Carbon footprint of shifting cultivation landscapes: current knowledge, assumptions and data gaps
Abstract
Shifting cultivation, a rotational land use system widely practiced in tropical regions, is often blamed for contributing to climate change due to the perceived association with deforestation and resulting greenhouse gas (GHG) emissions from slash and burn activities. This concern is often used to justify the implementation of national land use policies aiming at restricting or eradicating shifting cultivation while encouraging alternative land use systems. However, the contribution of shifting cultivation to global climate change is questionable. This study summarizes the available - and unavailable - data and knowledge required to calculate the carbon footprint (CFP) of shifting cultivation and highlights the methodological challenges and problematic assumptions that lie therein. Data on carbon stocks of fallows are found to be incomplete with large unexplained variation in the relationship between fallow age and carbon stocks of above- and belowground vegetation, and studies from Africa are under-represented. Knowledge of GHG emissions during burning is limited and associated with unsubstantiated assumptions on combustion completeness and emissions factors that represent important sources of uncertainty. Data on the global extent of shifting cultivation is coarse, and spatially explicit data on the rotation intensity of these systems is unavailable, thus hindering any upscaling of CFP calculations. Finally, it is concluded that the contribution of shifting cultivation to deforestation remains unclear, with remote sensing-based studies likely overestimating the scale of this due to methodological flaws. This review calls for caution when interpreting data on GHG emissions from shifting cultivation and suggests ways of addressing the identified data gaps.
Keywords
INTRODUCTION
Land use and land use change contribute around 23% of net anthropogenic greenhouse gas emissions (GHG)[1]. However, through sustainable management, land use systems can also act as GHG sinks through the enhancement of carbon (C) sequestration in soil and vegetation, leading to a reduced carbon footprint (CFP) (BOX 1)[1-4].
As climate change and its effects become more prevalent, land use systems are increasingly assessed, and compared, in relation to their CFP in a bid to find solutions or alternatives that would reduce global warming. However, land use systems should not only be evaluated based on their CFP, but also on their contribution to food security and local livelihoods[2]. This is especially relevant for the Global South, where food insecurity issues are prevalent (and likely to worsen due to the impacts of climate change)[5].
BOX 1: The Carbon Footprint concept:
Shifting cultivation is one of the oldest forms of agriculture and millions of people across the tropics depend fully or partly on the system for their livelihood and food security[8,9]. Due to the central practice of slashing and burning vegetation to prepare fields, the system is often portrayed as a source of GHG emissions and a driver of deforestation[10,11]. Critics, therefore, argue that to mitigate climate change and limit deforestation and forest degradation, shifting cultivation must be eradicated or reduced[10,12,13]. Accordingly, many countries in the Global South (particularly in Asia) have included eradication or "stabilization" of shifting cultivation in their strategies to reduce GHG emissions and adopted policies aimed at limiting the land area used for shifting cultivation or prohibiting the practice altogether while instead, promoting intensified agriculture or plantations (e.g., rubber, oil palm) that are perceived as sustainable alternatives[9,14,15]. However, empirical data on C emissions from shifting cultivation, and the promoted alternatives, are limited and much of the information that serves as the basis for such land use policies is inconsistent or has been misconstrued[16-18]. Land use policies are increasingly guided by desires to reduce the climate change impacts of land use systems, and should not uncritically be based on incorrect assumptions about shifting cultivation as a major source of GHG emissions. Therefore, efforts to accurately quantify the CFP of the system should be made.
There is no standardized protocol for quantifying the CFP of land use systems, but the process requires a land cover classification allowing for the units of a given system to be mapped (without double-counting or gaps), and data on the C stocks and GHG emissions of the different units. This data serves as a basis for an area-based calculation of the time-averaged C stocks and GHG emissions of a given system[7]. However, the lack of methodological standardization leads to highly variable CFPs with no consensus on which reference system to use or how a system’s boundary is defined[19]. While mapping the CFP of monocropping systems with clear boundaries and easily quantifiable inputs and outputs is relatively straightforward, the opposite is true for shifting cultivation systems that (among other things) are characterized by unclear boundaries and C stocks that are spatially heterogeneous and temporally dynamic.
Rather than attempting to quantify the CFP of shifting cultivation, this review summarizes the available - and unavailable - data and knowledge required for such a calculation and highlights the methodological challenges and problematic assumptions that lie therein. Moreover, it is hoped this review will raise awareness of not only the caution necessary when interpreting data on the CFP of shifting cultivation, but the need for more accurate data, and the development of methodological approaches.
Shifting cultivation
Shifting cultivation takes diverse forms, but it is essentially a rotational land use system that relies on the shifting of cultivation between different plots and extended fallow periods. Here we apply the definition suggested by Mertz et al., which broadly captures most systems in Asia, Africa, Latin America, and the Pacific[20]:
“[Shifting cultivation is] a land use system that employs a natural or improved fallow phase, which is longer than the cultivation phase of annual crops, sufficiently long to be dominated by woody vegetation, and cleared by means of fire.”
This rotational nature of shifting cultivation creates a mosaic landscape of patches of active plots (i.e., cultivated), fallows of varying age and - in many cases - areas of primary forest (e.g., forests on hilltops, community forests, and sacred forests)[21,22]. The management of traditional shifting cultivation systems is based on in-depth knowledge of the local environment (e.g., about soils, climate, plants, and fire management)[23,24]. Most shifting cultivation systems are focused on subsistence production, and the availability of products that can be harvested (or collected) both from the cultivated fields and the regrowing fallows throughout the year is important for the food security of local communities[25-27].
It is important to distinguish between the rotational clearing and burning of fallows used in shifting cultivation systems, and the permanent clearing of forests for pioneer agriculture which is also done by slashing and burning. The latter is often confused with shifting cultivation, although it is associated with fundamentally different land use systems and - as opposed to shifting cultivation - leads to net deforestation.
METHODOLOGY
As this review covers shifting cultivation and (i) carbon storage/stocks; and (ii) associated GHG emissions, two separate literature searches were conducted in the Web of Science Core Collection.
Shifting cultivation and carbon storage
For the identification of papers on shifting cultivation and carbon storage (i.e., biomass and soil), we took the point of departure in papers identified in a study on ecosystem services in secondary forests in shifting cultivation by Mertz et al. (please see this paper for a detailed description of the search strings and the methodology)[28]. Based on this collection of papers, we identified 78 papers reporting either on shifting cultivation and soil carbon storage/stocks or on shifting cultivation and carbon storage/stocks in above- or belowground biomass. We updated the search to identify recently published papers which only resulted in the identification of one additional paper.
Data were then extracted from the papers and aggregated according to region and rotation intensity or forest age to give an impression of the magnitude and range of values found in the current literature. The following categories for rotation intensities and forests were used: annual cultivation, fallows (1-5 years;
Shifting cultivation and GHG emissions
To identify papers on shifting cultivation and GHG emissions, we used the following search terms:
“Shifting cultivation” or “shifting agriculture” or swidden or “slash and burn” or “slash and burn” and “greenhouse gas emission*” or “emission factor*” or “biomass burning”
This search identified 111 papers that were screened at the abstract level, and the vast majority were found to be irrelevant as the search terms were only used in the general framing of the studies. We, therefore, used snowballing to identify more relevant papers.
Data regarding the quantification of GHG emissions of shifting cultivation systems were extracted from the papers along with the associated assumptions that we compare and discuss.
THE CARBON FOOTPRINT OF SHIFTING CULTIVATION
Calculation of the CFP of shifting cultivation requires data on the sizes of the various carbon stocks of the systems and knowledge of the amount of GHGs released during burning. Furthermore, knowledge about the rotation intensity - i.e., the relation between the number of years under fallow and the number of years under cultivation - is needed along with data on the extent of the system. Finally, data on the contribution of shifting cultivation to net deforestation - along with knowledge of the C stocks of the affected forests - is required.
The following section provides an overview of the available data and knowledge required to calculate the CPF of shifting cultivation and discusses data gaps along with associated assumptions and challenges that lie therein.
Carbon stocks in shifting cultivation systems
In shifting cultivation systems, C is mainly stored in the above- and belowground biomass and in the soil, while smaller amounts may be stored in dead biomass (typically as unburned tree trunks). In this section, the factors controlling the C dynamics of these pools are described, and the range in C stocks (above- and belowground biomass, and soil C of shifting cultivation at various rotation intensities and of forests of various ages measured to date are summarized.
Above- and belowground biomass
On a broad scale, the growth rates of the fallow vegetation are controlled by climate - especially rainfall[29]. However, within a certain climatic regime, vegetation growth rates may be strongly affected by management practices. Clearing of large plots and intensive burns may reduce the quantities of seeds available for fallow recolonization, while repeated cultivation of the same plot may deplete soil fertility, resulting in reduced fallow growth rates, and a reduction of the amount of C stored in the aboveground biomass under a given rotation intensity[29,30]. Young fallows are dominated by pioneer species, many of which are woody N2-fixing leguminous species. In some systems, such species are actively promoted, which may speed up rates of biomass recovery and nutrient accumulation[31].
Fallows are often used as sources of wood (e.g., for construction, fuel, etc.), which will lead to a reduction of C in the aboveground biomass. Alternatively, in some cases, useful tree species are left standing and protected from fire, which will contribute to C storage[23,32].
Accordingly, the size of the aboveground C stocks of shifting cultivation systems is context-specific, and the variability is high - even within the same climatic zones[16]. This is also evident from the (non-exhaustive) overview of estimates of aboveground carbon reported by studies of shifting cultivation in Africa, Asia, and Latin America [Table 1].
Above- and belowground carbon stocks (Mg C ha-1) of shifting cultivation at different rotation intensities and of forests of various ages
Region | Land cover (years) | Carbon stock (Min-Max; Mg C ha-1) | |
Aboveground biomass | Belowground biomass | ||
Latin America | |||
Fallow (1-5) | 5-70 | 3.3-11.5 | |
Fallow (6-10) | 11.8-158 | 7.6 | |
Fallow (11-15) | 15-152 | 15 | |
Fallow (16-20) | 37.5-118.2 | 10.5 | |
Fallow (21-30) | 46.5-196.6 | No data | |
Old growth (31-40) | 60-304.9 | 14-17 | |
Old growth (41-60) | 269.8-460.5 | No data | |
Primary forest | 105-335.6 | 11-23.8 | |
Africa | |||
Fallow (1-5) | No data | No data | |
Fallow (6-10) | No data | 2.5-3.5 | |
Fallow (11-15) | 7-11.5 | 5 | |
Fallow (16-20) | 2.5-3.5 | 3.5 | |
Fallow (21-30) | 13.5 | No data | |
Old growth (31-40) | 5 | No data | |
Old growth (41-60) | 15 | No data | |
Primary forest | 3.5 | 9 | |
Asia | |||
Fallow (1-5) | 2.1-33.5 | 0.5-11 | |
Fallow (6-10) | 6.2-35.7 | 2.0-6.5 | |
Fallow (11-15) | 25.0-43.3 | 6.2-10 | |
Fallow (16-20) | 40.0-48.5 | 8.4-13 | |
Fallow (21-30) | 41.6-59.5 | No data | |
Old growth (31-40) | 134.1 | No data | |
Old growth (41-60) | No data | 35-112 | |
Primary forest | No data | No data |
Apart from the large variation in the aboveground biomass stocks within the relatively narrow ranges of fallow ages, it is worth noting the very limited number of studies from Africa which, to the best of our knowledge, represents the available studies from this continent.
The aboveground biomass values reported by the studies from Africa are low compared to values from the other continents which partly reflects that all the African studies are from areas with less than 900 mm of precipitation, while studies from Asia and Latin America are from areas with average precipitation of
Knowledge of the C storage in the belowground biomass (roots) of shifting cultivation systems is notoriously limited and very few studies provide direct measurements of this C pool[17,33]. In the absence of allometric equations developed for secondary vegetation (fallows), most estimates of belowground C stocks in shifting cultivation systems are based on root:shoot ratios derived from studies of undisturbed forests, and only two studies[33,34] are based on direct measurements. As in the case of aboveground biomass, the number of studies from Africa is very limited [Table 1].
Soil carbon
During the fallow period, the regenerating vegetation provides inputs of biomass (i.e., litterfall, root turnover, and root exudates) to the soil. A fraction of this biomass is incorporated as “new” C into the SOC pool, thereby replenishing the carbon lost during cultivation[35,36]. The degree to which the soil C pool is replenished is dependent on the biomass productivity of the fallow vegetation and if the soil carbon sequestration capacity has been reached[36-38]. The conversion from fallow to cultivation leads to a decline in SOC stocks due to the reduced biomass input and higher decomposition rates caused by topsoil exposure and disturbances[39,40].
Theoretically, if the fallow length is sufficient to restore system productivity and the rotations are consistent, a dynamic equilibrium between soil C gains and losses will be reached[41]. This theoretical minimum fallow length, however, has been difficult to pinpoint because studies document inconsistent fallow length effects on soil C levels; positive, negative and neutral effects of fallow length on soil C levels have been found[36,42-48]. This is due to not only the high spatio-temporal variation found within shifting cultivation systems as previously discussed, but prior rotations and farmer preference also play an influential role. For example, Lawrence et al. found a positive correlation between the number of prior rotations (fallow-cultivation cycles) and SOC stocks[43]. This is likely attributed to the underestimated input of decaying roots from slashed fallow vegetation and the addition of incompletely combusted particles from the frequent slash and burn activity[47,49]. Farmers have also been known to preferentially select plots with more fertile soils, cultivating them more intensively, which would have a similar effect as fertile soils are likely to have higher C content than less fertile soils[40,50]. A study by Bruun et al. accounted for farmers’ preferential selection of plots in the sampling scheme and, nonetheless, found that fields cultivated more intensively (i.e., shorter fallows) have higher SOC stocks than extensively managed fields[47]. This finding was explained by the more frequent and recurrent additions of C from roots due to the shorter crop-fallow rotations.
Given such variation, it has been difficult to assign values to the soil C changes that occur within shifting cultivation and when it transitions to alternate land uses. A non-exhaustive overview of studies quantifying the soil C stocks (0-20 cm and 0-30 cm) in shifting cultivation systems in Latin America, Africa and Asia highlights the variable range that can be found [Table 2]. From the table, it is apparent that there is no consistent pattern in the effect of annual cultivation and fallow on soil C stocks [Table 2]. Furthermore, there is a lack of data for some fallow categories, especially from Africa, where shifting cultivation is an important land use system[22,51]. This is due to the limited number of studies from the region, inconsistencies in sampling methods (e.g., sampling depth) and in the way results are reported (e.g., soil C stocks vs soil C concentration) [Table 2]. Hence, it has proven to be extremely difficult to confidently characterize or quantify the soil C gains and losses from shifting cultivation; any such values should be used as guides and are not definitive.
Soil carbon stocks (Mg C ha-1) of shifting cultivation at different rotation intensities and of forests of various ages
Region | Land cover (years) | Carbon stock (Min-Max; Mg C ha-1) | |
0-20 cm | 0-30 cm | ||
Latin America | |||
Annual cultivation | 37.1 | 48.6 | |
Fallow (1-5) | 54.9 | 25.6 | |
Fallow (6-10) | 35.0-53.3 | 15.1-18.6 | |
Fallow (11-15) | 40.6 | 23.4-52.3 | |
Fallow (16-20) | 27.0-50.3 | 20.6 | |
Fallow (21-30) | No data | 22.9-23.3 | |
Old growth (31-40) | 20.0-68.4 | 17.0-34.7 | |
Old growth (41-60) | No data | 24.4-27.1 | |
Primary forest | 27.8-67.0 | 20.4-25.7 | |
Africa | |||
Annual cultivation | 35.3-46.0 | 9.0-40.3 | |
Fallow (1-5) | 53.9-75.6 | 11.0-53.0 | |
Fallow (6-10) | 53.5-72.5 | 9.5-81.5 | |
Fallow (11-15) | 52.5-56.6 | 54.7 | |
Fallow (16-20) | 60.6 | 56.5-59.4 | |
Fallow (21-30) | No data | 14.5 | |
Old growth (31-40) | No data | 53.0 | |
Old growth (41-60) | No data | No data | |
Primary forest | 53.3-62.0 | 16.9-67.5 | |
Asia | |||
Annual cultivation | 58.0 | No data | |
Fallow (1-5) | 42.1-85.2 | 50.6-119.7 | |
Fallow (6-10) | 22.2-79.8 | 33.6-134.3 | |
Fallow (11-15) | 39.5-92.0 | 50.7-65.9 | |
Fallow (16-20) | 49.8 | 66.6-72.3 | |
Fallow (21-30) | 39.7-42.0 | 55.7-56.4 | |
Old growth (31-40) | 47.0-49.1 | 61.0-65.2 | |
Old growth (41-60) | 34.4-35.9 | 46.4-48.0 | |
Primary forest | 125.1 | 85.0-153.4 |
Time-averaged carbon stocks
The C storage potential of rotational land use systems is not determined by the C stock at any point in time, but by the average amount of C stored in the system during its entire rotation - referred to as the “time-averaged C stock”[40,52]. In the case of shifting cultivation, the time-averaged C stock is dependent on the rotation intensity of the system and calculated as the sum of the C stocks in the biomass and soil each year of the rotation divided by the length of the rotation[18]. However, very few studies provide data allowing for a calculation of the time-averaged C stocks of shifting cultivation and most studies merely report a snapshot of C stocks of fallows of certain ages[28].
GHG emissions from shifting cultivation
The slash and burn activities associated with shifting cultivation are often categorized under the broader category of fires leading to “deforestation”; however, this classification is misleading as shifting cultivation does not lead to permanent deforestation.
The rotational nature of shifting cultivation means that a system with a stable rotation intensity will not be a net emitter of CO2 over the long term[11,53] because the CO2 released during the burning of fallows will be counterbalanced by the CO2 captured by the regrowing fallows elsewhere in the system. This will change if the rotation intensity decreases (i.e., longer fallows), which will result in a net CO2 uptake, while an increase in rotation intensity (i.e., shorter fallows) will lead to a net CO2 emission.
GHG emissions from burning
Shifting cultivation systems are likely to be net emitters of non-CO2 GHGs such as methane (CH4), carbon monoxide (CO), nitrous oxide (N2O) and nitrogen oxides (NOx) due to the slashing and burning of biomass[53]. Quantifying the release of GHGs from the burning of fallow biomass relies on many assumptions related to: (i) the amount of biomass per area unit (a function of fallow length and biomass productivity); (ii) combustion completeness (influenced by biophysical factors such as vegetation type, moisture content and weather conditions during the time of the burn); (iii) emission factors (especially influenced by the type of biomass and temperature); and (iv) local management practices (e.g., protection of large trees or other desired woody species from fire, collection of biomass beforehand for fuel or building material, etc.)[53,54].
As discussed (and shown in Table 1), amounts of C in aboveground biomass of fallows are very variable and difficult to predict solely based on fallow age. The combustion completeness used in studies to calculate the emissions from shifting cultivation is also highly variable; Silva et al. show combustion completeness ranges from 20.1%-87.5%, with an average of 40.6%, while Lauk & Erb use an assumed value of 53.8%, based on Fearnside[11,53,55]. A literature review by van Leeuwen et al. found a combustion completeness average of 47% (range 30%-64%) for shifting cultivation, but this conclusion is based on only two studies (Zambia and India) and results are thus inconclusive[54]. Emission factors, defined as the amount of compounds released per unit of dry biomass consumed (g kg-1), are a great source of uncertainty in model estimates of GHG emissions[53,54]. While ground measurements of species and biome-specific emission factors are available, the high spatial and temporal variability makes large-scale applications of GHG emission calculations challenging[56]. None of the identified studies of GHG emissions from shifting cultivation have included local management practices in estimations of the aboveground biomass burned, even though this is recognized as a potentially important factor[53].
Studies have attempted to estimate the emissions of GHGs from shifting cultivation with variable results. Silva et al. used spatial information derived from satellite imagery to estimate global CO2 emissions from shifting cultivation to be 741 Tg yr-1, with the largest GHG emissions coming from America, followed by Asia and then Africa [Table 3][53]. Previous estimates of the global CO2 emissions from shifting cultivation range from 950 Tg CO2 yr-1[11] to 2,764 Tg CO2 yr-1[57], highlighting the high degree of variability in the approach to quantifying emissions and the importance of the associated assumptions. These include assumptions about the geographical extent of shifting cultivation, rotation intensity, combustion completeness and emission factors, and local management practices. Hence, the reported GHG emissions should be considered as place-based scenarios that bring awareness to the sources and magnitude of uncertainty (as described above).
Regional GHG emission estimates from shifting cultivation in America, Africa, and Asia. Mean values (and standard deviations) are based on the Global Land Cover 2000 Map and adapted from Silva et al.[53]
CO2 (Tg yr-1) | CO (Tg yr-1) | CH4 (Gg yr-1) | NOx (Gg yr-1) | N2O (Gg yr-1) | |
America | 295 (197) | 18 (13) | 1,197 (892) | 410 (389) | 36 (33) |
Africa | 205 (139) | 13 (9) | 832 (626) | 285 (272) | 25 (23) |
Asia | 241 (132) | 15 (9) | 979 (618) | 335 (282) | 30 (24) |
The geographical extent of shifting cultivation
The spatio-temporal diversity and dynamic nature of shifting cultivation make it notoriously challenging to map, and the mosaic landscapes produced by shifting cultivation are often not captured by remote sensing-based land cover and land use classifications[22]. Moreover, as shifting cultivation falls between conventional agricultural and forestry land cover categories, the system is often not included in national land cover assessments, but rather referred to as “idle” or “degraded” land[28,58]. These methodological and political challenges mean that shifting cultivation is normally absent from land cover maps and that the knowledge of the extent of the system remains limited.
Efforts have been made to obtain a broad estimation of the global extent, but to date, the global area under shifting cultivation is based on few and varying estimates, with the most recent, and likely most accurate, being 280 Mha[22]. This study relied on a combination of expert information and visual inspection of very high-resolution satellite imagery to detect the specific spatio-temporal pattern of shifting cultivation at a one-degree cell resolution worldwide[22]. The study found signs of shifting cultivation in 62% of the investigated one-degree cells in the humid and sub-humid tropics[22]. The majority of these cells were found in Latin America (41%), followed by Africa (37%)[22].
Moreover, it was found that the area under shifting cultivation in Southeast Asia has declined since the early 2000s[22] - a finding that is corroborated by results of a case-study-based meta-analysis[59]. Trends in the extent of the system in Africa and Latin America vary by region and are both positive and negative but much more subtle than in the case of Southeast Asia[22,59].
Rotation intensity and system boundaries
There is no spatially explicit data on the rotation intensity of shifting cultivation systems at the global (or regional) scale, but a meta-analysis of land cover transformations observed reductions of fallow lengths in the majority of the reviewed cases[59]. It should also be noted that there are often large differences in the rotation intensities within the same area (at the village level) as this (among other things) is influenced by biophysical factors and household characteristics, such as soil types, availability of land, labor and other assets[47,60]. Hett et al. developed a land use intensity map for a 26 × 26 km area of Northern Laos with promising results, but the methodology used in their study is difficult to upscale and no attempts to do so have been made[21].
Another challenge related to the spatial complexity of shifting cultivation systems is the fact that the system is rarely found in isolation, but rather as a component of a landscape that also contains other land use types that may (or may not) be considered a part of the shifting cultivation system. Such landscapes are, for example, common in Borneo (Sarawak and Kalimantan), where rubber plots, pepper gardens and stands of fruit trees are often integrated into the shifting cultivation cycle[61-64] [Figure 1]. This implies that it is often notoriously difficult to draw meaningful boundaries around a shifting cultivation area.
Figure 1. Shifting cultivation area of the longhouse, Nanga Tapih, Betong Division, Sarawak, Malaysia. From a livelihood perspective, all the specified land uses are considered part of the shifting cultivation system. The pepper fields are likely to eventually be left fallow and included in the shifting cultivation rotation system, and the plots that are currently used for rubber or fruit trees are likely to eventually be slashed and burned and used for rice at the end of the life cycle of the trees (or if other factors encourage this conversion). Photo: Rob Cramb.
Shifting cultivation vs. deforestation
Shifting cultivation is often blamed for causing deforestation, but it is important to note that rotational shifting cultivation - meaning cycling between plots within the same landscape - does not constitute deforestation[16,65,66]. Deforestation only occurs when plots are established in forest areas that have not been cultivated before. Shifting cultivation systems under intensification, meaning increased frequency of cropping under shorter fallows, will experience a net tree cover loss that translates into reduced C storage at the landscape level.
In a widely cited global assessment of drivers of deforestation, Curtis et al. identify shifting cultivation as the dominant driver of observed forest loss in Africa, causing 93% ± 3% of this loss[67]. In comparison,
Interestingly, using the data derived from Curtis et al., a global study on C fluxes concludes that shifting cultivation is a net C sink[67,68]. This conclusion is based on several assumptions: (i) all shifting cultivation systems are managed under shortened fallows whereby the vegetation does not fully recover; (ii) the calculation of emission factors is based on a total loss of C in the above- and belowground, deadwood and litter pools, and a soil C loss determined by the soil type and ecological zone; and (iii) removal factors are calculated by linking available - and often limited - data on growth rates to the ecological zone, forest type, and age. Furthermore, any forest C sink within the same 10 km grid cell as a forest disturbance event attributed to shifting cultivation by Curtis et al. was attributed to shifting cultivation[67]. The fact that shifting cultivation can be seen both as a dominant driver of forest loss and a C net sink reflects the methodological challenges and the problematic assumptions made in remote-sensing-based GHG flux estimations or land use change that involves shifting cultivation.
CONCLUSION AND PERSPECTIVES
As discussed in this paper, quantification of the CFP of shifting cultivation is hindered by limited data availability and methodological challenges related to measuring the extent and intensity of shifting cultivation. This research documents that:
(1) Data on C stocks is incomplete
There are gaps in our understanding of C storage in belowground biomass of fallows and of C transfer from this pool to the soil when fallow vegetation is slashed. There are also large unexplained variations in the relationships between fallow age and C stocks of above- and belowground biomass of fallows within similar climate conditions. This could indicate large differences in local management practices, gaps in our understanding of C accumulation in fallows, or may be a sign of methodological inconsistencies between studies. Empirical data on C stocks of African shifting cultivation systems are particularly scarce and data allowing for calculation of time-averaged C stocks of the system are generally missing.
(2) Knowledge of GHG emissions during burning is limited
The number of studies of GHG emissions associated with burning in shifting cultivation is very limited and the few available studies employ numerous assumptions. Important sources of uncertainty are related to unsubstantiated assumptions regarding combustion completeness and emissions factors.
(3) Knowledge of the intensity and extent of shifting cultivation systems is limited
Spatially explicit data on the rotation intensity of shifting cultivation systems are unavailable, and data on the extent of the system (regardless of the intensity with which it is practiced) is prohibitively coarse, which hinders any upscaling of CFP calculations.
(4) The contribution of shifting cultivation to deforestation remains unclear
Rotational shifting cultivation does not lead to deforestation; however, systems undergoing intensification (i.e., an increased frequency of cropping under shorter fallows) do result in a net loss of tree cover. The methodological challenges and numerous assumptions in GHG flux measurements are obvious, considering that shifting cultivation has been identified simultaneously as a cause of forest loss and a net C sink.
Recommendations
Given these large gaps in data, we did not attempt to calculate the CFP of shifting cultivation, but based on the identified shortcomings, we advocate for comprehensive research focused at the local level to advance our understanding of the complex dynamics occurring within shifting cultivation systems to reduce the uncertainties that currently exist due to data scarcity and the associated assumptions.
This research should focus on the C dynamics of shifting cultivation as more data on these are clearly needed - especially from Africa. We also encourage the development of coupled biomass-soil C models for well-studied shifting cultivation systems. Such models would make it possible to assess the time-averaged C stocks of systems at various rotation intensities, which is an important input for assessing the CFP.
It was beyond the scope of this study to review the methodologies and context-specific characteristics of the identified studies of C pools of shifting cultivation, but such an analysis is called for to improve our understanding of the large differences observed in the dataset.
The lack of data on GHG emissions during burning remains a challenge and is probably best addressed through field experimental studies at the plot level integrated with model simulations and ground-airborne-satellite observations[69].
To address the lack of data on the extent and rotation intensity of shifting cultivation, efforts should be made to develop remote sensing methods that are able to capture the dynamic nature of shifting cultivation at the landscape level. Such methods are currently under development, and with recent advances in Earth observation systems providing images at both high spatial and temporal resolution coupled with the emergence of deep learning approaches in artificial intelligence, there is a large potential for improvements.
Perspectives
It was beyond the scope of this paper to discuss the CFP of the land use types that are currently replacing shifting cultivation in many areas - often with the encouragement of land use policies claiming to reduce the climate change impacts of land use systems. A recent pan-tropical meta-study concluded that shifting cultivation is mainly replaced by monocrop tree plantations, annual crops, permanent agroforestry systems and regrowing secondary vegetation[66], and while positive environmental outcomes prevail for transitions to permanent agroforestry and regrowing secondary vegetation, most of the transitions resulted in negative outcomes for the environment (including a decrease in carbon storage)[9,28,59,66,70].
Moreover, this review calls for caution when comparing CFPs of land use systems with no (or very limited) use of external inputs like shifting cultivation. Land use systems with a high reliance on external inputs to maintain production will have additional attributable GHG emissions such as from the production of fertilizers, pesticides, or transport - emissions which are not included in calculations of their CFPs[7]. Hence, comparing the CFPs of shifting cultivation and most alternative production systems (perhaps with the exception of permanent agroforestry) is inherently problematic. Furthermore, the smallholder farming communities practicing shifting cultivation often have low CFPs as practitioners produce the majority of their own food, live in relatively isolated areas lacking electricity, and have a low level of consumption of material goods.
A valid calculation of the CFP of shifting cultivation is not possible at this time for reasons discussed in this paper. Nonetheless, there is evidence to suggest that the C dynamics in shifting cultivation systems result in lower emissions compared to other, far more dominant systems. This, alone, is grounds for advancing shifting cultivation onto scientific and political agendas for sustainable land use. Any communication about the climate change impacts of the system should be done carefully, as such can have real repercussions on the livelihoods of its often resource-poor and socially vulnerable practitioners.
DECLARATIONS
AcknowledgementsThe graphical abstract was made by Louise Hedegaard Madsen.
Authors’ contributionsConceived the study: Bruun TB
Contributed equally to the development of the methodology, the data analysis and interpretation, and the writing and reviewing of the manuscript: Bruun TB, Hepp CM
Availability of data and materialsNot applicable.
Financial support and sponsorshipHepp CM is funded by the Research Institute for Humanity and Nature (FairFrontiers, Project Number 14200149).
Conflicts of interestBoth authors declared that there are no conflicts of interest.
Ethical approval and consent to participateNot applicable.
Consent for publicationNot applicable.
Copyright© The Author(s) 2023.
REFERENCES
1. IPCC. Climate change and land: an IPCC special report on climate change, desertification, land degradation, sustainable land management, food security, and greenhouse gas fluxes in terrestrial ecosystems; 2019. Available from: https://www.ipcc.ch/srccl/ [Last accessed on 1 June 2023].
2. IPBES. The global assessment report of the intergovernmental science-policy platform on biodiversity and ecosystem services; 2019.
4. Udawatta RP, Walter D, Jose S. Carbon sequestration by forests and agroforests: a reality check for the United States. Carbon Footprints 2023;2:2.
5. Salmon G, Teufel N, Baltenweck I, van Wijk M, Claessens L, Marshall K. Trade-offs in livestock development at farm level: different actors with different objectives. Glob Food Sec 2018;17:103-12.
6. Wiedmann T, Minx JC. A definition of carbon footprint improving the environmental performance of australian construction projects view project AI for evidence synthesis in climate change view project; 2008. Available from: https://www.novapublishers.com/catalog/product_info.php?products_id=5999 [Last accessed on 1 June 2023].
7. van Noordwijk M, Pham TT, Leimona B, et al. Carbon footprints, informed consumer decisions and shifts towards responsible agriculture, forestry, and other land uses? Carbon Footprints 2022;1:4.
8. Mertz O, Leisz SJ, Heinimann A, et al. Who counts? Demography of Swidden cultivators in southeast Asia. Hum Ecol 2009;37:281-9.
9. Dressler WH, Wilson D, Clendenning J, et al. The impact of swidden decline on livelihoods and ecosystem services in Southeast Asia: a review of the evidence from 1990 to 2015. Ambio 2017;46:291-310.
10. Borah JR, Evans KL, Edwards DP. Quantifying carbon stocks in shifting cultivation landscapes under divergent management scenarios relevant to REDD. Ecol Appl 2018;28:1581-93.
11. Fearnside PM. Global warming and tropical land-use change: greenhouse gas emissions from biomass burning, decomposition and soils in forest conversion, shifting cultivation and secondary vegetation. Clim Chang 2000;46:115-58.
12. Lestrelin G, Giordano M. Upland development policy, livelihood change and land degradation: interactions from a Laotian village. Land Degrad Dev 2007;18:55-76.
13. Filho AA, Adams C, Murrieta RSS. The impacts of shifting cultivation on tropical forest soil: a review. Bol Mus Para Emílio Goeldi Ciênc hum 2013;8:693-727.
14. Mertz O, Bruun TB. Shifting cultivation policies in Southeast Asia: a need to work with, rather than against, smallholder farmers. In: Cairns M, editor. Shifting cultivation policies: Balancing environmental and social sustainability. UK: CABI; 2017. pp. 27-42.
15. Bauernschuster S, Pichler M, Ingalls M, Thongmanivong S, Gingrich S. Discursive and biophysical dimensions of land sparing policies in Laos: implications for greenhouse gas emissions and food security. Land Use Policy 2022;120:106293.
16. Ziegler AD, Phelps J, Yuen JQ, et al. Carbon outcomes of major land-cover transitions in SE Asia: great uncertainties and REDD+ policy implications. Glob Chang Biol 2012;18:3087-99.
17. Yuen JQ, Fung T, Ziegler AD. Review of allometric equations for major land covers in SE Asia: Uncertainty and implications for above- and below-ground carbon estimates. For Ecol Manag 2016;360:323-40.
18. Bruun TB, Berry N, de Neergaard A, Xaphokahme P, Mcnicol I, Ryan CM. Long rotation swidden systems maintain higher carbon stocks than rubber plantations. Agric Ecosyst Environ 2018;256:239-49.
20. Mertz O, Padoch C, Fox J, et al. Swidden change in Southeast Asia: understanding causes and consequences. Hum Ecol 2009;37:259-64.
21. Hett C, Castella J, Heinimann A, Messerli P, Pfund J. A landscape mosaics approach for characterizing swidden systems from a REDD+ perspective. Appl Geogr 2012;32:608-18.
22. Heinimann A, Mertz O, Frolking S, et al. A global view of shifting cultivation: Recent, current, and future extent. PLoS One 2017;12:e0184479.
23. Carmenta R, Vermeylen S, Parry L, Barlow J. Shifting cultivation and fire policy: insights from the Brazilian amazon. Hum Ecol 2013;41:603-14.
24. Dressler WH, Smith W, Kull CA, Carmenta R, Pulhin JM. Recalibrating burdens of blame: anti-swidden politics and green governance in the philippine uplands. Geoforum 2021;124:348-59.
25. FAO, IWGIA, AIPP. Shifting cultivation, livelihood and food security: new and old challenges for indigenous peoples in Asia; 2015. Available from: http://www.iwgia.org/iwgia_files_publications_files/0694_AIPPShifting_cultivation_livelihoodfood_security.pdf [Last accessed on 1 June 2023].
26. Delang CO, Weiyi X, Brooke B, Chun KP. The effect of fallow period length on the abundance and diversity of usable plant assemblages in shifting cultivation system (Swidden agriculture) in Northern Laos. Pol J Ecol 2016;64:350-6.
27. Rasmussen LV, Mertz O, Christensen AE, Danielsen F, Dawson N, Xaydongvanh P. A combination of methods needed to assess the actual use of provisioning ecosystem services. Ecosyst Serv 2016;17:75-86.
28. Mertz O, Bruun TB, Jepsen MR, et al. Ecosystem service provision by secondary forests in shifting cultivation areas remains poorly understood. Hum Ecol 2021;49:271-83.
29. Szott LT, Palm CA, Buresh RJ. Ecosystem fertility and fallow function in the humid and subhumid tropics. Agrofor Syst 1999;47:163-96.
30. Lawrence D. Biomass accumulation after 10-200 years of shifting cultivation in Bornean rain forest. Ecology 2005;86:26-33.
32. Mertz O, Birch-thomsen T, Elberling B, et al. Changes in shifting cultivation systems on small Pacific islands. Geogr J 2012;178:175-87.
33. Mcnicol IM, Berry NJ, Bruun TB, et al. Development of allometric models for above and belowground biomass in swidden cultivation fallows of Northern Laos. For Ecol Manag 2015;357:104-16.
34. Sommer R, Denich M, Vlek PL. Carbon storage and root penetration in deep soils under small-farmer land-use systems in the Eastern Amazon region, Brazil. Plant Soil 2000;219:231-41.
35. Palm CA, Swift MJ, Woomer PL. Soil biological dynamics in slash and burn agriculture. Agric Ecosyst Environ 1996;58:61-74.
36. Hepp CM, de Neergaard A, Bruun TB. Short-term fallow in extensive upland shifting cultivation systems of Northern Lao PDR: Its role in soil fertility restoration. Land Degrad Dev 2018;29:2911-9.
37. Hassink J. The capacity of soils to preserve organic C and N by their association with clay and silt particles. Plant Soil 1997;191:77-87.
38. Gulde S, Chung H, Amelung W, Chang C, Six J. Soil carbon saturation controls labile and stable carbon pool dynamics. Soil Sci Soc Am J 2008;72:605-12.
39. van Noordwijk M, Cerri C, Woomer PL, Nugroho K, Bernoux M. Soil carbon dynamics in the humid tropical forest zone. Geoderma 1997;79:187-225.
40. Bruun TB, de Neergaard A, Lawrence D, Ziegler AD. Environmental consequences of the demise in swidden cultivation in southeast asia: carbon storage and soil quality. Hum Ecol 2009;37:375-88.
41. Murty D, Kirschbaum MUF, Mcmurtrie RE, Mcgilvray H. Does conversion of forest to agricultural land change soil carbon and nitrogen? A review of the literature. Glob Chang Biol 2002;8:105-23.
42. Roder W, Phengchanh S, Keoboulapha B. Relationships between soil, fallow period, weeds and rice yield in slash and burn systems of Laos. Plant Soil 1995;176:27-36.
43. Lawrence D, Suma V, Mogea JP. Change in species composition with repeated shifting cultivation: limited role of soil nutrients. Ecol Appl 2005;15:1952-67.
44. Chan N, Takeda S, Suzuki R, Yamamoto S. Assessment of biomass recovery and soil carbon storage of fallow forests after swidden cultivation in the Bago Mountains, Myanmar. New Forests 2016;47:565-85.
45. Lungmuana, Choudhury BU, Saha S, et al. Impact of postburn jhum agriculture on soil carbon pools in the north-eastern Himalayan region of India. Soil Res 2018;56:615-22.
46. Terefe B, Kim D. Shifting cultivation maintains but its conversion to mono-cropping decreases soil carbon and nitrogen stocks compared to natural forest in Western Ethiopia. Plant Soil 2020;453:105-17.
47. Bruun TB, Ryan CM, de Neergaard A, Berry NJ. Soil organic carbon stocks maintained despite intensification of shifting cultivation. Geoderma 2021;388:114804.
48. Arunrat N, Sereenonchai S, Hatano R. Effects of fire on soil organic carbon, soil total nitrogen, and soil properties under rotational shifting cultivation in northern Thailand. J Environ Manag 2022;302:113978.
49. Kotto-same J, Woomer PL, Appolinaire M, Louis Z. Carbon dynamics in slash and burn agriculture and land use alternatives of the humid forest zone in Cameroon. Agric Ecosyst Environ 1997;65:245-56.
50. Aumtong S, Magid J, Bruun S, de Neergaard A. Relating soil carbon fractions to land use in sloping uplands in northern Thailand. Agric Ecosyst Environ 2009;131:229-39.
51. Kilawe CJ, Maliondo SM, Bruun TB, Birch-Thomsen J, Silayo DSA, Mertz O. The intensification of shifting cultivation in Tanzania: effects on soil and vegetation. In: Rukhsana Alam A, editor. Agriculture, environment and sustainable development. 2022; pp. 21-42
52. Palm CA, van Noordwijk M, Woomer P, et al. Carbon losses and sequestration following land use change in the humid tropics. In: slash and burn agriculture - the search for alternatives. Columbia University Press; 2005; pp. 41-63. Available from: https://www.researchgate.net/publication/303211824_Carbon_losses_and_sequestration_following_land_use_change_in_the_humid_tropics [Last accessed on 1 June 2023].
53. Silva JMN, Carreiras JMB, Rosa I, Pereira JMC. Greenhouse gas emissions from shifting cultivation in the tropics, including uncertainty and sensitivity analysis. J Geophys Res 2011;116:20.
54. van Leeuwen TT, van der Werf GR, Hoffmann AA, et al. Biomass burning fuel consumption rates: a field measurement database. Biogeosciences 2014;11:7305-29.
55. Lauk C, Erb K. Biomass consumed in anthropogenic vegetation fires: global patterns and processes. Ecol Econ 2009;69:301-9.
56. van der Werf GR, Randerson JT, Giglio L, et al. Global fire emissions and the contribution of deforestation, savanna, forest, agricultural, and peat fires (1997-2009). Atmos Chem Phys 2010;10:11707-35.
57. Seiler W, Crutzen PJ. Estimates of gross and net fluxes of carbon between the biosphere and the atmosphere from biomass burning. Clim Chang 1980;2:207-47.
58. Kurien AJ, Lele S, Nagendra H. Farms or forests? Understanding and mapping shifting cultivation using the case study of West Garo hills, India. Land 2019;8:133.
59. van Vliet N, Mertz O, Heinimann A, et al. Trends, drivers and impacts of changes in swidden cultivation in tropical forest-agriculture frontiers: A global assessment. Glob Environ Chang 2012;22:418-29.
60. Coomes OT, Cheng Y, Takasaki Y, Abizaid C. What drives clearing of old-growth forest over secondary forests in tropical shifting cultivation systems? Evidence from the Peruvian Amazon. Ecol Econ 2021;189:107170.
61. Dove MR. Smallholder rubber and swidden agriculture in Borneo: A sustainable adaptation to the ecology and economy of the tropical forest. Econ Bot 1993;47:136-47.
62. Wadley RL, Mertz O. Pepper in a time of crisis: smallholder buffering strategies in Sarawak, Malaysia and West Kalimantan, Indonesia. Agric Syst 2005;85:289-305.
63. Cramb RA. Land and longhouse: agrarian transformation in the uplands of sarawak. Copenhagen: NIAS Press; 2011. Available from: https://urn.kb.se/resolve?urn=urn:nbn:se:norden:org:diva-4045 [Last accessed on 1 June 2023].
64. Mertz O, Egay K, Bruun TB, Colding TS. The last Swiddens of Sarawak, Malaysia. Hum Ecol 2013;41:109-18.
65. Frolking S, Palace MW, Clark DB, Chambers JQ, Shugart HH, Hurtt GC. Forest disturbance and recovery: a general review in the context of spaceborne remote sensing of impacts on aboveground biomass and canopy structure. J Geophys Res 2009;114:91.
66. Martin DA, Llopis JC, Raveloaritiana E, et al. Drivers and consequences of archetypical shifting cultivation transitions. People Nat 2023;5:529-41.
67. Curtis PG, Slay CM, Harris NL, Tyukavina A, Hansen MC. Classifying drivers of global forest loss. Science 2018;361:1108-11.
68. Harris NL, Gibbs DA, Baccini A, et al. Global maps of twenty-first century forest carbon fluxes. Nat Clim Chang 2021;11:234-40.
69. Su M, Shi Y, Yang Y, Guo W. Impacts of different biomass burning emission inventories: simulations of atmospheric CO2 concentrations based on GEOS-Chem. Sci Total Environ 2023;876:162825.
70. Castella J, Lu J, Friis C, et al. Beyond the boom-bust cycle: an interdisciplinary framework for analysing crop booms. Glob Environ Chang 2023;80:102651.
71. Vieira ICG, de Almeida AS, Davidson EA, Stone TS, de Carvalho CJR, Guerrero JB. Classifying successional forests using Landsat spectral properties and ecological characteristics in eastern Amazônia. Remote Sens Environ 2003;87:470-81.
72. Leite MFA, Luz RL, Muchavisoy KHM, et al. The effect of land use on aboveground biomass and soil quality indicators in spontaneous forests and agroforests of eastern Amazonia. Agrofor Syst 2016;90:1009-23.
73. Gehring C, Denich M, Vlek PLG. Resilience of secondary forest regrowth after slash and burn agriculture in central Amazonia. J Trop Ecol 2005;21:519-27.
74. Broadbent EN, Almeyda Zambrano AM, Asner GP, et al. Integrating stand and soil properties to understand foliar nutrient dynamics during forest succession following slash and burn agriculture in the Bolivian Amazon. PLoS One 2014;9:e86042.
75. Aryal DR, De Jong BH, Ochoa-gaona S, Esparza-olguin L, Mendoza-vega J. Carbon stocks and changes in tropical secondary forests of southern Mexico. Agric Ecosyst Environ 2014;195:220-30.
76. Lawrence D, Foster D. Changes in forest biomass, litter dynamics and soils following shifting cultivation in southern Mexico: an overview. 2002. Available from: https://www.redalyc.org/pdf/339/33907103.pdf [Last accessed on 1 June 2023].
77. Urquiza-haas T, Dolman PM, Peres CA. Regional scale variation in forest structure and biomass in the Yucatan Peninsula, Mexico: Effects of forest disturbance. For Ecol Manag 2007;247:80-90.
78. Eaton JM, Lawrence D. Loss of carbon sequestration potential after several decades of shifting cultivation in the Southern Yucatán. For Ecol Manag 2009;258:949-58.
79. Leprun J, Grouzis M, Randriambanona H. Post-cropping change and dynamics in soil and vegetation properties after forest clearing: example of the semi-arid Mikea region (southwestern Madagascar). Comptes Rendus Geoscience 2009;341:526-37.
80. McNicol IM, Ryan CM, Williams M. How resilient are African woodlands to disturbance from shifting cultivation? Ecol Appl 2015;25:2320-36.
81. Kenzo T, Ichie T, Hattori D, Kendawang JJ, Sakurai K, Ninomiya I. Changes in above- and belowground biomass in early successional tropical secondary forests after shifting cultivation in Sarawak, Malaysia. For Ecol Manag 2010;260:875-82.
82. Chan N, Takeda S, Suzuki R, Yamamoto S. Establishment of allometric models and estimation of biomass recovery of swidden cultivation fallows in mixed deciduous forests of the Bago Mountains, Myanmar. For Ecol Manag 2013;304:427-36.
83. Van Do T, Osawa A, Thang NT. Recovery process of a mountain forest after shifting cultivation in Northwestern Vietnam. For Ecol Manag 2010;259:1650-9.
84. Frizano J, Vann DR, Johnson AH, Johnson CM, Vieira ICG, Zarin DJ. Labile phosphorus in soils of forest fallows and primary forest in the Bragantina region, Brazil. Biotropica 2003;35:2-11.
85. Johnson CM, Vieira IC, Zarin DJ, Frizano J, Johnson AH. Carbon and nutrient storage in primary and secondary forests in eastern Amazônia. For Ecol Manag 2001;147:245-52.
86. Lemos ECM, Vasconcelos SS, Santiago WR, de Oliveira MCM, Souza CMDA. The responses of soil, litter and root carbon stocks to the conversion of forest regrowth to crop and tree production systems used by smallholder farmers in eastern Amazonia. Soil Use Manag 2016;32:504-14.
87. Nounamo L, Yemefack M, Tchienkoua M, Njomgang R. Impact of natural fallow duration on topsoils characteristics of a Ferralsol of southern Cameroon. 2002. Available from: https://www.researchgate.net/publication/233420608 [Last accessed on 1 June 2023].
88. Yemefack M, Rossiter DG, Jetten VG. Empirical modelling of soil dynamics along a chronosequence of shifting cultivation systems in southern Cameroon. Geoderma 2006;133:380-97.
89. Ralte V, Pandey H, Barik S, Tripathi R, Prabhu SD. Changes in microbial biomass and activity in relation to shifting cultivation and horticultural practices in subtropical evergreen forest ecosystem of north-east India. Acta Oecologica 2005;28:163-72.
90. Montfort F, Nourtier M, Grinand C, et al. Regeneration capacities of woody species biodiversity and soil properties in Miombo woodland after slash and burn agriculture in Mozambique. For Ecol Manag 2021;488:119039.
Cite This Article
Export citation file: BibTeX | RIS
OAE Style
Bruun TB, Hepp CM. Carbon footprint of shifting cultivation landscapes: current knowledge, assumptions and data gaps. Carbon Footprints 2023;2:11. http://dx.doi.org/10.20517/cf.2023.06
AMA Style
Bruun TB, Hepp CM. Carbon footprint of shifting cultivation landscapes: current knowledge, assumptions and data gaps. Carbon Footprints. 2023; 2(2): 11. http://dx.doi.org/10.20517/cf.2023.06
Chicago/Turabian Style
Bruun, Thilde Bech, Catherine Maria Hepp. 2023. "Carbon footprint of shifting cultivation landscapes: current knowledge, assumptions and data gaps" Carbon Footprints. 2, no.2: 11. http://dx.doi.org/10.20517/cf.2023.06
ACS Style
Bruun, TB.; Hepp CM. Carbon footprint of shifting cultivation landscapes: current knowledge, assumptions and data gaps. Carbon. Footprints. 2023, 2, 11. http://dx.doi.org/10.20517/cf.2023.06
About This Article
Copyright
Data & Comments
Data
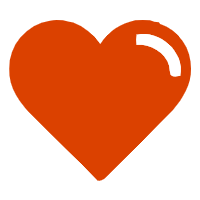

Comments
Comments must be written in English. Spam, offensive content, impersonation, and private information will not be permitted. If any comment is reported and identified as inappropriate content by OAE staff, the comment will be removed without notice. If you have any queries or need any help, please contact us at support@oaepublish.com.