designed 3D carbon fiber features gradient lithiophilic sites. During electrochemical cycling, the Li resources gradually self-transferred into the 3D carbon fiber from bottom to up, ensuring the high reversibility and electrode volume stability. As a result, the hybrid anode containing ultrathin Li metal and 3D carbon fiber exhibits a Li utilization rate of about 83% and a long cycling performance of over 2,000 h. Full cell assembled by this hybrid anode delivers up to 180 stable cycles under a low N/P ratio (≤ 1.5). These results show good potential for the self-adapting electrochemical regulating strategy in prolonging the lifespan of ultrathin Li metal anode for high-specific-energy Li metal batteries.
DECLARATIONS
Authors’ contributions
Data curation, formal analysis, investigation, writing - original draft: Zeng SY
Data curation, formal analysis, investigation: Wang WL
Writing - review and editing: Li D
Supervision, writing - review and editing: Yang C
Supervision, funding acquisition, writing - review and editing: Zheng ZJ
Availability of data and materials
The data supporting our work can be found in the Supplementary Materials.
Financial support and sponsorship
This work was supported by the National Natural Science Foundation of China (grant No. 22179036).
Conflicts of interest
All authors declared that there are no conflicts of interest.
Ethical approval and consent to participate
Not applicable.
Consent for publication
Not applicable.
Copyright
© The Author(s) 2024.
Supplementary Materials
REFERENCES
1. Lin D, Liu Y, Cui Y. Reviving the lithium metal anode for high-energy batteries. Nat Nanotechnol 2017;12:194-206.
2. Zhang Y, Zuo T, Popovic J, et al. Towards better Li metal anodes: challenges and strategies. Mater Today 2020;33:56-74.
3. Lu G, Nai J, Luan D, Tao X, Lou XWD. Surface engineering toward stable lithium metal anodes. Sci Adv 2023;9:eadf1550.
4. Zhang W, Zhang F, Liu S, et al. Regulating the reduction reaction pathways via manipulating the solvation shell and donor number of the solvent in Li-CO2 chemistry. Proc Natl Acad Sci U S A 2023;120:e2219692120.
5. Shi P, Cheng XB, Li T, et al. Electrochemical diagram of an ultrathin lithium metal anode in pouch cells. Adv Mater 2019;31:e1902785.
6. Chen H, Yang Y, Boyle DT, et al. Free-standing ultrathin lithium metal-graphene oxide host foils with controllable thickness for lithium batteries. Nat Energy 2021;6:790-8.
7. Jin D, Roh Y, Jo T, Ryou M, Lee H, Lee YM. Robust cycling of ultrathin Li metal enabled by nitrate-preplanted Li powder composite. Adv Energy Mater 2021;11:2003769.
8. Li T, Shi P, Zhang R, Liu H, Cheng X, Zhang Q. Dendrite-free sandwiched ultrathin lithium metal anode with even lithium plating and stripping behavior. Nano Res 2019;12:2224-9.
9. Cheng XB, Zhang R, Zhao CZ, Zhang Q. Toward safe lithium metal anode in rechargeable batteries: a review. Chem Rev 2017;117:10403-73.
10. Gao RM, Yang H, Wang CY, Ye H, Cao FF, Guo ZP. Fatigue-resistant interfacial layer for safe lithium metal batteries. Angew Chem Int Ed Engl 2021;60:25508-13.
11. Cheng B, Zheng ZJ, Yin X. Recent progress on the air-stable battery materials for solid-state lithium metal batteries. Adv Sci 2024;11:e2307726.
12. Ye H, Zhang Y, Yin YX, Cao FF, Guo YG. An outlook on low-volume-change lithium metal anodes for long-life batteries. ACS Cent Sci 2020;6:661-71.
13. Wang C, Yang C, Zheng Z. Toward practical high-energy and high-power lithium battery anodes: present and future. Adv Sci 2022;9:e2105213.
14. Liu Y, Tao X, Wang Y, et al. Self-assembled monolayers direct a LiF-rich interphase toward long-life lithium metal batteries. Science 2022;375:739-45.
15. Wu H, Jia H, Wang C, Zhang J, Xu W. Recent progress in understanding solid electrolyte interphase on lithium metal anodes. Adv Energy Mater 2021;11:2003092.
16. Lee J, Choi SH, Qutaish H, et al. Structurally stabilized lithium-metal anode via surface chemistry engineering. Energy Storage Mater 2021;37:315-24.
17. Li Q, Zhang J, Zeng Y, et al. Lithium reduction reaction for interfacial regulation of lithium metal anode. Chem Commun 2022;58:2597-611.
18. Cheng Y, Wang Z, Chen J, et al. Catalytic chemistry derived artificial solid electrolyte interphase for stable lithium metal anodes working at 20 mA cm-2 and 20 mAh cm-2. Angew Chem Int Ed Engl 2023;62:e202305723.
19. Wang Y, Wang Z, Zhao L, et al. Lithium metal electrode with increased air stability and robust solid electrolyte interphase realized by silane coupling agent modification. Adv Mater 2021;33:e2008133.
20. Kang D, Sardar S, Zhang R, et al. In-situ organic SEI layer for dendrite-free lithium metal anode. Energy Storage Mater 2020;27:69-77.
21. Hou LP, Li Y, Li Z, et al. Electrolyte design for improving mechanical stability of solid electrolyte interphase in lithium-sulfur batteries. Angew Chem Int Ed Engl 2023;62:e202305466.
22. Wang Z, Wang Y, Wu C, Pang WK, Mao J, Guo Z. Constructing nitrided interfaces for stabilizing Li metal electrodes in liquid electrolytes. Chem Sci 2021;12:8945-66.
23. Chen C, Liang Q, Wang G, Liu D, Xiong X. Grain-boundary-rich artificial SEI layer for high-rate lithium metal anodes. Adv Funct Mater 2022;32:2107249.
24. Liu D, Xiong X, Liang Q, Wu X, Fu H. An inorganic-rich SEI induced by LiNO3 additive for a stable lithium metal anode in carbonate electrolyte. Chem Commun 2021;57:9232-5.
25. Xiao J, Zhai P, Wei Y, et al. In-situ formed protecting layer from organic/inorganic concrete for dendrite-free lithium metal anodes. Nano Lett 2020;20:3911-7.
26. Zhang QK, Zhang XQ, Wan J, et al. Homogeneous and mechanically stable solid-electrolyte interphase enabled by trioxane-modulated electrolytes for lithium metal batteries. Nat Energy 2023;8:725-35.
27. Feng Y, Zhang C, Li B, Xiong S, Song J. Low-volume-change, dendrite-free lithium metal anodes enabled by lithophilic 3D matrix with LiF-enriched surface. J Mater Chem A 2019;7:6090-8.
28. Zheng ZJ, Su Q, Zhang Q, et al. Low volume change composite lithium metal anodes. Nano Energy 2019;64:103910.
29. Jiang Q, Xiong P, Liu J, et al. A redox-active 2D metal-organic framework for efficient lithium storage with extraordinary high capacity. Angew Chem Int Ed Engl 2020;59:5273-7.
30. Ye H, Zheng ZJ, Yao HR, et al. Guiding uniform Li plating/stripping through lithium-aluminum alloying medium for long-life Li metal batteries. Angew Chem Int Ed Engl 2019;58:1094-9.
31. Chen J, Zhao J, Lei L, et al. Dynamic intelligent Cu current collectors for ultrastable lithium metal anodes. Nano Lett 2020;20:3403-10.
32. Yang T, Li L, Wu F, Chen R. A soft lithiophilic graphene aerogel for stable lithium metal anode. Adv Funct Mater 2020;30:2002013.
33. Liu S, Wang A, Li Q, et al. Crumpled graphene balls stabilized dendrite-free lithium metal anodes. Joule 2018;2:184-93.
34. Feng X, Wu H, Gao B, Świętosławski M, He X, Zhang Q. Lithiophilic N-doped carbon bowls induced Li deposition in layered graphene film for advanced lithium metal batteries. Nano Res 2022;15:352-60.
35. Xiong P, Yin H, Chen Z, et al. Thiourea-based polyimide/RGO composite cathode: a comprehensive study of storage mechanism with alkali metal ions. Sci China Mater 2020;63:1929-38.
36. Zeng SY, Wang CY, Yang C, Zheng ZJ. Limited lithium loading promises improved lithium-metal anodes in interface-modified 3D matrixes. ACS Appl Mater Interfaces 2022;14:41065-71.
37. Lee Y, Cho K, Lee S, et al. Construction of hierarchical surface on carbon fiber paper for lithium metal batteries with superior stability. Adv Energy Mater 2023;13:2203770.
38. Chen H, Li M, Li C, et al. Electrospun carbon nanofibers for lithium metal anodes: Progress and perspectives. Chin Chem Lett 2022;33:141-52.
39. Lu Q, Jie Y, Meng X, et al. Carbon materials for stable Li metal anodes: challenges, solutions, and outlook. Carbon Energy 2021;3:957-75.
40. Yuan H, Nai J, Tian H, et al. An ultrastable lithium metal anode enabled by designed metal fluoride spansules. Sci Adv 2020;6:eaaz3112.
41. Huang W, Liu S, Yu R, Zhou L, Liu Z, Mai L. Single-atom lithiophilic sites confined within ordered porous carbon for ultrastable lithium metal anodes. Energy Environ Mater 2023;6:e12466.
42. Lu C, Tian M, Wei C, Zhou J, Rümmeli MH, Yang R. Synergized N, P dual-doped 3D carbon host derived from filter paper for durable lithium metal anodes. J Colloid Interface Sci 2023;632:1-10.
43. Feng YS, Li YN, Wang P, Guo ZP, Cao FF, Ye H. Work-function-induced interfacial electron/ion transport in carbon hosts toward dendrite-free lithium metal anodes. Angew Chem Int Ed Engl 2023;62:e202310132.
44. Zheng ZJ, Ye H, Guo ZP. Recent progress in designing stable composite lithium anodes with improved wettability. Adv Sci 2020;7:2002212.
Cite This Article
Export citation file: BibTeX | RIS
OAE Style
Zeng SY, Wang WL, Li D, Yang C, Zheng ZJ. Stable ultrathin lithium metal anode enabled by self-adapting electrochemical regulating strategy. Energy Mater 2024;4:400029. http://dx.doi.org/10.20517/energymater.2023.93
AMA Style
Zeng SY, Wang WL, Li D, Yang C, Zheng ZJ. Stable ultrathin lithium metal anode enabled by self-adapting electrochemical regulating strategy. Energy Materials. 2024; 4(2): 400029. http://dx.doi.org/10.20517/energymater.2023.93
Chicago/Turabian Style
Zeng, Si-Yuan, Wen-Long Wang, Deyuan Li, Chunpeng Yang, Zi-Jian Zheng. 2024. "Stable ultrathin lithium metal anode enabled by self-adapting electrochemical regulating strategy" Energy Materials. 4, no.2: 400029. http://dx.doi.org/10.20517/energymater.2023.93
ACS Style
Zeng, S.Y.; Wang W.L.; Li D.; Yang C.; Zheng Z.J. Stable ultrathin lithium metal anode enabled by self-adapting electrochemical regulating strategy. Energy Mater. 2024, 4, 400029. http://dx.doi.org/10.20517/energymater.2023.93
About This Article
Copyright
Data & Comments
Data
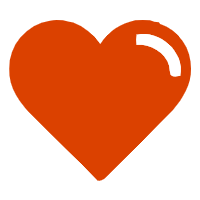

Comments
Comments must be written in English. Spam, offensive content, impersonation, and private information will not be permitted. If any comment is reported and identified as inappropriate content by OAE staff, the comment will be removed without notice. If you have any queries or need any help, please contact us at support@oaepublish.com.